ABSTRACT
The coastal regions of the McMurdo Dry Valleys, Antarctica, contain deposits of the Ross Sea Drift, sedimentary material left from the Ross Sea ice sheet from the advance of the West Antarctic ice sheet during the Last Glacial Maximum. Much of this deposit is ice-cored, but data on the stable isotopic composition of water from this ice, which may contain a valuable climate archive, are sparse or incomplete. Widespread thermokarstic ground subsidence in this “coastal thaw zone” of the McMurdo Dry Valleys suggests that these potential records are rapidly being lost due to the melting of ground ice and permafrost. We collected samples of massive buried ice from the Ross Sea Drift in eastern Taylor Valley for δ18O-H2O and δ2H-H2O and measured a broad range of values (δ18O = −27.7 to −37.3 ‰; δ2H = −210 to −295 ‰). These buried ice deposits do not show evidence of alteration through sublimation or evaporation, plot along the local meteoric water line, and have values that indicate ice deposition under a colder climate than present conditions. We propose that this ice was sourced from the Ross Sea ice sheet during the Last Glacial Maximum and contains a valuable and accessible climate record.
Introduction
The McMurdo Dry Valleys (MDV) are the largest ice-free area in Antarctica (; Levy et al. Citation2013), meaning that unlike most of the continent, the ice sheet does not cover the region. Instead, alpine glaciers descend from the mountains into the valley bottoms, and glaciers flowing from the East Antarctic ice sheet (EAIS) block several valley outlets. However, subsurface ice is ubiquitous in this “ice-free” region. Preservation of this ice is possible due to cold mean annual temperatures, nominally −20°C, and the insulating properties of the sandy-gravelly tills that blanket the valley floors (Bockheim, Campbell, and McLeod Citation2007; Levy and Schmidt Citation2016; Obryk et al. Citation2020). A review of 850 shallow (<1.5 m) excavations to examine permafrost in the MDV region found that 55 percent were ice cemented, 43 percent were dry frozen, and 2 percent contained buried glacial ice (Bockheim, Campbell, and McLeod Citation2007). Further north along the Victoria Land coast in the Northern Foothills, ice-free areas are underlain by continuous permafrost and sometimes buried glacier ice under a shallow layer of till (Guglielmin and French Citation2004).
Figure 1. McMurdo Dry Valleys: Landsat Image Mosaic of Antarctica (LIMA) imagery (overview map) and Worldview 2 imagery (inset). Sample locations are displayed on the inset. The dashed green line is a ground-penetrating radar survey where no massive ice was detected, and red represents areas where buried ice was detected. Shaded orange regions on the overview map and the orange outline on inset represent inferred extent of ice-cored Ross Sea Drift (RSD; both data sets used with permission from Levy et al. Citation2018).
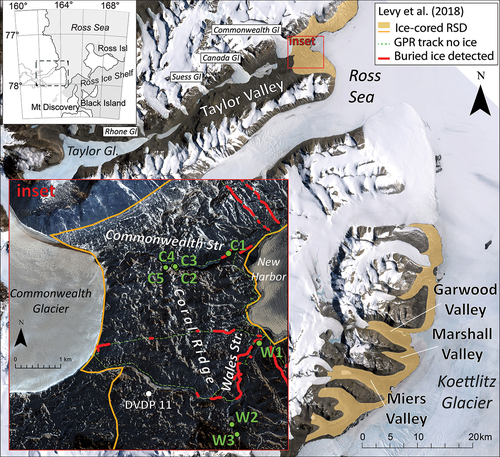
Subsurface ice exists in many forms in the different regions of the MDV, including as extensive ice-cemented soils/permafrost (Dickinson and Rosen Citation2003; Bockheim, Campbell, and McLeod Citation2007; Hagedorn, Sletten, and Hallet Citation2007; Lapalme et al. Citation2017), thin horizontal bands of excess ice (i.e., subsurface ice that exceeds sediment pore volume; Swanger et al. Citation2010; Levy et al. Citation2011; Lacelle et al. Citation2013), buried snowpack (Heldmann et al. Citation2012), and ice generated from surface melt and subsurface refreezing (Harris et al. Citation2007; Levy et al. Citation2011). In addition, massive buried ice deposits have been identified throughout the MDV. These ice deposits are thick (greater than 1 m) and laterally extensive (tens of meters or more) with little or no sediment. The origin and age of these deposits are variable. Massive buried ice deposits in the colder, upland, and hyper-arid portions of the MDVs have been identified as glacial remnants (both ice sheet lobes and alpine glaciers) ranging in age from Miocene to Pleistocene (Sugden et al. Citation1995; Marchant et al. Citation2002; Swanger et al. Citation2010; Lacelle et al. Citation2011; Swanger Citation2017). Warmer