ABSTRACT
Vast mosaics of lakes, wetlands, and rivers on the Arctic Coastal Plain give the impression of water surplus. Yet long winters lock freshwater resources in ice, limiting freshwater habitats and water supply for human uses. Increasingly the petroleum industry relies on lakes to build temporary ice roads for winter oil exploration. Permitting water withdrawal for ice roads in Arctic Alaska is dependent on lake depth, ice thickness, and the fish species present. Recent winter warming suggests that more winter water will be available for ice- road construction, yet high interannual variability in ice thickness and summer precipitation complicates habitat impact assessments. To address these concerns, multidisciplinary researchers are working to understand how Arctic freshwater habitats are responding to changes in both climate and water use in northern Alaska. The dynamics of habitat availability and connectivity are being linked to how food webs support fish and waterbirds across diverse freshwater habitats. Moving toward watershed-scale habitat classification coupled with scenario analysis of climate extremes and water withdrawal is increasingly relevant to future resource management decisions in this region. Such progressive refinement in understanding responses to change provides an example of adaptive management focused on ensuring responsible resource development in the Arctic.
Introduction
Alaska’s Arctic Coastal Plain (ACP) covers approximately 75,000 km2 (roughly the size of South Carolina) with one major permanent road, the Dalton Highway, which tracks the Trans-Alaska Pipeline System (TAPS). In the winter, this road network expands considerably with roads made of ice and constructed from fresh surface water to facilitate new exploration (). Discovery of oil on Alaska’s North Slope in 1968 and subsequent passage of the Alaska Native Claims Settlement Act (ANCSA) in 1971 limited the ACP’s human footprint to Prudhoe Bay and eight Inupiaq communities (Coates Citation1993). Arctic sprawl of roads and pipelines has since expanded outward from Prudhoe Bay (Streever Citation2002), recently crossing the Colville River and extending into the National Petroleum Reserve in Alaska (NPR-A; USDOI-BLM Citation2014) about 100 km to the west (). The NPR-A was set aside in 1923 under the Naval Petroleum Reserve Production Act, and transferred from the U.S. Navy to the Bureau of Land Management in 1976 (Coates Citation1993) but has until recently remained untapped for its original purpose. New exploration into places such as the NPR-A happens almost exclusively during the winter via ice roads, leaving a vastly different and seemingly transient footprint compared to the smaller network of permanent roads connecting drilling pads and accessing pipelines.
Figure 1. Views of the human footprint on the Arctic Coastal Plain of northern Alaska in winter (A) and summer (B).
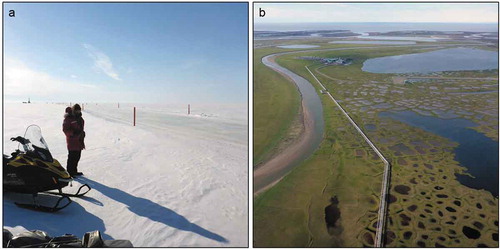
Figure 2. Physiographic, cultural, and land-management features of the North Slope of Alaska. Lakes are shown in blue and the Fish Creek Watershed is outlined in yellow.
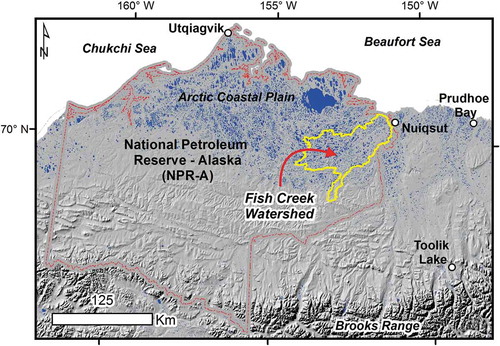
Temporary winter ice roads are designed to protect the tundra and underlying permafrost, while allowing exploration for new oil and gas reserves in more remote areas (Sibley et al. Citation2008). Water for ice-road construction in Alaska comes primarily from freshwater lakes (Jones et al. Citation2009a), which are highly abundant throughout much of the ACP, covering more than 20 percent of the land surface in many areas (Grosse, Jones, and Arp Citation2013). Ice roads have also long been used in Canada, Sweden, and Russia, but are primarily routed over networks of frozen rivers and lakes and thus rely much less on water extraction (Prowse et al. Citation2009). Winter water use has varied considerably from year to year since monitoring was required in Alaska’s NPR-A, but has increased dramatically in recent years with new oil exploration and discoveries (). Lake-water withdrawal for ice roads is regulated with the goal of protecting overwintering fish habitat for subsistence use and ecological integrity (Cott et al. Citation2008).
Figure 3. Lake water extracted in the northeastern NPR-A (approximately 70,000 ha land area) during the winters from 2008–2009 to present (2017–2018) as reported by ConocoPhillips-Alaska Inc. (CPAI) to the Bureau of Land Management’s Arctic District Office.
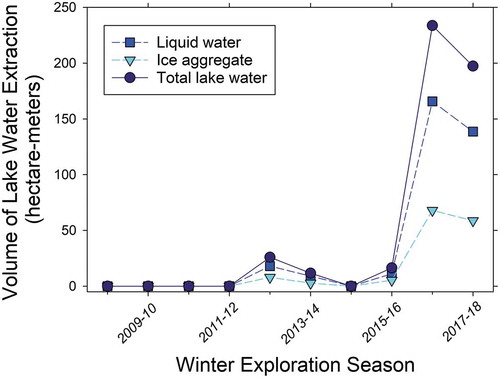
At the same time as the industrial footprint has expanded on the ACP, climate change has become an increasing concern in the Arctic, with warming much above global trends (Serreze and Barry Citation2011; Wendler, Moore, and Galloway Citation2014). Ecosystem responses to climate change on Alaska’s North Slope include earlier snowmelt (Stone et al. Citation2002), shrubification (Sturm, Racine, and Tape Citation2001), permafrost warming (Arp et al. Citation2016; Romanovsky, Smith, and Christiansen Citation2010) and degradation (Jorgenson, Shur, and Pullman Citation2006; Raynolds et al. Citation2014; Liljedahl et al. Citation2016), new tundra fires (Jones et al. Citation2009c), accelerating coastal erosion (Jones et al. Citation2009b), earlier breakup of lakes and rivers (Smejkalova, Edwards, and Dash Citation2017), and hydrologic intensification in the form of more variable watershed runoff (Rawlins et al. Citation2010; Stuefer et al. Citation2017). Climate-change impacts on native Inupiaq communities and the petroleum industry in northern Alaska are wide ranging with mixed consequences (Larsen et al. Citation2008; Raynolds et al. Citation2014; Brinkman et al. Citation2016). For example, Pacific salmon (Oncorhynchus spp.), with historically limited distributions in North Slope streams, may be extending their range and are now being harvested in several ACP villages (Nielson, Ruggerone, and Zimmerman Citation2013); simultaneously, traditional whitefish (Coregonus spp.) fisheries may be threatened by Saprolegniasis, a previously undocumented disease in the region (Sformo et al. Citation2017). Observed trends toward thinner lake ice are considered to be increasing winter water supply for ice-road construction (Arp et al. Citation2012a, Citation2018), yet warmer winters are shrinking the time period when ice roads can be built and utilized in northern Alaska and other regions of the Arctic (Prowse et al. Citation2009; Instanes et al. Citation2016).
