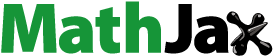
ABSTRACT
In various studies, researchers have utilised different solvents to extract sulfur compounds from the fuels. This paper introduces a novel magnetic deep eutectic solvent employed in the ultrasound-assisted oxidative desulfurisation of dibenzothiophene within a model fuel. The solvent, synthesised at 85°C by combining choline chloride, p-toluene sulfonic acid, and FeCl₃, underwent characterisation through Fourier transform-infrared, Vibrating-sample magnetometer, and Raman spectrometry. Exploration of the key parameters, including oxidant to model fuel volumetric fraction percentage (0.2–0.3), Iron (III) chloride to choline chloride mole fraction (0.1–0.3), and Magnetic deep eutectic solvent to model fuel volumetric fraction (0.2–0.3), was conducted to assess their impact on dibenzothiophene conversion rates. Employing response surface methodology based on central composite experimental design, optimal operational parameters for the ultrasound-assisted oxidative desulfurisation process were identified, resulting in a sulfur removal rate of 98.7%. The findings highlight the solvent sustained efficiency even at higher dibenzothiophene concentrations. Additionally, the solvent paramagnetic properties facilitated an expedited separation from the fuel, leading to the significantly reduced process times.
Nomenclature
MDES | = | Magnetic deep eutectic solvent |
DES | = | Deep eutectic solvent |
UAOD | = | Ultrasound-assisted oxidative desulfurisation |
ECODS | = | Extractive and catalytic oxidative desulfurisation |
ODS | = | oxidative desulfurisation |
HBA | = | Hydrogen bond acceptor |
HBD | = | Hydrogen bond donor |
DBT | = | Dibenzothiophene |
ChCl | = | Choline chloride |
p-TsOH | = | Para Toluenesulfonic acid |
RSM | = | Response surface methodology |
CCD | = | Central composite experimental design |
FT-IR | = | Fourier transform-infrared |
VSM | = | Vibrating-sample magnetometer |
1. Introduction
The presence of sulfur compounds in fossil fuels causes undesirable side-effects such as emissions of S that contribute to air and environmental pollution. To mitigate these effects, stringent regulations have been implemented to produce fossil fuels with significantly reduced sulfur content (Song Citation2003; Srivastava Citation2012). Currently, hydrodesulfurization (HDS) stands as a common desulfurisation process employed for the removal of sulfur components from conventional fuels (Gaudino et al. Citation2014; Khodaei, Sobati, and Shahhosseini Citation2016). However, HDS technology faces challenges in effectively eliminating certain aromatic sulfur compounds, like dibenzothiophene (DBT), due to their steric hindrances, even under harsh operating conditions involving high temperatures and pressures (Macaud et al. Citation2000; Sobati, Dehkordi, and Shahrokhi Citation2010; Song and Ma Citation2003). Therefore, other desulfurisation technologies, such as absorption, extraction, and oxidative desulfurisation, have gained more attention in recent years (Rezaee, Feyzi, and Dehghani Citation2021; Saedi et al. Citation2022; Shu and Sun Citation2016; Tang et al. Citation2015). In the realm of the alternative desulfurisation technologies, oxidative desulfurisation (ODS) has garnered significant interest due to its feasibility under mild operating conditions. After the oxidation of the sulfur compounds, sulfoxide and sulfone are produced. Next, these polar oxidised products can be eliminated from the fuel through techniques such as adsorption and solvent extraction (Ja’fari, Ebrahimi, and Khosravi-Nikou Citation2018; Yang et al. Citation2016). However, the use of traditional organic solvents in these processes raises concerns about their toxicity, volatility, and flammability, leading to environmental and human health issues. In response, efforts have been directed towards identifying green and environmentally benign solvents(Gano et al. Citation2015; Jiang et al. Citation2016). Due to the many desirable properties of ionic liquids (ILs), such as non-volatile, recyclable, high solubility, and low vapour pressure, they can be used in the Extractive Desulfurization (EDS) process or Extractive Catalytic Oxidative Desulfurization (ECODS) process as the new extractants (Ferreira et al. Citation2020; Hansmeier, Meindersma, and de Haan Citation2011; Jiang et al. Citation2016; Jiang et al. Citation2016; Mafi, Dehghani, and Mokhtarani Citation2018; Yan et al. Citation2019). However, due to some challenges such as high costs, purification difficulties, and hazardous toxicity, the use of IL technologies on large scales has been limited (Pätzold et al. Citation2019). In recent years, DESs, as a new class of green solvents, often referred to as ionic liquids analogs, have been paid more attention. DESs exhibit excellent physical and chemical properties, including thermal stability, nontoxicity, polarity, surface tension, low vapour pressure, low costs, biodegradability, and renewability (Cooper et al. Citation2004; Hao et al. Citation2017; Wang et al. Citation2016). DES is typically composed of two or more components, HBA and HBD. HBAs are usually quaternary ammonium salts such as ChCl, and HBD include organic acids, alcohols, and others that are combined with particular molar ratios(Smith, Abbott, and Ryder Citation2014; Tiecco et al. Citation2019; Yucui, Congfei, and Weize Citation2018). Beyond their applications in various fields like catalysis, electrochemistry, separation, and synthesis, DESs have found utility as green solvents in oxidative desulfurisation processes (Guo et al. Citation2013; Oliveira et al. Citation2013; Saaedi et al. Citation2022; Stepankova et al. Citation2014; Zaidi et al. Citation2014; Zhang et al. Citation2012; Zhang et al. Citation2014). Yin et al. (Yin et al. Citation2015) used the DES choline chloride/p-toluenesulfonic acid (ChCl/p-TsOH) and tetrabutylammonium chloride/p-toluenesulfonic acid (TBAC/p-TsOH) for the deep oxidation/extraction desulfurisation of model fuel (benzothiophene in n-octane) where efficiency of 99.99% was achieved. Wei Jiang et al. (Jiang et al. Citation2019) report on a class of ternary DESs formed by ChCl, polyethylene glycol (PEG), and boric acid (BA) in which the desulfurisation of DBT reached 99.2% in two hours at 60
and O/S molar ratio of Khezeli et al(Khezeli and Daneshfar Citation2017). Notably, the incorporation of iron chloride (FeCl3) as a Magnetic Agent in Magnetic Deep Eutectic Solvent (MDES) has been introduced for thiophene extraction from heptane (Khezeli and Daneshfar Citation2017). The application of MDES in Extractive Catalytic Oxidative Desulfurization (ECODS) has been explored for various sulfur compounds (DBT, 4,6-DMDBT, BT). In their work, Metal-based deep eutectic solvents synthesised with ChCl as HBA and various HBDs, coupled with cobalt chloride (CoCl2) as a magnetic agent, demonstrated high desulfurisation efficiency (PMS). (Xu et al. Citation2018).