coastal regions contain rapidly melting ice-cored moraine previously attributed to remnants of the West Antarctic ice sheet (WAIS) from the Last Glacial Maximum (LGM; Stuiver, Denton et al. Citation1981; Hall, Denton, and Hendy Citation2000; Levy et al. Citation2013). However, this interpretation is based wholly on morphological evidence and the location of ice deposits relative to radiocarbon dates of material within related moraines. It is not known whether these deposits represent ice sheet remnants from the LGM, frozen lakes and ponds formed during ice sheet recession, or other processes. In this article, we interpret the stable isotopic signature of coastal massive buried ice in relation to the LGM.
Péwé (Citation1960) was one of the first to observe massive buried glacial ice in the MDVs in the form of ice-cored moraines, which are common to the coastal edge of the valleys that open to McMurdo Sound. Péwé (Citation1960) had speculated that in the southernmost valleys, this ice originated from the Koettlitz Glacier (). However, recent work found that the Koettlitz Glacier actually retreated during the LGM (Walther Citation2022), supporting the idea that the expansion of the Ross Sea ice sheet filled the mouths of the valleys. Extensive buried ice has been documented in both central and coastal Garwood Valley (Pollard, Doran, and Wharton Citation2002; Levy et al. Citation2013). Stable isotope measurements of oxygen (δ18O) and hydrogen (δ2H) in the buried ice from these latter two studies yielded variable signatures, complicating the interpretation its origins.
Work on glacial deposits along with newer lidar measurements and ground-penetrating radar suggest that massive buried ice is common (), not only in eastern Taylor Valley (TV) but all along the MDV coastal zone (Stuiver, Denton et al. Citation1981; Denton and Marchant Citation2000; Levy et al. Citation2018). The early interpretations made by Péwé (Citation1960) implied that a previous alpine glacier advance in TV could have also deposited ice from the alpine Commonwealth Glacier in the New Harbor region of eastern TV. Hall, Denton, and Hendy (Citation2000) later documented massive ice deposits in this portion of TV and described them as possible remnants of the WAIS from the LGM. Though the authors analyzed eastern TV buried ice deposits for δ18O, no measurements of δ2H were made, so comparisons to meteoric water that could provide information on postdepositional alteration or identification of evaporated proglacial meltwater ponds or lakes as a source were not possible.
Glaciers along the western Ross Sea coastline and alpine glaciers within the MDVs have been stable or at equilibrium over the past half century (Fountain, Glenn, and Scambos Citation2017). However, long-term observations and detailed lidar comparisons (Levy et al. Citation2018) indicate that rapid surface elevation change is occurring in the coastal and lowland regions of the MDVs. Increasing temperatures and humidity have led to greater streamflow and thawing, which results in the wetting of formerly dry and insulating soils, and buried ice melt and thermokarst development (Levy et al. Citation2013, Citation2018; Fountain et al. Citation2014).
The origin and fate of coastal buried ice are vital to understanding past and future climate in this region of Antarctica. These climate archives could potentially be lost or altered in the future due to current and anticipated temperature increases. Investigations on the formation and preservation of these buried deposits have brought about disagreements on the past climatic history of the continent and the utility and interpretation of these ice features as climatic proxies. We present measurements of δ18O and δ2H of water from massive buried ice collected in eastern coastal TV. To the extent of our knowledge, we are the first to report δ2H of massive buried ice in eastern TV. We present a review of existing isotopic data on massive buried ice in the coastal thaw zone of the MDV and discuss its probable source and utility as a climate record. Finally, we discuss the potential fate of this ice and the implications of ice loss and geomorphic modification of the region.