The combined pressure of local industrial development and regional climate-change impacts will present ongoing challenges to multiple Arctic stakeholders (Walker et al. Citation1987; Martin et al. Citation2009; Streever et al. Citation2011). For government agencies charged with managing Arctic lands and resources, rapid changes in both climate and land use require adaptive management driven by interdisciplinary science teams with input from stakeholders (Wilby et al. Citation2010). Here, we review the integrated results of research focused on the 4,600 km2 Fish Creek Watershed (FCW) located in the northeast portion of the NPR-A (). This hydrologic unit encompasses most major freshwater habitats and many species of interest on the ACP (Whitman et al. Citation2011; Arp et al. Citation2012b), is an area valued for native subsistence hunting and fishing, and is the site of new petroleum development and exploration. These key characteristics and documented ecosystem responses to climate (e.g., Arp et al. Citation2016; Jorgenson, Shur, and Pullman Citation2006; Arp et al. Citation2012a; Engram et al. Citation2018) and land-use change (e.g., Lawson Citation1986) make the FCW an ideal setting to frame how freshwater ecosystems are responding and to show how adaptive management is addressing these competing pressures on the Arctic.
An important and related factor in our review of this research is that while oil development in the NPR-A only began in 2016, permitting and associated environmental monitoring and assessment was initiated in the FCW in 1998 (Whitman et al. Citation2011). Resulting environmental data sets and habitat studies that are focused on the FCW provided a major underpinning for much of the interdisciplinary research presented here.
Winter climate change and freshwater habitat responses
Strong empirical evidence for climate change in Arctic Alaska comes from the National Weather Service’s station at Utqiaġvik (formerly Barrow; ). Reliable climate records began there in 1921, and during a period of satellite sea-ice observations (1979–2012), which showed a marked decline in sea-ice extent, mean annual air temperature increased by 2.7°C (Wendler, Moore, and Galloway Citation2014). Mean annual precipitation has also shown an increasing trend during this period of sea-ice decline, albeit with considerable variability. The majority of this warming and wetting is occurring in the late fall and early winter in relation to declining sea-ice extent (Wendler, Moore, and Galloway Citation2014). Similar changes in climate are thought to be occurring across much of the ACP, including in the FCW, yet empirical evidence there is based on shorter station records starting in 1998 (USDOI-USGS Citation2014). To remedy this gap and anticipate future conditions, dynamically downscaled climate simulations using Polar Weather Research and Forecasting (Polar WRF) are used to analyze historic (1950–2005) and future (2006–2100) climate (Cai et al. Citation2018a). Polar WRF projections for the FCW indicate an additional 2°C of warming by 2100, dominated by winter warming with increased amounts and variability in precipitation (), particularly in the fall and late winter in relation to reduced sea-ice extent (Alexeev et al. Citation2016; Cai et al. Citation2018b).
Figure 4. Historic (1950–2014) and projected (2015–2100) changes in summer precipitation (A) and winter air temperature (B) for the Fish Creek Watershed using a regional climate model Polar WRF (Weather Research and Forecasting; Cai et al. Citation2018a). Historic normal (control year) and extreme drought and wet summers (A) and cold and warm winters (B) are used for creating scenarios to evaluate freshwater habitat responses.
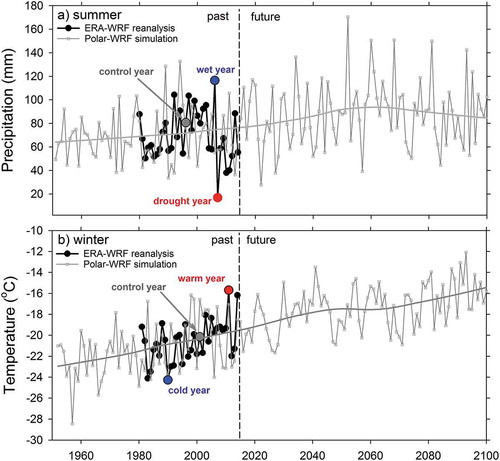
Winter warming is highly relevant to freshwater habitat responses because of impacts to lake-ice growth. In Arctic Alaska, lake ice traditionally grows thick, up to 2 m or more by winter’s end, resulting in large portions of shallow lakes that freeze solid with bedfast ice (Jeffries, Morris, and Liston Citation1996). Strong winter warming and more snowfall limits ice growth, and creates more liquid-water availability (Arp et al. Citation2012a; Alexeev et al. Citation2016). This dynamic has been documented using satellite-based synthetic aperture radar (SAR) to inventory the abundant thermokarst lakes of the Barrow Peninsula from 1992 to 2011 (Surdu et al. Citation2014). Engram et al. (Citation2018) recently extended this SAR analysis to 2016 and for the full range of lake-rich Arctic Alaska landscapes, including the FCW (). Despite progressive winter warming during this period, high year-to-year variability in the extent of floating ice lakes suggests that uncertainty in snowfall, late summer rainfall, and even sustained water withdrawal factor into winter habitat dynamics (Arp et al. Citation2018, Engram et al. Citation2018). Model experiments using Polar WRF show how reductions in late fall sea-ice extent can reduce early winter lake-ice growth: more open water generates more ocean-effect snowfall along coastal lowlands, insulating ice and preventing rapid thickening in the early winter (Alexeev et al. Citation2016). Changes in lake-ice regime (floating ice to bedfast ice) lead to later ice-out timing and lower sensitivity to drought conditions at the individual lake level (Arp et al. Citation2015a), and, at the regional level, a shift in the distribution of available habitat types (Arp et al. Citation2012a). The link between Arctic marine dynamics and freshwater habitat responses in adjacent coastal lowlands () underscores the complexity of forecasting feedbacks in the Arctic system (Serreze and Barry Citation2011) and the potential for future extreme events (e.g., ocean-effect snowstorms that can lead to very thin lake ice [Alexeev et al. Citation2016]).
Table 1. Comparison of lake-water supply and habitat characteristics among regions of the North Slope of Alaska including in the Fish Creek Watershed. The proportion of floating ice is based on a twenty-five-year (1992–2016) late-winter analysis using synthetic aperture radar (Engram et al. Citation2018) and maximum ice thickness is based on measured (Arp et al. Citation2018) and simulated values (Cai et al. Citation2018a).
Figure 5. The Beaufort Sea early winter sea-ice extent correlates with the floating-ice area of freshwater lakes (Surdu et al. Citation2014), linking changes in the marine system to freshwater habitat dynamics on the Arctic Coastal Plain of northern Alaska (after Alexeev et al. Citation2016).
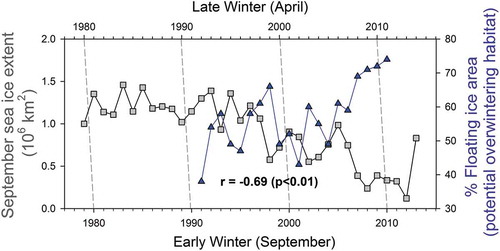
The availability of freshwater resources is likely increasing during the winter. Many historically bedfast-ice lakes now have perennial liquid water below floating ice, potentially expanding the overwintering fish habitat and increasing the winter water supply for ice roads (White et al. Citation2008; Jones et al. Citation2009a). Fine-scale (1.25 m resolution) SAR analysis of lakes, ponds, beaded streams, and river channels in the FCW reveals the full extent of potential overwintering habitats (; Jones et al. Citation2013), adding context to coarser resolution long-term analysis that shows a regime shift in this region’s lakes (Arp et al. Citation2012a; Engram et al. Citation2018).
Figure 6. Views of the Crea Creek Watershed in the summer from high-resolution aerial photography (A); in the winter from synthetic aperture radar (SAR), showing bedfast- and floating-ice lakes (B); and classification of freshwater habitats using a combination of SAR, other remotely sensing analysis, and field studies (C) (after Jones et al. Citation2013).