Moreover, MDESs, With their favourable conductivity and appropriate polarity, find applications beyond desulfurisation. In biotechnology, MDESs serve as solvents for Solvent-Based Liquid–Liquid Extraction, enabling selective extraction and separation of Ribonucleic acid (RNA) (Peng et al. Citation2022; Shi et al. Citation2022). In the food industry, MDESs have been employed for microextraction purposes, such as the extraction and pre-concentration of melamine from milk and milk-based products (Elik et al. Citation2023). Similarly, Babaee et al. utilised MDESs for liquid–liquid microextraction in the determination of hexanal and heptanal in edible oils, followed by gas chromatography (Babaee and Daneshfar Citation2018).
Ultrasound irradiation stands out as a potent and efficient tool for enhancing mixing between the oil phase and the aqueous phase. In the Ultrasound-Assisted Oxidative Desulfurization (UAOD) process, the application of ultrasound generates micro-bubbles, significantly intensifying mixing and influencing the mass transfer rate. Additionally, ultrasound irradiation exerts an impact on the kinetic reaction, leading to an accelerated oxidation reaction and notably reducing the desulfurisation time frame. (Karami et al. Citation2017; Khodaei, Sobati, and Shahhosseini Citation2017; Li et al. Citation2016; Mofidi and Shahhosseini Citation2022).
In the existing literature, the impact of incorporating FeCl₃ into the deep eutectic solvent (choline chloride/p-toluene sulfonic) has not been explored(Makoś-Chełstowska et al. Citation2022). Consequently, this study investigates the Extractive Catalytic Oxidative Desulfurization (ECODS) process utilising the innovative Magnetic Deep Eutectic Solvent (MDES). Furthermore, ultrasound irradiation was employed to maximise mixing efficiency. Leveraging the magnetic properties of MDES, an inventive investigation into the influence of a magnetic field on the separation of organic and inorganic phases after the oxidation stage was conducted. The study also systematically assessed the effects of operational variables on sulfur oxidation using the Response Surface Methodology (RSM) approach, specifically the central composite design, to optimise ECODS efficiency with MDES.
2. Materials and methods
2.1. Materials
All materials were purchased according to and used without further purification.
Table 1. Materials Inventory.
2.2. Synthesisation of the magnetic deep eutectic solvent and model fuel
The MDES was synthesised by the heating method. ChCl and p-TsOH were selected as HBA and HBD, respectively. The synthesis involved mixing the specified quantities of ChCl, p-TsOH, and FeCl3 in a molar ratio of 1:2:x (HBA: HBD: FeCl3), where x was varied. In this ratio, the viscosity of the solvent is at its lowest point(Rodriguez Rodriguez, Machiels, and Binnemans Citation2019). The mixture was then heated to 85 ℃ in an oil bath for two hours, undergoing intense mixing with a heater stirrer until a homogenous red solution was achieved. Subsequently, a model fuel was prepared with an initial sulfur concentration of 500 ppm by dissolving 1.4921 grams of DBT in 500 ml of n-Decane. The calculation for the amount of DBT in the model fuel is as follows:
(1)
(1) The structures of each element of DES are shown in .
Table 2. Structures of each component of the Magnetic deep eutectic solvent.
2.3. UAOD process of the model fuel
In each ECODS test, 25 ml of model fuel (500 ppm), MDES, and were added into a 33-mL jacket-type reactor. In order to apply ultrasound irradiation in the reactor, a 400 W ultrasound device featuring a steady frequency of 20 kHz and titanium probe tip (12 mm diameter) was used. The ultrasound probe was inserted 1 cm into the reactant approximately and the device power was set at 360 W. Simultaneously with the addition of H₂O₂ to the mixture of the model fuel and MDES, the reaction time commenced. The three-necked flask was equipped with an electronic thermometer and condenser. The reaction was carried out at a constant temperature of 50
. After 3 min, the reaction was stopped and the oil and aqueous phases were separated from each other by a separatory funnel. In order to ascertain the amount of final concentration of DBT in the model fuel post-reaction, a specified quantity of the model fuel was separated and analyzed by GC analysis (GC Agilent 7890 FID). The DBT conversion can be determined as follows:
(2)
(2) In which
is equal to DBT concentration in the model fuel before the UAOD process and,
is DBT concentration at the end.
2.4. Design of the experiments
In this study, three operating variables as presented in (FeCCholine Chloride mole fraction,
percentage and, VDES/VFuel ratio) were optimised using the RSM method to achieve the maximum sulfur conversion rate. represents the ranges of the effective parameters and factor levels of the UAOD process. To ensure accuracy of the results, each test was repeated a minimum of two times, while some tests were randomly repeated three times. The results revealed a maximum discrepancy of 0.3% compared to the original outcome, ensuring certainty of the findings.
Table 3. Factor level of the process variables.
3. Results and discussion
3.1. Characterisation of MDES
In order to understand the magnetic and structural properties of the synthesised MDES. Fourier transform-infrared (FTIR), Vibrating-sample magnetometer (VSM), HNMR, CNMR, TGA and Raman spectroscopy analyses were performed.