Background: Regional glacial history through the last glacial maximum
Grounded ice from the Ross Sea ice sheet encroached into seaward-facing valleys in the MDV region during the LGM, achieving a maximum thickness and extent 18.7 to 12.8 ka (Denton and Marchant Citation2000; Hall et al. Citation2013, Citation2015). During this time, alpine glaciers terminating in MDV were less extensive (Denton et al. Citation1989; Stuiver, Denton et al. Citation1981). The Ross Sea ice sheet blocked valley openings, creating ice-dammed proglacial lakes, notably Lake Washburn in TV, which reached up to 330 m.a.s.l. elevation (e.g., Stuiver, Yang et al. Citation1981; Hall and Denton Citation2000; Toner, Sletten, and Prentice Citation2013). A moraine called Coral Ridge (), mapped as the Ross Sea Drift (RSD), blocks the coastal opening of TV and marks the last long-duration stand of the ice dam formed by the Ross Sea ice sheet (Stuiver, Denton et al. Citation1981; Hall, Denton, and Hendy Citation2000). Perennially ice-covered proglacial Lake Washburn transported material farther westward via a floating lake ice conveyor system, which deposited RSD material as far west as the present location of Lake Hoare (Stuiver, Denton et al. Citation1981; Hall, Denton, and Hendy Citation2000). Regional ice flow models of the LGM suggest that ice in eastern TV was likely sourced locally from Ross island and adjacent Ferrar Glacier, an outlet of the EAIS (Hall et al. Citation2015). Ross Island likely blocked additional ice from the EAIS from flowing into TV, but because TV is at this boundary, it is also possible that the valley received input from the EAIS and outlet glaciers such as Skelton and Mulock Glaciers. The Ross Sea ice sheet began to recede from the MDV around 12.8 ka (Hall et al. Citation2015), and marine shells at the mouth of TV suggest that deglaciation was complete by about 5400 14C yr BP (Hall and Denton Citation2000).
As a result, the surficial geology of TV to the east of Canada Glacier and below the high stand of Lake Washburn (330 m.a.s.l.) is dominated RSD deposits, many of which contain buried ice (Stuiver, Denton et al. Citation1981; Hall, Denton, and Hendy Citation2000; Levy et al. Citation2018). Denton and Marchant (Citation2000) mapped the extent of the RSD southward along the coastal MDVs to Koettlitz Glacier and identified ice-cored deposits in the openings of Miers, Marshall, and Garwood Valleys as having formed by grounded ice lobes and the lake ice conveyor system previously described. Hall, Denton, and Hendy (Citation2000) and Hall and Denton (Citation2000) sampled buried ice in the New Harbor region of TV and suggested that it was left over from the encroachment of the Ross Sea ice sheet, based on its location within the RSD. Levy et al. (Citation2018) show that buried ice is common in the RSD and New Harbor area of eastern TV using a combination of repeat lidar measurements and ground penetrating radar, the extent of which is displayed in .
Hall, Denton, and Hendy (Citation2000) described two separate moraines in eastern TV within the RSD, from the northern and southern walls of Taylor Valley, which combine to form the Coral Ridge moraine that separates the Lake Fryxell basin to the west from coast. The largest outcrops of massive buried ice along the Commonwealth Stream, which cuts through the ridge, are at the ridge crest or very near it. All of the buried ice samples collected for this study are from the RSD and were likely covered by the grounded Ross Sea ice sheet during the LGM.
Field sampling and analytical methods
Eastern TV, like much of the MDVs, is a polar desert landscape of soils, ice-covered lakes, seasonally flowing streams and alpine glaciers (Fountain et al. Citation1999). The mean annual temperature is −19.2°C at the Explorers Cove weather station (−77.588733, 163.417523, 25 m.a.s.l.) and precipitation is approximately 50 mm water-equivalent per year (Doran et al. Citation2002; Marchant and Head Citation2007; Fountain et al. Citation2010; Obryk et al. Citation2020). During the summer, soil surface temperatures can rise above 0°C. Climatically, this part of the valley resides in the “coastal thaw zone,” which contrasts with the hyper-arid, very cold, and “stable upland” zones at higher elevations (Marchant and Head Citation2007). Much of the coastal thaw zone contains ice-cored moraine remnants of the RSD (Stuiver, Denton et al. Citation1981; ).