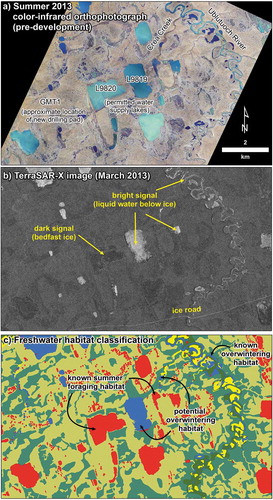
Winter water use and overwintering habitat
The technology for ice-road construction is constantly being adapted to changing transportation and economical demands and climate conditions (Masterson Citation2009; Prowse et al. Citation2009; Instanes et al. Citation2016). Nonetheless, regulations for winter water withdrawal have remained largely stagnant and are based on static water volumes relative to expected ice thickness for lakes with fish species considered either sensitive (e.g., broad whitefish [Coregonus nasus; ]) or nonsensitive (e.g., ninespine stickleback [Pungitius pungitius; ]); sensitivity is primarily with respect to dissolved oxygen levels (Sibley et al. Citation2008; Jones et al. Citation2009a). Extensive inventories of lake bathymetry and fish presence have been conducted on ACP lakes to permit winter lake-water withdrawal. If only nonsensitive species are present, up to 30 percent of under-ice water volume can be extracted in a given season. In lakes with summer populations of sensitive species, up to 15 percent of under-ice water volume can be extracted per year. For lakes where no fish species are present, typically isolated lakes with bedfast-ice regimes, 20 percent of the total water volume can be removed. In any of these cases, ice aggregate mechanically excavated from the lake surface may be used in addition to or instead of liquid water (), although the calculated maximum allowable volume remains the same. These amounts of lake water available for withdrawal are likely conservative for lakes where sensitive fish species have been captured, because water use guidelines assume up to 2.1 m of ice. This thickness is in comparison to assuming an ice thickness of 1.5 m for lakes where only resistant nonsensitive fish species have been captured, which may underestimate ice thickness in some years. Considering potential habitat use by fish species provides another level of protection, although current criteria to protect overwintering habitat is based on summer inventories of fish species (e.g., Cotts et al. Citation2008).
Figure 7. Examples of key organisms making up the freshwater food web of the Arctic Coastal Plain of northern Alaska. Photo credits: Hannah Uher-Koch (A), Matthew Whitman (B), Thomas Renicker (C), and Jason McFarland (D).
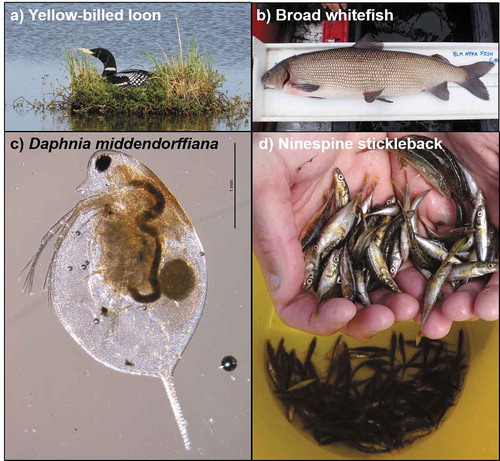
At least twelve species of fish inhabit the freshwaters of the FCW (Whitman et al. Citation2011) and their presence and abundance in ACP lakes and ponds is strongly linked to ice regime and connectivity (Haynes et al. Citation2014a; Jones et al. Citation2017). Species richness increases greatly with lake depth and connections to other water bodies, yet even bedfast-ice lakes can provide important summer foraging habitats (Haynes et al. Citation2014a; Heim et al. Citation2018). Although shallow waters freeze solid through the winter, if connected to stream systems or rivers during breakup flooding they readily become recolonized during the open-water season by fish, such as ninespine stickleback (), to take advantage of high productivity from abundant large-bodied zooplankton, such as Daphnia middendorffiana (; Laske et al. Citation2017; Beaver et al. in review). If connectivity to stream systems permits, productive bedfast-ice lakes also provide important foraging opportunities for piscivorous fishes, such as arctic grayling (Thymallus arcticus; Heim et al. Citation2018). Shallow bedfast-ice lakes also provide important foraging habitat for planktivorous shorebirds (e.g., red-necked phalarope [Phalaropus lobatus]) and piscivorous birds (e.g., yellow-billed loons [Gavia adamsii; ] and arctic terns [Sterna paradisaea]). Thus, winter conditions drive variability in overwintering habitat and also set the stage for habitat availability and quality in the following summer with both ice regimes (bedfast-ice and floating-ice lakes) providing important, but very different, forage bases for fish and waterbird species (Beaver et al. in review). Understanding the distribution of these lake habitats across diverse ACP landscapes and how they are responding to winter climate change (Engram et al. Citation2018) provides spatially explicit guidance for managing aquatic resources at broader scales.
Managing lake-water supply based on natural ranges of variability can also have advantages over static assessments (Instanes et al. Citation2016). Recent multitemporal SAR analysis of late winter-ice regimes during a twenty-five-year period shows that liquid water availability can vary substantially from year to year, as well as regionally, in Arctic Alaska (Engram et al. Citation2018). For example, in the upper portion of the FCW, floating-ice extent averaged 54 percent of total lake area and varied by 18 percent, corresponding to approximately 0.7 m difference in lake-ice thickness during this same period (). Other regions of the ACP have shallower lake depth distributions, but higher ranges of variation in floating-ice extent and ice thickness. This range of variability suggests that more under-ice water could be made available than static bathymetry assessments currently permit, depending of course on whether current under-ice water volume proportions (e.g., 30 percent for nonsensitive species and 15 percent for sensitive species) have a solid scientific basis, and this information could be brought to bear on specific regions or lakes to refine winter water management (Jones et al. Citation2017). This range of variability also provides guidance for protecting overwintering habitats and lake-recharge potential by ensuring that the minimum ranges of lake-water availability are not exceeded ().
Still, knowing where and how Arctic fish overwinter remains largely a mystery in ACP waters, adding high levels of uncertainty in managing both water withdrawal and fish communities (Cott et al. Citation2008). Using radio telemetry to track arctic grayling, broad whitefish, and burbot (Lota lota) through winter in the FCW showed that many fish species overwinter in deep pools of alluvial river channels and a few lakes with strong connections to river systems (Morris Citation2003). Movement studies of arctic grayling during multiple summers in a small tributary (Crea Creek) of the Ublutuoch River in FCW () reveal out-migration of these fish to larger river channels cued by declining flows and freezing temperatures (Heim et al. Citation2016). Considering connected overwintering habitats (i.e., river systems) compared to isolated pockets of liquid water in ponds and beaded streams (; Jones et al. Citation2013) highlights that understanding how overwintering habitats are arrayed in freshwater systems should be incorporated into winter water management (Jones et al. Citation2017). Because fish disperse widely into shallow, seasonally frozen habitats such as beaded streams and bedfast-ice lakes in the summer (Heim et al. Citation2016, Citation2018), maintaining adequate stream flow and depth for their return to perennial overwintering habitats is an important consideration. Bedfast-ice lakes are most sensitive to drought conditions (Arp et al. Citation2015a; ) and as currently managed are the lakes where the highest proportion of water withdrawal is permitted—up to 20 percent of total volume. More broadly, current permitting of winter water withdrawal considers lakes as isolated hydrologic units, but future management could be improved by accounting for how lakes are linked to other habitats (i.e., downstream rivers) to maintain connectivity and the availability of complimentary habitats to support food webs and the range of seasonal movement.