3.1.1. FTIR spectroscopy
FTIR analysis was employed to confirm the existence of hydrogen bonds among the components of the MDES. The analysis was conducted using the PerkinElmer Spectrum100 instrument. depicts FTIR spectra for MDES, PTS Acid, and ChCl. As illustrated in , the C–H vibration shifted from 3430 in ChCl to 3390
in MDES. Consistent with previous studies, these shifts to lower frequencies indicate the presence of hydrogen bonds in that region(Gautam, Kumar, and Lynam Citation2020; Zhu et al. Citation2016).
In p-TsOH spectrum, the presence of lower peaks at 1189 and 1179 cm⁻¹ indicates the presence of a strong asymmetric bond between sulfur and oxygen, while a symmetric bond is observed at 1040 cm⁻¹. Additionally, the lower peaks at 1128 and 117 cm⁻¹ suggest the presence of a bond between carbon and hydrogen, with a peak at 1009 cm⁻¹ indicating the presence of a C–C bond. Moreover, a peak at 640 cm⁻¹ corresponds to the bond between carbon and sulfur. The obtained spectra demonstrate a significant correlation with the expected wavelengths, validating the presence of these specific bonds (Kang et al. Citation2017; Pejov, Ristova, and Šoptrajanov Citation2011). Regarding ChCl, several significant peaks can be observed: at 3020, 2963 cm⁻¹, 1721cm⁻¹, 1232, and 1086 cm⁻¹. These peaks correspond to OH stretching, C–H stretching, C–O stretching, sp² C–O stretching, and sp³ C–O stretching, respectively. These spectral features provide evidence for the presence of these specific chemical bonds within the ChCl molecular structure(Gautam, Kumar, and Lynam Citation2020).
3.1.2. VSM analysis
Due to the use of FeC in the eutectic solvent, the magnetic property of the MDES is determined using VSM analysis. The VSM analysis was performed using the Magnetic Daneshpajoh Kashan MDKB model. As shown in , the magnetic susceptibility is directly proportional to the applied magnetic field, as indicated by the straight line. This confirms that the deep eutectic solvent exhibits paramagnetic behaviour. The slope of the fitted line is 0.05 emu/g for MDES. The paramagnetic properties of the eutectic solvent facilitate straightforward separation through the application of an external magnetic field.
3.1.3. Raman spectra
One of the techniques of FeCl4− detection is Raman spectroscopy. The Raman spectroscopy was performed by Teksan TakRam N1-541 model. Some sources attribute the strong binding at 330 cm−1 to FeCl4− (Bäcker et al. Citation2011; García-Saiz et al. Citation2015). According to , the presence of a peak in the diagram at 336 cm−1 indicates the symmetric Fe-Cl stretch in MDES. This bond is a proof of the FeCl4−1 existence. The direction of the magnetic field for separation is determined according to the presence of the anions.
3.1.4. H-NMR and C-NMR
presents NMR spectra for the MDES, with a depicting the HNMR (proton nuclear magnetic resonance) spectrum and b showing the CNMR (carbon nuclear magnetic resonance) spectrum. This analysis was conducted using a Bruker DRX-500 spectrometer, with Dimethyl sulfoxide (DMSO) employed as the solvent.
According to , the spectra of H-NMR reveal distinct peaks at 3.22, 3.42, and 3.85 ppm, which correspond to the hydrogen nuclei of Choline chloride. Additionally, the peaks at 2.29, 5.67, 7.15, and 7.48 ppm are observed, corresponding to the hydrogen nuclei of p-Toluenesulfonic acid. In the C-NMR spectrum, we observe distinct peaks at 52.96, 55.67, and 66.74 ppm, which are indicative of the carbon nuclei in Choline Chloride. Furthermore, there are discernible peaks at 20.90, 125.13, 128.6, 139.28, and 143.49 ppm, corresponding to the carbon nuclei in p-Toluenesulfonic acid.
It is worth noting that also specifies the peaks associated with DMSO (Dimethyl sulfoxide), which serves as the solvent in this analysis.
3.1.5. TGA
Thermogravimetric analysis (TGA) was conducted to investigate the thermal stability of the material, and the results are depicted in a. The analysis was carried out using a METTLER TOLEDO TGA/DSC/1 instrument, with a temperature range spanning from 25°C to 450°C and a heating rate of 20°C per minute. The decomposition of the MDES material exhibited two steps. The initial decomposition began at 25°C and concluded at 223°C, resulting in a weight loss of approximately 16%. However, the primary decomposition occurred within the subsequent temperature range, from 223°C to 450°C, resulting in a significant weight loss of nearly 69%. Additionally, the derivative thermogravimetry (DTG) data was presented in b, which serves as the derivative of the TGA analysis. As expected, milestones align with valleys. As evident from the graph, it exhibits two distinct lower peaks. The first peak is relatively small, indicating a minor change in weight, while the second peak is more substantial, indicating significant changes in the weight. Considering the temperature range required for desulfurisation operations, it is evident that the MDES material exhibits excellent heat stability.
3.1.6. Density and viscosity
The viscosity and density of the solvent were measured using the BROOKFIELD L (LV-DVE 23) at temperatures of 25°C, 35°C, 45°C, and 55°C. Density measurements were taken at the same temperatures. Additionally, viscosity was determined at different Round Per Minute (RPM) rates. The trends of viscosity and density can be observed in and .
3.2. Model fitting
In the current study, 20 experiments were conducted to eliminate the sulfur component (DBT) from the model fuel, and the outcomes are presented in . Among various parameters influencing DBT removal, three specific parameters are addressed in this report. The Response Surface Methodology (RSM) employed a second-order polynomial to analyze and determine the optimum response, as per EquationEquation (3)(3)
(3) .
(3)
(3) Where A, B, C, and, D represent FeC
/Choline chloride mole fraction
percentage, VDES/VFuel ratio, and the sulfur conversion (%), respectively. The influence of the three parameters on the response can be calculated using EquationEquation 3
(3)
(3) . Notably, among the independent factors, the term B, with a coefficient of 6.87, exhibits the most significant effect on the yield. Furthermore, among the two-parameter interactions, A and B, with a coefficient of 1.62, have the most substantial impact.(Asghar, Abdul Raman, and Daud Citation2014; Mahmoudi et al. Citation2021).