Figure 8. Examples of the scales at which winter water withdrawal from lakes and freshwater habitats can be managed using a Winter Water Supply Index based on a lake habitat classification system for the Fish Creek Watershed (Jones et al. Citation2017).
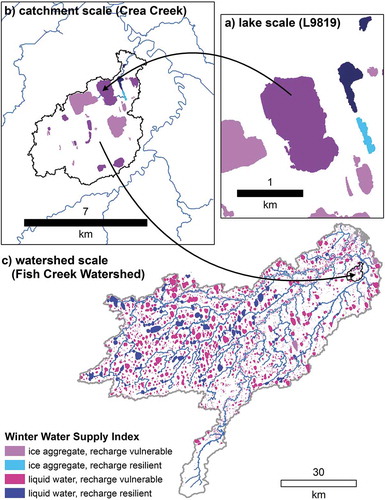
Managing for habitat diversity and connectivity
In Arctic landscapes dominated by lakes, connectivity among freshwater habitats is highly predictive of fish species presence (Haynes et al. Citation2014a) and diversity (Hershey et al. Citation1999; Lesack and Marsh Citation2010; Laske et al. Citation2017). A wide range of other ecosystem attributes (i.e., productivity and water chemistry) also corresponds to the degree to which lakes are linked by surface flows to other aquatic systems in the Arctic (Lesack and Marsh Citation2010) and other regions (e.g., Jones Citation2010), making connectivity an essential attribute to consider for watershed- or landscape-scale management of freshwater habitat. Yellow-billed loons (YBLO; ), for example, are an avian species of interest on the ACP because of their low population size, their restricted breeding range, and the probability that resource extraction may encroach into high-density nesting areas (Schmidt, Flamme, and Walker Citation2014). Inventory and behavioral studies of YBLO and Pacific loons (PALO) in the FCW suggest that preferred nesting habitats on lake shorelines are related to connectivity, lake depth, and lake area (Jones et al. Citation2017), similar to other regions of Arctic Alaska (Earnst, Platte, and Bond Citation2006; Schmidt, Flamme, and Walker Citation2014). Predicting which lakes will be used for nesting by these species relates both to interspecific competition between YBLO and PALO and the abundance of forage fish populations, such as ninespine stickleback and Alaska blackfish (Haynes et al. Citation2014b). Ninespine stickleback () in particular shape Arctic food webs by supporting a wide range of upper trophic levels and regulating lower-level plankton communities (Laske et al. Citation2017; Beaver et al. in review). In Crea Creek, for example (), large arctic grayling are predominantly piscivorous, consuming large quantities of ninespine stickleback (McFarland, Wipfli, and Whitman Citation2017).
The abundance and composition of plankton communities in Arctic lakes integrate much information about how the system supports higher trophic levels and responds to physical habitat changes. Although Arctic freshwater ecosystems are often considered oligotrophic (low productivity), shallow depths and terrestrial subsidies from dynamically eroding shorelines associated with thermokarst lakes can produce a wide range of variation in nutrient status and productivity (Kokelj, Zajdlik, and Thompson Citation2009; Larsen et al. Citation2017). However, seasonal and multiyear analyses of zooplankton data show that biomass and community composition strongly relate to lake connectivity, suggesting top-down control of food-web dynamics (Beaver et al. in review). This research also points to zooplankton sensitivity to water temperature and ice-out timing, such that continued response to Arctic climate change should enhance freshwater productivity and cause cascading effects through Arctic food webs.
An improved understanding of lake food-web dynamics relative to position in the watershed and changes in hydrology, whether the result of regional changes in climate or local lake-water withdrawal, can help guide better management of Arctic freshwater habitats. Having the ability to predict fish species presence or absence based on zooplankton species composition (Beaver et al. in review) could also expand the scope of regulating lake-water withdrawal, going beyond fish species to include their food webs and supporting ecosystem processes. Rigorous multigear fish-sampling methods are time consuming and costly, although regionally validated occupancy models derived from such efforts present a useful alternative (Haynes et al. Citation2014a). Industry is required to determine only if sensitive and resistant species are present, not to characterize the entire fish community at water-source lakes or the forage base they rely on. Detecting fish species presence using environmental DNA (eDNA) methods is also being explored for Arctic freshwater systems both at the lake and watershed scale (Klobucar, Rodgers, and Budy Citation2017), including the FCW, potentially leading to more integrated and comprehensive assessments of fish habitat use and understanding of how freshwater habitats can be managed at broader scales.
Moving beyond individual lake-based management of winter water withdrawal is increasingly facilitated by a new lake-based classification in the FCW. By combining attributes of lake depth with stream connectivity, a winter water supply index (WWSI) has been proposed (Jones et al. Citation2017). The WWSI differentiates lakes into four classes based on water availability and potential for lake recharge (). Taken at the scale of an individual lake along a proposed ice-road route (), this provides initial guidance as to (1) whether liquid water or only ice chips should be available and (2) if water is extracted, how much can be taken without jeopardizing lake recharge and downstream flows during the following summer. This second consideration of recharge potential and downstream connectivity can then be evaluated by hydrographic analysis of lake setting relative to stream networks, downstream rivers, and other lakes in its catchment (). For example, if a lake permitted for water withdrawal has no connection to downstream river systems used by overwintering fishes, then this habitat may be considered of lower value for summer foraging. Alternatively, if other lakes in the catchment have similar habitat characteristics as the permitted lake, these could serve to augment the availability of summer foraging habitat. The integration of predicted food-web characteristics into such a classification, using plankton data, for example (Beaver et al. in review), could greatly expand the value of this lake-based classification toward more holistic ecosystem management. Taking the WWSI to the full watershed scale () provides similar guidance to the previous example and may also prove valuable in planning and permitting ice-road routes over longer distances. Expanding the ability to both maximize winter water supply and mitigate the loss or reduction of key habitat types should be an outcome of taking a watershed-scale approach to the management of Arctic lakes.
Scenarios analysis to address uncertainty in future climate and land use
Uncertainty is a fundamental issue in adaptation to climate change (Wilby et al. Citation2010). Region-specific considerations and approaches are necessary to best account for future ranges of variability (Instanes et al. Citation2016). For Arctic freshwater ecosystems, predicting responses requires bracketing the future ranges of variation in hydrology and ice regimes. Dynamically downscaled simulations of climate for the FCW show historic and projected ranges of variability in summer precipitation and winter air temperature (Cai et al. Citation2018a; )—first-order controls on hydrologic and ice regimes, respectively.
Hydrologic intensification is a great source of uncertainty for Arctic freshwater systems (Wrona et al. Citation2016). In general, this process occurs because warmer air holds more water, thus resulting in more precipitation, but also that warmer air causes more evapotranspiration, such that both fluxes may increase (Rawlins et al. Citation2010). Strong warming coupled with more open-water extent from sea-ice decline make Arctic coastlands particularly susceptible to hydrologic intensification, which is generally expected to result in wetter conditions (Cai et al. Citation2018a). A wetting trend in the Arctic does not however eliminate the potential for extreme droughts (Instanes et al. Citation2016). Climate projections from Polar WRF show a wetting trend for the ACP, but with many extreme dry years interspersed (). The summer drought of 2007 is prominent in the recent history of the Alaskan North Slope, resulting in a major tundra fire (Jones et al. Citation2009c), shrinkage of lakes (Jones et al. Citation2009a), and reduced river runoff and hydrologic connectivity (Arp et al. Citation2012b; Betts and Kane Citation2015; Stuefer et al. Citation2017).