Table 4. Experimental results of desulfurisation of model fuel by the MDES.
shows the results of the Analysis of Variance (ANOVA), reporting both the F-value and P-value. According to this model, the P value model is less than 10−4. A low P-value shows that the second-order polynomial EquationEq. (3)(3)
(3) is substantial. In fact, if p-values is less than 0.5, it indicates the remarkableness of the factor (Khodaei, Sobati, and Shahhosseini Citation2017). In this context, the F-value, denoted as 340.84, is substantial, affirming the statistical significance of the model and its terms.
Table 5. Analysis of variance (ANOVA) for DBT conversion. Response 1: Sulfur removal.
According to P and F values, the terms A, B, C, AB, AC, ,
and,
are the significant model variables of the proposed correlation and, between them, B, C and,
are the most striking terms. In addition, among single terms, B with the F value of 1314.96 has the main impact on the sulfur conversion.
The coefficient of determination (R²) of 0.9968 indicates a strong agreement between the predicted polynomial equation and the experimental data. This implies that the model accounts for 99.68% of the total variation in the response. The normal probability plot of residuals depicted in a reveals that the residuals follow a normal distribution, and the actual data align well with the predicted values, affirming a robust fit between them. b, illustrating the residual values versus the predicted values, further supports the statistical reliability of the correlation, with all data falling within the range of −4.14579 and +4.14579. Consequently, these results validate the proposed correlation. Additionally, c showcases a strong agreement between the predicted values and the experimental data. (Asghar, Abdul Raman, and Daud Citation2014; Jensen Citation2017; Mahmoudi et al. Citation2021).
3.3. Effect of different parameters on UAOD
The response surface plots, depicted in , illustrate the interactions between various independent process parameters (A, B, and C) in the UAOD process in the presence of Magnetic Deep Eutectic Solvent (MDES) and the rate of sulfur removal.
3.3.1. Effect of A: FeC
/Choline Chloride mole fraction and B: 
percentage.
a shows the relationship between A and B with sulfur removal. It can be seen that increasing in the A parameter at high values of the B parameter leads to an augmentation in the sulfur conversion rate. According to the contour (a), The B parameter exhibits a more pronounced impact on sulfur conversion compared to the A parameter. In essence, variations in the B parameter have a more substantial influence on sulfur conversion. The highest sulfur conversion is achieved when the B parameter falls within the range of 1-1.5%, and the A parameter is in the range of 0.2-0.3. Furthermore, an increase in the B parameter up to 1.35% leads to enhanced sulfur removal, but further increases result in a slight decrease in sulfur conversion. This phenomenon can be attributed to the potential formation of water at higher B parameter values, leading to solvent dilution. Consequently, the acidity of the solvent diminishes, potentially reducing the sulfur conversion rate. Thus, there appears to be an optimal value for the amount of hydrogen peroxide (H₂O₂) used in the process. This observation aligns well with the findings of Mofidi and Shahhosseini Citation2022.
3.3.2. Effect of A: FeC
/Choline Chloride mole fraction and C: VDES/VFuel
According to b, the effect of increasing the C parameter on DBT conversion is relatively minor. As shown in the b, the maximum sulfur removal is achieved when A is within the range of 0.2-0.3, and the highest sulfur removal occurs when C ≈ 0.23. Additionally, b indicates that elevating the A parameter up to 0.27 leads to an increase in the desulfurisation rate. However, exceeding this threshold may result in a decrease in the sulfur conversion rate. Consequently, it can be inferred that the presence of FeCl₃ positively influences the performance of the deep eutectic solvent and enhances the sulfur conversion rate.
3.3.3. Effect of B: 
percentage and C: VDES/VFuel
c shows the interaction between the B and C parameters. As shown in c, increasing the B parameter up to the optimum value enhances the efficiency of the ECODS process. The sulfur removal rate exhibits nearly linear growth with increasing B, underscoring the high quality of the MDES. Specifically, at B = 1.35% and C = 0.23, the maximum sulfur conversion of 98.7% can be attained. As elucidated in Sections 3.3.1 and 3.3.2, exceeding the optimal values for both parameters B and C may result in a reduction in sulfur conversion.
Indeed, the various diagrams obtained through the Response Surface Methodology (RSM) highlight that, the FeCl3/Choline Chloride mole fraction, percentage, and the VDES/VFuel ratio are three main and effective parameters for sulfur removal that should be optimised.
3.4. Process optimisation
One of the primary objectives of this study was to optimise the independent operating variables to attain maximum sulfur removal through the ECODS process with MDES. This optimisation utilised Response Surface Methodology (RSM) with Central Composite Design (CCD). According to the RSM results, the optimal sulfur removal of 98.7% was achieved with A = 0.27, B = 1.35%, and C = 0.23 at a constant temperature of 50 ℃.
To validate the proposed model, the experiments were replicated three times under the optimal operating conditions. illustrates that the experimental sulfur conversion closely aligns with the predicted values, affirming the validity of the proposed model using the RSM method.
Table 6. Validation tests under optimum operating conditions.
As indicated by Li et al. (Citation2016) in their research, the presence of metal ions in DESs can enhance the desulfurisation rate. This study demonstrates an increase in the amount of desulfurisation by approximately 1% compared to (Mofidi and Shahhosseini Citation2022) and (Yin et al. Citation2015)under the same operational conditions.
3.5. MDES performance in different initial concentrations of DBT
One of the critical parameters to consider in desulfurisation processes is the initial sulfur content of the model fuel. In this study, a DBT conversion of 98.71% was achieved after 3 min when the initial sulfur content was 500 ppm (model fuel, DBT) using the Ultrasound-Assisted Oxidative Desulfurization (UAOD) process. However, when the initial sulfur content was increased to 1000 ppm (model fuel, DBT), the sulfur removal rate decreased to 92.18%, as depicted in . This observation indicates that the desulfurisation efficiency diminishes with an increase in the initial sulfur content under constant operating conditions. Nonetheless, the synthesised solvent still exhibits acceptable performance across different DBT concentrations.
3.6. Effect of the solvent magnetic property on desulfurisation rate
To investigate the impact of magnetic properties on the desulfurisation rate, a funnel separator was positioned inside a solenoid generating a magnetic field of 300 Gauss for 5 min. As illustrated in , following the Right-hand rule, the direction of the field lines should be from the bottom to the top due to the presence of an anion in the solvent. The obtained results were compared to those of an equivalent sample under the same conditions without applying a magnetic field.
demonstrates that the presence of a magnetic field at a specific time significantly influences the desulfurisation rate. It can be inferred that the utilisation of a magnetic field expedites the overall process time to achieve a consistent desulfurisation rate. Given sufficient time, both samples would eventually reach the same final desulfurisation percentage. Thus, the presence of the magnetic field primarily expedites the separation stage.
3.7. Suggested mechanism for DBT removal
Understanding the mechanism of catalytic oxidative desulfurisation with deep eutectic solvents (DESs) is essential for optimising their physicochemical properties. Upon adding the oxidant, p-TsOH undergoes conversion to peroxysulfonic acid, subsequently oxidising dibenzothiophene (DBT) to its corresponding sulfones. (Li et al. Citation2018). Due to the high polarity of DBTO2, it tends to dissolve and persist in the polar deep eutectic solvents (DESs). In catalytic oxidative desulfurisation with DESs, sulfur compounds in the organic phase undergo oxidation and extraction into the deep eutectic solvent, driven by their increasing polarity. It is essential to emphasise that DESs simultaneously serve as a solvent, catalyst, and extractant in the desulfurisation process.
Ultrasound irradiation proves to be a valuable method for enhancing the performance and rate of the oxidative desulfurisation process. Ultrasound-assisted oxidative desulfurisation (UAOD) can augment the reaction through acoustic cavitation, intensifying micro-mixing in the biphasic oxidation system and boosting the mass transfer rates between the two phases.(Mofidi and Shahhosseini Citation2022).
4. Conclusion
The ECODS process, employing Magnetic Deep Eutectic Solvent (MDES) and ultrasound irradiation, has proven effective in reducing the sulfur content of a model fuel. The MDES formulation, consisting of ChCl/p-TsOH/FeC, serves as a robust medium for separating sulfur compounds from the model fuel. The Response Surface Methodology (RSM) approach, specifically the Central Composite Design (CCD), was adopted to explore the impacts of key parameters, namely A: FeC
Choline Chloride mole fraction, the B:
percentage, and the C: VDES/VFuel. and to identify optimal values for these operating parameters. The RSM method led to the identification of optimum conditions, resulting in the highest sulfur conversion of 98.71%. In the context of this study, the findings suggest that variations in the initial sulfur content of the fuel do not significantly diminish the efficiency of the MDES. Furthermore, the introduction of FeCl3 into the eutectic solvent imparts paramagnetic properties, facilitating a rapid separation from the model fuel when subjected to an external magnetic field.
Expanding upon these insights, a noteworthy research gap emerges in the evaluation of the burning efficiency of desulfurised fuel. Future studies could delve into assessing the combustion characteristics and overall performance of the fuel treated through the ECODS process. This avenue of investigation holds promise for comprehensively understanding the practical implications and environmental benefits of desulfurised fuels. Moreover, the optimisation of additional parameters presents an exciting prospect for future research endeavours. Parameters such as the power of ultrasound, reaction time, reaction temperature, the magnitude of the magnetic field, and variations in the formulation of the solvent offer rich avenues for exploration. Investigating these aspects could lead to enhanced process efficiency, sustainability, and broader applicability of the ECODS method. An intriguing suggestion for future studies involves the incorporation of magnetic nanoparticles into MDES synthesis. This innovative approach has the potential to yield more favourable outcomes, leveraging the unique properties of magnetic nanoparticles for improved separation and desulfurisation efficiency.
In essence, while this study successfully demonstrates the efficacy of ECODS with MDES in sulfur removal, there exist exciting avenues for future research to optimise and extend the applicability of this desulfurisation method.
Disclosure statement
No potential conflict of interest was reported by the author(s).
Correction Statement
This article was originally published with errors, which have now been corrected in the online version. Please see Correction https://doi.org/10.1080/14786451.2024.2365005.
Additional information
Funding
References
- Asghar, A., A. A. Abdul Raman, and W. M. A. W. Daud. 2014. “A Comparison of Central Composite Design and Taguchi Method for Optimizing Fenton Process.” The Scientific World Journal 2014: 14. Article ID: 869120. https://doi.org/10.1155/2014/869120.
- Babaee, S., and A. Daneshfar. 2018. “Magnetic Deep Eutectic Solvent-Based Ultrasound-Assisted Liquid–Liquid Microextraction for Determination of Hexanal and Heptanal in Edible Oils Followed by gas Chromatography–Flame Ionization Detection.” Analytical Methods 10 (34): 4162–4169. https://doi.org/10.1039/C8AY01058G.
- Bäcker, T., O. Breunig, M. Valldor, K. Merz, V. Vasylyeva, and A.-V. Mudring. 2011. “In-Situ Crystal Growth and Properties of the Magnetic Ionic Liquid [C2mim][FeCl4].” Crystal Growth & Design 11 (6): 2564–2571. https://doi.org/10.1021/cg200326n.
- Cooper, E. R., C. D. Andrews, P. S. Wheatley, P. B. Webb, P. Wormald, and R. E. Morris. 2004. “Ionic Liquids and Eutectic Mixtures as Solvent and Template in Synthesis of Zeolite Analogues.” Nature 430 (7003): 1012–1016. https://doi.org/10.1038/nature02860.
- Elik, A., S. Fesliyan, N. Gürsoy, H. U. Haq, R. Castro-Muñoz, and N. Altunay. 2023. “An air-Assisted Dispersive Liquid Phase Microextraction Method Based on a Hydrophobic Magnetic Deep Eutectic Solvent for the Extraction and Preconcentration of Melamine from Milk and Milk-Based Products.” Food Chemistry 426: 136573.