How should winter water withdrawal be managed considering that average projections suggest not only that the next summer will be wetter than normal but also that extreme drought is a real possibility? A first-order approach to addressing this uncertainty is to develop climate scenarios that assess extreme cases of wet and dry years based on past records, thus providing a full and realistic range of potential responses (Wilby et al. Citation2010). Using Polar WRF simulations for the FCW (Cai et al. Citation2018a), single- to multiyear drought scenarios have been developed relative to region-specific climate normals by selecting representative years from the past record (; Gädeke et al. Citation2016). Examining the response to a particular extreme year has obvious benefits in communicating results to stakeholders who may have direct experience with those conditions and how ecosystems responded. Developing scenarios based on recent and observed climate behavior present simple, realistic sets of conditions to evaluate, such that results can be framed based on known occurrences. The use of model experiments for observed years with contrasting sea-ice conditions in the Beaufort Sea to evaluate freshwater lake responses (Alexeev et al. Citation2016) provides an excellent example of using scenarios based on actual data to isolate processes and provide a realistic context for communicating potential outcomes to managers and decision makers (Wilby et al. Citation2010).
To evaluate freshwater habitat responses to both climate and water extraction scenarios, a physically based watershed hydrology model, Water Balance Simulation Model (WaSiM), was employed to simulate streamflow and connectivity. WaSiM was developed at the local scale for the Crea Creek Watershed (30 km2), a focal area for new oil development (; Gädeke et al. Citation2016), and at a regional hydrologic scale, the entire FCW (4,600 km2; ; Daanen et al. Citation2017) for a set of extreme climate scenarios. WaSiM has also recently been used to evaluate changes in connectivity in ice-wedge networks under differing scenarios of degradation and snow cover to assess runoff responses, and then extended to pan-Arctic scales (Liljedahl et al. Citation2016). Scenarios of lake-water withdrawal are also being simulated to evaluate the impact on downstream flows in the preceding summer and longer term relative to controlled climate conditions (). Combining scenarios of water withdrawal with climate scenarios (i.e., multiyear drought) are then being used to address the uncertainty with permitting winter water withdrawal across a range of possible summer recharge scenarios. New oil-drilling infrastructure (Greater Moose’s Tooth 1) is now in operation adjacent to Crea Creek as of 2018, which relies on water from lakes () that feed downstream flows (Arp et al. Citation2015b) and maintain connectivity among fish habitats (Heim et al. Citation2016, Citation2018). Simulations using WaSiM are providing a timely analysis of scenarios directly applicable to the resource management of this system, and are likely representative of other freshwater habitats of ACP watersheds ().
Figure 9. Example of freshwater habitat response (stream–lake system) to scenarios of drought, water withdrawal, and their combined effects on streamflow relative to a control climate scenario (based on Gädeke et al. Citation2016).
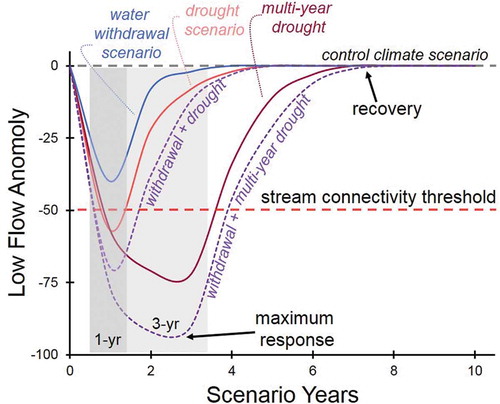
Applying this understanding of habitat responses to extreme climate scenarios also provides the needed dynamic element to the FCW lake-habitat classification (Jones et al. Citation2017). Bracketing the variation in potential lake recharge and connectivity to downstream freshwater habitats across a range of lake types throughout the watershed greatly expands our ability to efficiently manage the habitat and species at an appropriate scale. Being able to maximize winter water supply and mitigate the impact on freshwater habitats will increasingly rely on spatially explicit process-based frameworks. Once in place, the use of habitat classification coupled with targeted field studies and model simulations of habitat responses provide a framework for adaptive management that can be responsive to anticipated concerns (i.e., multiyear drought) and available for future unanticipated needs (e.g., exploration and development in new watersheds).
Adaptive management in Arctic watersheds
Applying adaptive management to land use and climate change relies on scientists from multiple disciplines and agencies working interactively with stakeholders to address both longstanding (e.g., tundra impacts from development) and emerging (e.g., freshwater habitat connectivity) issues (Martin et al. Citation2009; Wilby et al. Citation2010; Streever et al. Citation2011). Managing ice roads and the freshwater habitats that they are built from (i.e., lakes and connected watersheds) in the NPR-A is only one example of the complexities of science-informed decision making in the Arctic. Challenges related to permafrost degradation, flooding, and coastal erosion are currently impacting industry and native village infrastructure (Streever et al. Citation2011; Raynolds et al. Citation2014; Toniolo et al. Citation2017). Maintaining populations of subsistence fish and wildlife (Martin et al. Citation2009) and a strong subsistence culture in native communities will be ongoing struggles for Arctic Alaska (Brinkman et al. Citation2016).
Changes in climate and land use will continue to cause a range of responses in the freshwater ecosystems of the FCW and elsewhere on the ACP. Through ongoing efforts, habitat responses are becoming better understood and more predictable, allowing management to be more responsive and effective despite uncertainties surrounding future climate and land use. Several examples presented in this research synthesis demonstrate management decisions already facilitated by such efforts. Recent evidence of shifting lake-ice regimes (Arp et al. Citation2012a; Alexeev et al. Citation2016; Engram et al. Citation2018) and their hydrologic consequences (Arp et al. Citation2015a) are being incorporated into how lakes are being permitted for winter water withdrawal in the NPR-A. This example of adaptive management in action is facilitated through a new lake-based classification system developed specifically for the FCW by a multidisciplinary, multiagency science team (Jones et al. Citation2017). Studies of fish habitat use and migration timing (Heim et al. Citation2016, Citation2018) coupled with hydrologic modeling (Gädeke et al. Citation2016) are providing site-specific information for guiding infrastructure design to help maintain habitat connectivity and fish passage and to inform the impact of water use on downstream flows through a range of climate conditions. Results from these studies and ongoing research in the FCW have relied heavily on long-term monitoring data and collaboration between land-management agencies and government and academic scientists. The adaptive management process in the FCW, and interdisciplinary science in general, is necessarily slow and has required a great deal of patience to merge concepts and methods at a level applicable to management decisions.
The strength of multidisciplinary science and adaptive management in a time of rapid changes in both climate and land use is certainly being put to the test in the NPR-A with recent expansion of exploration and development. Continually testing the efficacy of adaptive management in this and other settings is how the process will be improved. The 2017 opening of the 1002 area coastal plain of the Arctic National Wildlife Refuge (ANWR) to oil and gas exploration and development is a perfect example of an unexpected change in land management where existing long-term monitoring data sets and already functioning multidisciplinary research teams would facilitate sound adaptive management. The degree to which science-based adaptive management works in this setting (ANWR’s coastal plain) may provide a very contrasting case study to the approach taken in the NPR-A. We suggest that supporting long-term and progressive adaptive management frameworks based on multidisciplinary science teams, such as an integrated monitoring and research program in the Fish Creek Watershed, is a robust strategy to address environmental uncertainty and to provide for specific science-based environmental policy.