- Ferreira, E. S., I. V. Voroshylova, N. M. Figueiredo, C. M. Pereira, and M. N. D. Cordeiro. 2020. “Computational and Experimental Study of Propeline: A Choline Chloride Based Deep Eutectic Solvent.” Journal of Molecular Liquids 298: 111978. https://doi.org/10.1016/j.molliq.2019.111978.
- Gano, Z. S., F. S. Mjalli, T. Al-Wahaibi, Y. Al-Wahaibi, and I. M. AlNashef. 2015. “Extractive Desulfurization of Liquid Fuel with FeCl3-Based Deep Eutectic Solvents: Experimental Design and Optimization by Central-Composite Design.” Chemical Engineering and Processing: Process Intensification 93: 10–20. https://doi.org/10.1016/j.cep.2015.04.001.
- García-Saiz, A., I. de Pedro, O. Vallcorba, P. Migowski, I. Hernandez, L. F. Barquin, I. Abrahams, M. Motevalli, J. Dupont, and J. A. Gonzalez. 2015. “1-Ethyl-2,3-dimethylimidazolium Paramagnetic Ionic Liquids with 3D Magnetic Ordering in its Solid State: Synthesis, Structure and Magneto-Structural Correlations.” Rsc Advances 5 (75): 60835–60848. https://doi.org/10.1039/C5RA05723J.
- Gaudino, E. C., D. Carnaroglio, L. Boffa, G. Cravotto, E. M. Moreira, M. A. Nunes, V. L. Dressler, and E. M. Flores. 2014. “Efficient H2O2/CH3COOH Oxidative Desulfurization/Denitrification of Liquid Fuels in Sonochemical Flow-Reactors.” Ultrasonics Sonochemistry 21 (1): 283–288. https://doi.org/10.1016/j.ultsonch.2013.04.009.
- Gautam, R., N. Kumar, and J. G. Lynam. 2020. “Theoretical and Experimental Study of Choline Chloride-Carboxylic Acid Deep Eutectic Solvents and Their Hydrogen Bonds.” Journal of Molecular Structure 1222: 128849. https://doi.org/10.1016/j.molstruc.2020.128849.
- Guo, W., Y. Hou, W. Wu, S. Ren, S. Tian, and K. N. Marsh. 2013. “Separation of Phenol from Model Oils with Quaternary Ammonium Saltsvia Forming Deep Eutectic Solvents.” Green Chemistry 15 (1): 226–229. https://doi.org/10.1039/C2GC36602A.
- Hansmeier, A. R., G. W. Meindersma, and A. B. de Haan. 2011. “Desulfurization and Denitrogenation of Gasoline and Diesel Fuels by Means of Ionic Liquids.” Green Chemistry 13 (7): 1907–1913. https://doi.org/10.1039/c1gc15196g.
- Hao, L., M. Wang, W. Shan, C. Deng, W. Ren, Z. Shi, and H. Lü. 2017. “L-proline-based Deep Eutectic Solvents (DESs) for Deep Catalytic Oxidative Desulfurization (ODS) of Diesel.” Journal of Hazardous Materials 339: 216–222. https://doi.org/10.1016/j.jhazmat.2017.06.050.
- Ja’fari, M., S. L. Ebrahimi, and M. R. Khosravi-Nikou. 2018. “Ultrasound-assisted Oxidative Desulfurization and Denitrogenation of Liquid Hydrocarbon Fuels: A Critical Review.” Ultrasonics Sonochemistry 40: 955–968. https://doi.org/10.1016/j.ultsonch.2017.09.002.
- Jensen, W. A. 2017. “Response Surface Methodology: Process and Product Optimization Using Designed Experiments 4th Edition.” Journal of Quality Technology 49 (2): 186. https://doi.org/10.1080/00224065.2017.11917988.
- Jiang, W., H. Jia, H. Li, L. Zhu, R. Tao, W. Zhu, H. Li, and S. Dai. 2019. “Boric Acid-Based Ternary Deep Eutectic Solvent for Extraction and Oxidative Desulfurization of Diesel Fuel.” Green Chemistry 21 (11): 3074–3080. https://doi.org/10.1039/C9GC01004A.
- Jiang, W., H. Li, C. Wang, W. Liu, T. Guo, H. Liu, W. Zhu, and H. Li. 2016. “Synthesis of Ionic-Liquid-Based Deep Eutectic Solvents for Extractive Desulfurization of Fuel.” Energy & Fuels 30 (10): 8164–8170. https://doi.org/10.1021/acs.energyfuels.6b01976.
- Jiang, B., H. Yang, L. Zhang, R. Zhang, Y. Sun, and Y. Huang. 2016. “Efficient Oxidative Desulfurization of Diesel Fuel Using Amide-Based Ionic Liquids.” Chemical Engineering Journal 283: 89–96. https://doi.org/10.1016/j.cej.2015.07.070.
- Kang, S., G. Zhang, X. Yang, H. Yin, X. Fu, J. Liao, J. Tu, X. Huang, F. G. Qin, and Y. Xu. 2017. “Effects of p-Toluenesulfonic Acid in the Conversion of Glucose for Levulinic Acid and Sulfonated Carbon Production.” Energy & Fuels 31 (3): 2847–2854. https://doi.org/10.1021/acs.energyfuels.6b02675.
- Karami, E., M. A. Sobati, B. Khodaei, and K. Abdi. 2017. “An Experimental Investigation on the Ultrasound-Assisted Oxidation of Benzothiophene in Model Fuel: Application of Response Surface Methodology.” Applied Thermal Engineering 118: 691–702. https://doi.org/10.1016/j.applthermaleng.2017.03.028.
- Khezeli, T., and A. Daneshfar. 2017. “Synthesis and Application of Magnetic Deep Eutectic Solvents: Novel Solvents for Ultrasound Assisted Liquid-Liquid Microextraction of Thiophene.” Ultrasonics Sonochemistry 38: 590–597. https://doi.org/10.1016/j.ultsonch.2016.08.023.
- Khodaei, B., M. A. Sobati, and S. Shahhosseini. 2016. “Optimization of Ultrasound-Assisted Oxidative Desulfurization of High Sulfur Kerosene Using Response Surface Methodology (RSM).” Clean Technologies and Environmental Policy 18 (8): 2677–2689. https://doi.org/10.1007/s10098-016-1186-z.
- Khodaei, B., M. A. Sobati, and S. Shahhosseini. 2017. “Rapid Oxidation of Dibenzothiophene in Model Fuel Under Ultrasound Irradiation.” Monatshefte für Chemie - Chemical Monthly 148 (2): 387–396. https://doi.org/10.1007/s00706-016-1801-z.
- Li, Z., D. Liu, Z. Men, L. Song, Y. Lv, P. Wu, B. Lou, Y. Zhang, N. Shi, and Q. Chen. 2018. “Insight Into Effective Denitrification and Desulfurization of Liquid Fuel with Deep Eutectic Solvents: An Innovative Evaluation Criterion to Filtrate Extractants Using the Compatibility Index.” Green Chemistry 20 (13): 3112–3120. https://doi.org/10.1039/C8GC00828K.
- Li, C., J. Zhang, Z. Li, J. Yin, Y. Cui, Y. Liu, and G. Yang. 2016. “Extraction Desulfurization of Fuels with ‘Metal Ions’ Based Deep Eutectic Solvents (MDESs).” Green Chemistry 18 (13): 3789–3795. https://doi.org/10.1039/C6GC00366D.
- Macaud, M., A. Milenkovic, E. Schulz, M. Lemaire, and M. Vrinat. 2000. “Hydrodesulfurization of Alkyldibenzothiophenes: Evidence of Highly Unreactive Aromatic Sulfur Compounds.” Journal of Catalysis 193 (2): 255–263. https://doi.org/10.1006/jcat.2000.2897.
- Mafi, M., M. R. Dehghani, and B. Mokhtarani. 2018. “Liquid-liquid Equilibrium Data for Extractive Desulfurization Using 1-Butyl-3-Methyl Imidazolium Thiocyanate, n- Alkane and Thiophene.” Fluid Phase Equilibria 456: 109–115. https://doi.org/10.1016/j.fluid.2017.10.017.
- Mahmoudi, V., A. M. Kermani, M. Ghahramaninezhad, and A. Ahmadpour. 2021. “Oxidative Desulfurization of Dibenzothiophene by Magnetically Recoverable Polyoxometalate-Based Nanocatalyst: Optimization by Response Surface Methodology.” Molecular Catalysis 509: 111611. https://doi.org/10.1016/j.mcat.2021.111611.
- Makoś-Chełstowska, P., M. Kaykhaii, J. Płotka-Wasylka, and M. de la Guardia. 2022. “Magnetic Deep Eutectic Solvents – Fundamentals and Applications.” Journal of Molecular Liquids 365: 120158. https://doi.org/10.1016/j.molliq.2022.120158.
- Mofidi, M., and S. Shahhosseini. 2022. “Ultrasound Assisted Oxidative Desulfurization of a Model Fuel Using a Deep Eutectic Solvent: Optimization and Experimental Design.” Chemical Engineering and Processing - Process Intensification 171: 108724. https://doi.org/10.1016/j.cep.2021.108724.
- Oliveira, F. S., A. B. Pereiro, L. P. Rebelo, and I. M. Marrucho. 2013. “Deep Eutectic Solvents as Extraction Media for Azeotropic Mixtures.” Green Chemistry 15 (5): 1326–1330. https://doi.org/10.1039/c3gc37030e.
- Pätzold, M., S. Siebenhaller, S. Kara, A. Liese, C. Syldatk, and D. Holtmann. 2019. “Deep Eutectic Solvents as Efficient Solvents in Biocatalysis.” Trends in Biotechnology 37 (9): 943–959. https://doi.org/10.1016/j.tibtech.2019.03.007.
- Pejov, L., M. Ristova, and B. Šoptrajanov. 2011. “Quantum Chemical Study of p-Toluenesulfonic Acid, p-Toluenesulfonate Anion and the Water–p-Toluenesulfonic Acid Complex. Comparison with Experimental Spectroscopic Data.” Spectrochimica Acta Part A: Molecular and Biomolecular Spectroscopy 79 (1): 27–34. https://doi.org/10.1016/j.saa.2011.01.007.
- Peng, F., X. Wang, W. Tao, Y. Chen, Y. Ma, and X. Ding. 2022. “Development of Magnetic Deep Eutectic Solvent-Based Liquid–Liquid Extraction for the Selective Extraction and Separation of RNA.” Langmuir 38 (36): 10934–10942. https://doi.org/10.1021/acs.langmuir.2c00882.
- Rezaee, M., F. Feyzi, and M. R. Dehghani. 2021. “Extractive Desulfurization of Dibenzothiophene from Normal Octane Using Deep Eutectic Solvents as Extracting Agent.” Journal of Molecular Liquids 333: 115991. https://doi.org/10.1016/j.molliq.2021.115991.
- Rodriguez Rodriguez, N., L. Machiels, and K. Binnemans. 2019. “p-Toluenesulfonic Acid-Based Deep-Eutectic Solvents for Solubilizing Metal Oxides.” ACS Sustainable Chemistry & Engineering 7 (4): 3940–3948. https://doi.org/10.1021/acssuschemeng.8b05072.
- Saaedi, M., M. Mehrpooya, A. Shabani, and S. Moosavian. 2022. “Design and Economic Analysis of Heat Exchangers Used in Solar Cogeneration Systems Based on Nanoworking Fluid.” Chemical Papers 76 (12): 7475–7492. https://doi.org/10.1007/s11696-022-02427-2.
- Saedi, M., M. Mehrpooya, A. Shabani, A. Zaitsev, and A. Nikitin. 2022. “Proposal and Investigation of a Novel Process Configuration for Production of Neon from Cryogenic air Separation Unit.” Sustainable Energy Technologies and Assessments 50: 101875. https://doi.org/10.1016/j.seta.2021.101875.
- Shi, R., F. Zhou, Y. Chen, Z. Liu, S. Liu, and T. Mu. 2022. “Magnetic Deep Eutectic Solvents: Formation and Properties.” Physical Chemistry Chemical Physics 24 (34): 20073–20081. https://doi.org/10.1039/D2CP01592G.