Acknowledgments
This project arose from the creative, forward-thinking leadership of Arctic Landscape Conservation Cooperative’s science coordinator, Philip Martin, who lead the effort to organize and support projects such as Fish CAFE. The ALCC’s Species and Habitat Working Group catalyzed this effort and additional ALCC leadership from Drs. Dave Payer and Wendy Loya helped see it through to completion. C. Hiemstra, M. Wipfli, and E. Torvinen contributed to the early portion of the Fish CAFE interdisciplinary science process. The Bureau of Land Management’s Arctic District Office and the U.S. Fish and Wildlife Service’s Fairbanks Fish and Wildlife Conservation Office contributed important personnel and logistical resources in addition to direct funding. Additional support was provided by the U.S. Geological Survey, Alaska Department of Natural Resources, and grants from the National Science Foundation, specifically Alaska EPSCoR Northern Test Case (OIA-1208927) and Arctic Systems Science Program (OPP-1417300). Any use of trade, product, or firm names is for descriptive purposes only and does not imply endorsement by the U.S. Government.
Disclosure statement
No potential conflict of interest was reported by the authors.
Additional information
Funding
References
- Alexeev, V. A., C. D. Arp, B. M. Jones, and L. Cai. 2016. Arctic sea ice decline contributes to thinning lake ice trend in northern Alaska. Environmental Research Letters 11 (7):1–9. doi:10.1088/1748-9326/11/7/074022.
- Arp, C. D., B. M. Jones, A. K. Liljedahl, K. M. Hinkel, and J. A. Welker. 2015a. Depth, ice thickness, and ice-out timing cause divergent hydrologic responses among Arctic lakes. Water Resources Research 51 (12):9379–401. doi:10.1002/2015WR017362.
- Arp, C. D., B. M. Jones, G. Grosse, A. C. Bondurant, V. E. Romanovsky, K. M. Hinkel, and A. D. Parsekian. 2016. Threshold sensitivity of shallow Arctic lakes and sublake permafrost to changing winter climate. Geophysical Research Letters 43 (12):6358–65. doi:10.1002/2016GL0868506.
- Arp, C. D., B. M. Jones, M. Engram, V. Alexeev, L. Cai, A. Parsekian, K. M. Hinkel, A. C. Bondurant, and A. L. T. Creighton. 2018. Contrasting lake ice responses to winter climate indicate future variability and trends on the Alaskan Arctic Coastal Plain. Environmental Research Letters. doi:10.1088/1748-9326/aae994.
- Arp, C. D., B. M. Jones, Z. Lu, and M. S. Whitman. 2012a. Shifting balance of lake ice regimes on the Arctic Coastal Plain of northern Alaska. Geophyscial Research Letters 39 (L16503):1–5. doi:10.1029/2012GL052518.
- Arp, C. D., M. S. Whitman, B. M. Jones, G. Grosse, B. V. Gaglioti, and K. C. Heim. 2015b. Distribution and biophysical processes of beaded streams of Arctic permafrost landscapes. Biogeosciences 12:1–19. doi:10.5194/bg-12-29-2015.
- Arp, C. D., M. S. Whitman, B. M. Jones, R. Kemnitz, G. Grosse, and F. E. Urban. 2012b. Drainage network structure and hydrologic behavior of three lake-rich watersheds on the Arctic Coastal Plain, Alaska. Arctic, Antarctic, and Alpine Research 44 (3):385–98. doi:10.1657/1938-4246-44.4.385.
- Beaver, J., C. D. Arp, B. M. Jones, M. S. Whitman, T. Renciker, E. Samples, D. Ordosch, K. Scotess. In Review. Top-down control of plankton community composition and size structure in Arctic lakes reveal responses to changing Ice and hydrologic connectivity. Freshwater Biology.
- Betts, E. D., and D. L. Kane. 2015. Linking North Slope of Alaska climate, hydrology, and fish migration. Hydrology Research 46 (4):578–90. doi:10.2166/nh.2014.031.
- Brinkman, T. J., W. D. Hansen, F. S. Chapin, G. Kofinas, S. BurnSilver, and T. S. Rupp. 2016. Arctic communities perceive climate impacts on access as a critical challenge to availability of subsistence resources. Climatic Change 139 (3):413–27. doi:10.1007/s10584-016-1819-6.
- Cai, L., V. A. Alexeev, C. D. Arp, B. M. Jones, A. K. Liljedahl, and A. Gädeke. 2018a. The Polar WRF downscaled historical and projected twenty-first century climate for the coast and foothills of Arctic Alaska. Frontiers in Earth Science 5 (111). doi: 10.3389/feart.2017.00111.
- Cai, L., V. A. Alexeev, C. D. Arp, B. M. Jones, and V. E. Romanovsky. 2018b. Modeling the impacts of projected sea ice decline on the low atmosphere and near-surface permafrost on the North Slope of Alaska. International Journal of Climatology 1–14. doi:10.1002/joc.5741.
- Coates, P. A. 1993. The Trans-Alaska Pipeline controversy: Technology, conservation, and the frontier. Fairbanks, AK: University of Alaska Press.
- Cott, P. A., P. K. Sibley, W. M. Somers, M. R. Lilly, and A. M. Gordon. 2008. A review of water level fluctuations on aquatic biota with an emphasis on fishes in ice-covered lakes. Journal of the American Water Resources Association 44 (2):343–59. doi:10.1111/j.1752-1688.2007.00166.x.
- Daanen, R. P., A. Gaedeke, A. K. Liljedahl, C. D. Arp, M. S. Whitman, B. M. Jones, L. Cai, and V. A. Alexeev. 2017. Simulating low-flow conditions in an arctic watershed using WaSiM. American Geophysical Union Fall Meeting, New Orleans, LA.
- Earnst, S. L., R. A. Platte, and L. Bond. 2006. A landscape-scale model of yellow-billed loon (Gavia adamsii) habitat preferences in northern Alaska. Hydrobiologia 567:227–36. doi:10.1007/s10750-006-0042-2.
- Engram, M., C. D. Arp, B. M. Jones, O. A. Ajadi, and F. J. Meyer. 2018. Analyzing floating and bedfast lake ice regimes across Arctic Alaska using 25 years of space-borne SAR imagery. Remote Sensing of Environment 209:660–76. doi:10.1016/j.rse.2018.02.022.
- Gädeke, A., A. K. Liljedahl, C. D. Arp, R. D. Daanen, V. A. Alexeev, L. Cai, and M. S. Whitman. 2016. Process based hydrological modeling to assess changes in surface water connectivity in an Arctic watershed. Arctic Observing Summit, Fairbanks, Alaska
- Grosse, G., B. Jones, and C. Arp. 2013. Thermokarst lake, drainage, and drained basins. In Treatise on geomorphology, ed. J. Shroder, R. Giardino, and J. Harbor, 1–29. San Diego, CA: Academic Press.
- Haynes, T. B., A. E. Rosenberger, M. S. Lindberg, M. Whitman, and J. A. Schmutz. 2014a. Patterns of lake occupancy by fish indicate different adaptations to life in a harsh Arctic environment. Freshwater Biology 59 (9):1884–96. doi:10.1111/fwb.12391.
- Haynes, T. B., J. A. Schmutz, M. S. Lindberg, K. G. Wright, B. D. Uher-Koch, and A. E. Rosenberger. 2014b. Occupancy of yellow-billed and Pacific loons: Evidence for interspecific competition and habitat mediated co-occurrence. Journal of Avian Biology 45 (3):296–304. doi:10.1111/jav.2014.45.issue-3.