- Shu, C., and T. Sun. 2016. “Extractive Desulfurisation of Gasoline with Tetrabutyl Ammonium Chloride-Based Deep Eutectic Solvents.” Separation Science and Technology 51 (8): 1336–1343. https://doi.org/10.1080/01496395.2016.1155602.
- Smith, E. L., A. P. Abbott, and K. S. Ryder. 2014. “Deep Eutectic Solvents (DESs) and Their Applications.” Chemical Reviews 114 (21): 11060–11082. https://doi.org/10.1021/cr300162p.
- Sobati, M. A., A. M. Dehkordi, and M. Shahrokhi. 2010. “Extraction of Oxidized Sulfur-Containing Compounds of non-Hydrotreated Gas Oil.” Chemical Engineering & Technology 33 (9): 1515–1524. https://doi.org/10.1002/ceat.200900622.
- Song, C. 2003. “An Overview of new Approaches to Deep Desulfurization for Ultra-Clean Gasoline, Diesel Fuel and jet Fuel.” Catalysis Today 86 (1-4): 211–263. https://doi.org/10.1016/S0920-5861(03)00412-7.
- Song, C., and X. Ma. 2003. “New Design Approaches to Ultra-Clean Diesel Fuels by Deep Desulfurization and Deep Dearomatization.” Applied Catalysis B: Environmental 41 (1-2): 207–238. https://doi.org/10.1016/S0926-3373(02)00212-6.
- Srivastava, V. C. 2012. “An Evaluation of Desulfurization Technologies for Sulfur Removal from Liquid Fuels.” RSC Advances 2 (3): 759–783. https://doi.org/10.1039/C1RA00309G.
- Stepankova, V., P. Vanacek, J. Damborsky, and R. Chaloupkova. 2014. “Comparison of Catalysis by Haloalkane Dehalogenases in Aqueous Solutions of Deep Eutectic and Organic Solvents.” Green Chemistry 16 (5): 2754–2761. https://doi.org/10.1039/C4GC00117F.
- Tang, X. D., Y. F. Zhang, J. J. Li, Y. Q. Zhu, D. Y. Qing, and Y. X. Deng. 2015. “Deep Extractive Desulfurization with Arenium ion Deep Eutectic Solvents.” Industrial & Engineering Chemistry Research 54 (16): 4625–4632.
- Tiecco, M., F. Cappellini, F. Nicoletti, T. Del Giacco, R. Germani, and P. Di Profio. 2019. “Role of the Hydrogen Bond Donor Component for a Proper Development of Novel Hydrophobic Deep Eutectic Solvents.” Journal of Molecular Liquids 281: 423–430. https://doi.org/10.1016/j.molliq.2019.02.107.
- Wang, X., W. Jiang, W. Zhu, H. Li, S. Yin, Y. Chang, and H. Li. 2016. “A Simple and Cost-Effective Extractive Desulfurization Process with Novel Deep Eutectic Solvents.” Rsc Advances 6 (36): 30345–30352. https://doi.org/10.1039/C5RA27266A.
- Xu, H., D. Zhang, F. Wu, X. Wei, and J. Zhang. 2018. “Deep Desulfurization of Fuels with Cobalt Chloride-Choline Chloride/Polyethylene Glycol Metal Deep Eutectic Solvents.” Fuel 225: 104–110. https://doi.org/10.1016/j.fuel.2018.03.159.
- Yan, X., S. Anguille, M. Bendahan, and P. Moulin. 2019. “Ionic Liquids Combined with Membrane Separation Processes: A Review.” Separation and Purification Technology 222: 230–253. https://doi.org/10.1016/j.seppur.2019.03.103.
- Yang, H., B. Jiang, Y. Sun, L. Hao, Z. Huang, and L. Zhang. 2016. “Synthesis and Oxidative Desulfurization of Novel Lactam-Based Brønsted-Lewis Acidic Ionic Liquids.” Chemical Engineering Journal 306: 131–138. https://doi.org/10.1016/j.cej.2016.07.044.
- Yin, J., J. Wang, Z. Li, D. Li, G. Yang, Y. Cui, A. Wang, and C. Li. 2015. “Deep Desulfurization of Fuels Based on an Oxidation/Extraction Process with Acidic Deep Eutectic Solvents.” Green Chemistry 17 (9): 4552–4559. https://doi.org/10.1039/C5GC00709G.
- Yucui, H., Y. Congfei, and W. Weize. 2018. “Deep Eutectic Solvents: Green Solvents for Separation Applications.” Acta Physico-Chimica Sinica 34 (8): 873–885. https://doi.org/10.3866/PKU.WHXB201802062.
- Zaidi, W., A. L. Boisset, J. Jacquemin, L. Timperman, and M. R. M. Anouti. 2014. “Deep Eutectic Solvents Based on N-Methylacetamide and a Lithium Salt as Electrolytes at Elevated Temperature for Activated Carbon-Based Supercapacitors.” The Journal of Physical Chemistry C 118 (8): 4033–4042. https://doi.org/10.1021/jp412552v.
- Zhang, Y., F. Lü, X. Cao, and J. Zhao. 2014. “Deep Eutectic Solvent Supported TEMPO for Oxidation of Alcohols.” RSC Advances 4 (76): 40161–40169. https://doi.org/10.1039/C4RA05598E.
- Zhang, Q., K. D. O. Vigier, S. Royer, and F. Jérôme. 2012. “Deep Eutectic Solvents: Syntheses, Properties and Applications.” Chemical Society Reviews 41 (21): 7108–7146. https://doi.org/10.1039/c2cs35178a.
- Zhu, S., H. Li, W. Zhu, W. Jiang, C. Wang, P. Wu, Q. Zhang, and H. Li. 2016. “Vibrational Analysis and Formation Mechanism of Typical Deep Eutectic Solvents: An Experimental and Theoretical Study.” Journal of Molecular Graphics and Modelling 68: 158–175. https://doi.org/10.1016/j.jmgm.2016.05.003.