- Heim, K. C., C. D. Arp, M. S. Whitman, and M. S. Wipfi. 2018. The complementary role of lentic and lotic habitats for Arctic grayling in a complex stream-lake network in Arctic Alaska. Ecology of Freshwater Fish. doi:10.1111/eff.12444.
- Heim, K. C., M. S. Wipfli, M. S. Whitman, C. D. Arp, J. Adams, and J. A. Falke. 2016. Seasonal cues of Arctic grayling movement in a small Arctic stream: The importance of surface water connectivity. Environmental Biology of Fishes 99 (1):49–65. doi:10.1007/s10641-015-0453-x.
- Hershey, A. E., G. M. Gettel, M. E. McDonald, M. C. Miller, H. Mooers, W. J. O’Brien, J. Pastor, C. Richards, and J. A. Schuldt. 1999. A geomorphic-trophic model for landscape controls of Arctic lake food webs. Bioscience 49 (11):887–97. doi:10.2307/1313648.
- Instanes, A., V. Kokorev, R. Janowicz, S. K. Bruland, and T. Prowse. 2016. Changes to freshwater systems affecting Arctic infrastructure and natural resources. Journal of Geophysical Research: Biogeosciences 121:567–85. doi:10.1002/2015JG003125.
- Jeffries, M. O., K. Morris, and G. E. Liston. 1996. A method to determine lake depth and water availability on the North Slope of Alaska with spaceborne imaging radar and numerical ice growth modelling. Arctic 49 (4):367–74. doi:10.14430/arctic1212.
- Jones, B. M., A. Gusmeroli, C. D. Arp, T. Strozzi, G. Grosse, B. V. Gaglioti, and M. S. Whitman. 2013. Classification of freshwater ice conditions on the Alaskan Arctic Coastal Plain using ground penetrating radar and TerraSAR-X satellite data. International Journal of Remote Sensing 34 (23):8267–79. doi:10.1080/2150704X.2013.834392.
- Jones, B. M., C. A. Kolden, R. Jandt, J. T. Abatzoglou, F. Urban, and C. D. Arp. 2009c. Fire behavior, weather, and burn severity of the 2007 Anaktuvuk River Tundra fire, North Slope, Alaska. Arctic Antarctic and Alpine Research 41 (3):309–16. doi:10.1657/1938-4246-41.3.309.
- Jones, B. M., C. D. Arp, K. M. Hinkel, R. A. Beck, J. A. Schmutz, and B. Winston. 2009a. Arctic lake physical processes and regimes with implications for winter water availability and management in the National Petroleum Reserve Alaska. Environmental Management 43 (6):1071–84. doi:10.1007/s00267-008-9241-0.
- Jones, B. M., C. D. Arp, M. S. Whitman, D. Nigro, I. Nitze, J. Beaver, A. Gadeke, C. Zuck, A. K. Liljedahl, R. Daanen, et al. 2017. A lake cover classification to guide research and inform management decisions in an arctic watershed in northern Alaska experiencing climate and land-use change. Ambio 46 (7):769–86. doi:10.1007/s13280-017-0915-9.
- Jones, B. M., C. D. Arp, M. T. Jorgenson, K. M. Hinkel, J. A. Schmutz, and P. L. Flint. 2009b. Increase in the rate and uniformity of coastline erosion in Arctic Alaska. Geophyscial Research Letters 36 (L03503):1–5.
- Jones, C. E. 2010. Incorporating lakes within the river discontinuum: Longitudinal changes in ecological characteristics in stream–lake networks. Canadian Journal of Fisheries and Aquatic Science 67:1350–62. doi:10.1139/F10-069.
- Jorgenson, M. T., Y. L. Shur, and E. R. Pullman. 2006. Abrupt increase in permafrost degradation in Arctic Alaska. Geophysical Research Letters 33 (L02503):1–4. doi:10.1029/2005GL024960.
- Klobucar, S. L., T. W. Rodgers, and P. Budy. 2017. At the forefront: Evidence of the applicability of using environmental DNA to quantify the abundance of fish populations in natural lentic waters with additional sampling considerations. Canadian Journal of Fisheries and Aquatic Sciences 74. doi:10.1139/cjfas-2017-0114.
- Kokelj, S. V., B. Zajdlik, and M. S. Thompson. 2009. The impact of thawing permafrost on the chemistry of lakes across the subarctic boreal-tundra transition, Mackenzie Delta Region, Canada. Permafrost and Periglacial Processes 20:185–99. doi:10.1002/ppp.641.
- Larsen, A. S., J. A. O’Donnell, J. H. Schmidt, H. J. Kristenson, and D. K. Swanson. 2017. Physical and chemical characteristics of lakes across heterogeneous landscapes in arctic and subarctic alaska. Journal Of Geophysical Research: Biogeosciences 122 (4):989–1008.
- Larsen, P. H., S. Goldsmith, O. Smith, M. L. Wilson, K. Strzepek, P. Chinowsky, and B. Saylor. 2008. Estimating future costs of Alaska public infrastructure at risk from climate change. Global Environmental Change 18 (3):442–57. doi:10.1016/j.gloenvcha.2008.03.005.
- Laske, S. M., A. E. Rosenberger, W. J. Kane, M. S. Wipfli, and C. E. Zimmerman. 2017. Top-down control of invertebrates by ninespine stickleback in arctic ponds. Freshwater Science 36 (1):124–37. doi: 10.1086/690675.
- Lawson, D. E. 1986. Response of permafrost terrain to disturbance: A synthesis of observations from northern Alaska, USA. Arctic and Alpine Research 18:1–17.
- Lesack, L. F. W., and P. Marsh. 2010. River-to-lake connectivities, water renewal, and aquatic habitat diversity in the Mackenzie River delta. Water Resources Research 46 (W12504):1–16. doi:10.1029/2010WR009607.
- Liljedahl, A. K., J. Boike, R. P. Daanen, A. N. Fedorov, G. V. Frost, G. Grosse, L. Hinzman, Y. Iijma, J. C. Jorgenson, N. Matveyeva, et al. 2016. Pan-Arctic ice-wedge degradation in warming permafrost and its influence on tundra hydrology. Nature Geoscience 9 (4):312-+. doi:10.1038/ngeo2674.
- Martin, P. D., J. L. Jenkins, F. J. Adams, M. T. Jorgenson, A. C. Matz, D. C. Payer, P. E. Reynolds, A. C. Tidwell, and J. R. Zelenak. 2009. Wildlife response to environmental Arctic change: Predicting future habitats for Arctic Alaska. Fairbanks: U.S. Fish and Wildlife Service.
- Masterson, D. M. 2009. State of the art of ice bearing capacity and ice construction. Cold Regions Science and Technology 58 (3):99–112. doi:10.1016/j.coldregions.2009.04.002.
- McFarland, J. J., M. S. Wipfli, and M. S. Whitman. 2017. Trophic pathways supporting Arctic grayling in a small stream on the Arctic Coastal Plain, Alaska. Ecology of Freshwater Fish. doi:10.1111/eff.12336.
- Morris, W. 2003. Seasonal movements and habitat use fo Arctic grayling (Thymallus arcticus), burbot (Lota lota), and broad whitefish (Coregonus nasus) within the fish creek drainage of the National Petroleum Reserve—Alaska, 2001–2002. Technical Report No. 03-02. Alaska Department of Natural Resources, Office of Habitat management and Permitting.
- Nielsen, J. L., G. T. Ruggerone, and C. E. Zimmerman. 2013. Adaptive strategies and life history characteristics in a warming climate: Salmon in the Arctic? Environmental Biology of Fishes 96 (10):1187–226.
- Prowse, T. D., C. Furgal, R. Chouinard, H. Melling, D. Milburn, and S. L. Smith. 2009. Implications of climate change for economic development in northern Canada: Energy, resources, and transportation sectors. Ambio 38 (5):272–81.
- Rawlins, M. A., M. Steele, M. M. Holland, J. C. Adam, J. E. Cherry, J. A. Francis, P. Y. Groisman, L. D. Hinzman, T. G. Huntington, D. J. Kane, et al. 2010. Analysis of the Arctic system for freshwater cycle intensification: Observations and expectations. Journal of Climate 23:5715–37. doi:10.1175/2010JCLI3421.1.
- Raynolds, M. K., D. A. Walker, K. J. Ambrosius, J. Brown, K. R. Everett, M. Kanevskiy, G. P. Kofinas, V. E. Romanovsky, Y. Shur, and P. J. Webber. 2014. Cumulative geoecological effects of 62 years of infrastructure and climate change in ice-rich permafrost landscapes, Prudhoe Bay Oilfield, Alaska. Global Change Biology 20 (4):1211–24. doi:10.1111/gcb.12500.
- Romanovsky, V. E., S. L. Smith, and H. H. Christiansen. 2010. Permafrost thermal state in the polar Northern Hemisphere during the international polar year 2007–2009: A synthesis. Permafrost and Periglacial Processes 21:106–16. doi:10.1002/ppp.689.
- Schmidt, J. H., M. J. Flamme, and J. Walker. 2014. Habitat use and population status of Yellow-billed and Pacific loons in western Alaska, USA. Condor 116 (3):483–92. doi:10.1650/CONDOR-14-28.1.
- Serreze, M. C., and R. G. Barry. 2011. Processes and impacts of Arctic amplification: A research synthesis. Global and Planetary Change 77 (1–2):85–96. doi:10.1016/j.gloplacha.2011.03.004.
- Sformo, T. L., J. C. Adams, J. A. Seigle, M. K. Ferguson, M. K. Purcell, R. Stimmelmayr, J. H. Welch, L. M. Ellis, J. C. Leppi, and J. C. George. 2017. Observations and first reports of saprolegniosis in Aanaaktiq, broad whitefish (Coregonus nasus), from the Colville River near Nuiqsut, Alaska. Alaska. Polar Science 14:78–82. doi:10.1016/j.polar.2017.07.002.
- Sibley, P. K., D. M. White, P. A. Cott, and M. R. Lilly. 2008. Introduction to water use from Arctic lakes: Identification, impacts, and decision support. Journal of the American Water Resources Association 44 (2):273–75. doi:10.1111/j.1752-1688.2007.00159.x.
- Smejkalova, T., M. E. Edwards, and J. Dash. 2017. Arctic lakes show strong decadal trend in earlier spring ice-out. Scientific Reports 1–8. doi:10.1038/srep38449.
- Stone, R. S., E. G. Dutton, J. M. Harris, and D. Longenecker. 2002. Earlier spring snowmelt in northern Alaska as an indicator of climate change. Journal of Geophysical Research 107 (D10):1–15. doi:10.1029/2000JD000286.
- Streever, B. 2002. Science and emotion, on ice: The role of science on Alaska’s North Slope. Bioscience 52 (2):179–84. doi:10.1641/0006-3568(2002)052[0179:SAEOIT]2.0.CO;2.
- Streever, B., R. Suydam, J. F. Payne, R. Shuchman, R. P. Angliss, G. Balogh, J. Brown, J. Grunblatt, S. Guyer, D. L. Kane, et al. 2011. Environmental change and potential impacts: Applied research priorities for Alaska’s North Slope. Arctic 64 (3):390–97. doi:10.14430/arctic4137.
- Stuefer, S. L., C. D. Arp, D. L. Kane, and A. K. Liljedahl. 2017. Recent extreme runoff observations from coastal arctic watersheds in Alaska. Water Resources Research 53:9145–9163. doi:10.1002/2017WR020567.
- Sturm, M., C. Racine, and K. Tape. 2001. Increasing shrub abundance in the Arctic. Nature 411:546–47. doi:10.1038/35079180.
- Surdu, C. M., C. R. Duguay, L. C. Brown, and D. Prieto. 2014. Response of ice cover on shallow lakes of the North Slope of Alaska to contemporary climate conditions (1950–2011): Radar remote-sensing and numerical modeling data analysis. Cryosphere 8 (1):167–80. doi:10.5194/tc-8-167-2014.
- Toniolo, H., J. Stutzke, A. Lai, E. Youcha, T. Tschetter, D. Vas, J. Keech, and K. Irving. 2017. Antecedent conditions and damage caused by 2015 spring flooding on the Sagavanirktok River, Alaska. Journal of Cold Regions Engineering 31 (2):05017001. doi:10.1061/(ASCE)CR.1943-5495.0000127.
- United States Department of the Interior (USDOI), Bureau of Land Management (BLM). 2014. Final supplemental environmental impact statement for the alpine satellite development plan for the proposed greater mooses tooth one development project. DOI‐BLM‐AK‐0000‐2013‐0001‐EIS, USDOI, BLM, Anchorage, Alaska.
- U.S. Geological Survey. 2014. DOI/GTN-P climate and active-layer data acquired in the National Petroleum Reserve–Alaska and the Arctic National Wildlife Refuge, 1998–2013.
- Walker, D. A., P. J. Webber, E. F. Binnian, K. R. Everett, N. D. Lederer, E. A. Nordstrand, and M. D. Walker. 1987. Cumulative impacts of oil fields on Northern Alaskan landscapes. Science 238 (4828):757–61. doi:10.1126/science.238.4828.757.
- Wendler, G., B. Moore, and K. Galloway. 2014. Strong temperature increase and shrinking sea ice in Arctic Alaska. The Open Atmospheric Science Journal 8:7–15. doi:10.2174/1874282301408010007.
- White, D. M., P. Prokein, M. K. Chambers, M. R. Lilly, and H. Toniolo. 2008. Use of synthetic aperture radar for selecting Alaskan lakes for winter water use. Journal of the American Water Resources Association 44 (2):276–84. doi:10.1111/j.1752-1688.2007.00160.x.
- Whitman, M. S., C. D. Arp, B. M. Jones, W. Morris, G. Grosse, F. Urban, and R. Kemnitz. 2011. Developing a long-term aquatic monitoring network in a complex watershed of the Alaskan Arctic Coastal Plain. In Medley, C. N., Patterson, G., and Parker, M. J. (eds.), Proceedings of the fourth interagency conference on research in watersheds: Observing, studying, and managing for change. Scientific Investigations Report 2011-5169:15-20, United States Geological Survey, Reston.
- Wilby, R. L., H. Orr, G. Watts, R. W. Battarbee, P. M. Berry, R. Chadd, S. J. Dugdale, M. J. Dunbar, J. A. Elliott, C. Extence, et al. 2010. Evidence needed to manage freshwater ecosystems in a changing climate: Turning adaptation principles into practice. Science of the Total Environment 408 (19):4150–64. doi:10.1016/j.scitotenv.2010.05.014.
- Wrona, F. J., M. Johansson, J. M. Culp, A. Jenkins, J. Mård, I. H. Myers-Smith, T. D. Prowse, W. F. Vincent, and P. A. Wookey. 2016. Transitions in Arctic ecosystems: Ecological implications of a changing hydrological regime. Journal of Geophysical Research: Biogeosciences 121 (3):650–74.