Buried ice samples were collected in eastern TV from the incised channels of Wales and Commonwealth streams () during the 2019–2020 austral summer season. Wales Stream flows from the Wales Glacier in the Kukri Hills that border the south side of TV. The stream channel extends approximately 5 km northward to McMurdo Sound at New Harbor. Commonwealth Stream flows from the Commonwealth Glacier in the Asgard Range on the north side of the valley. The channel extends approximately 3.8 km eastward to McMurdo Sound. Both stream channels are incised (15–30 m deep in Commonwealth and ~15 m deep in Wales) through portions of their length, with outcropping buried ice a few meters thick accessible along the banks ().
Figure 2. (a) Wales Stream sample site, facing southeast. White arrow indicates buried ice, covered in till, actively being undercut by Wales Stream above the delta where sample W1 was collected. New Harbor camp is out of the frame to the left. (b) Commonwealth Stream sample site facing northwest toward the accumulation zone of Commonwealth Glacier. White arrow indicates east–west trending horizontally continuous layer of buried ice near Commonwealth Stream along Coral Ridge from which samples C3 bottom, middle, and top were collected.
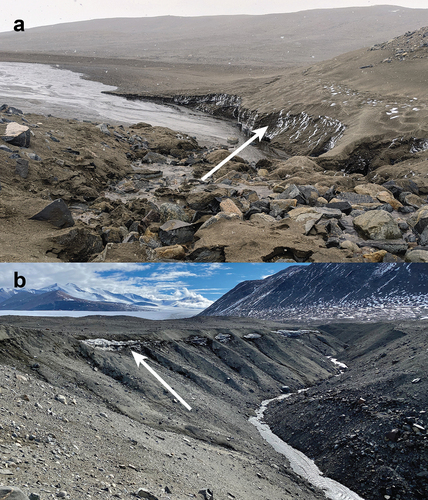
The easternmost accessible buried ice in eastern TV occurs directly upstream from the deltas of both streams (samples C1 and W1) at approximately 6 to 13 m.a.s.l. where they are actively cutting through/under ice layers (). At Wales Stream, the visible ice extends several tens of meters laterally and is approximately 2 m thick and covered with approximately 1 m of RSD sediment. At Commonwealth Stream, this ice was loosely consolidated and granular (sample C1) and covered almost completely by 2 to 3 m of till that had to be removed before sampling. Ice was also collected upstream within the stream channels beginning at approximately 64 m.a.s.l. in Commonwealth Stream and 90 m.a.s.l. in Wales Stream (samples C2 and W2) and occurred as horizontally continuous massive clear buried ice. Two samples were collected upstream along the Wales Stream channel, where ice outcrops were tens of meters wide and 1 to 2 m thick, covered by 2 to 3 m of till along the western bank of the stream. Seven were collected along the Commonwealth Stream channel. Samples were collected on the southern side of the stream channel where ice outcrops were several meters thick and laterally continuous southeast–northwest for 250 to 300 m (), covered by 3 to 4 m of sediment. The north–south extent is not known, but ground-penetrating radar surveys on the north side of the stream did not detect massive buried ice (Levy et al. Citation2018). At sample site C3, three samples were collected in a vertical section, each approximately 1 m apart (labeled top, middle, bottom). At sample site C5, two samples were collected several meters apart laterally (labeled C5A and C5B). The low-elevation deposits above the deltas of both streams are vertically separated from the upstream buried ice by 80 m on the south side (Wales Stream channel) and 50 m of elevation on the north side of the valley (Commonwealth Stream channel).
Samples were collected along the slope of the stream channel by digging through overlying sediment if necessary and removing the outermost layer of ice with an ice ax until clear clean massive ice was reached. Samples were collected in plastic Whirl-pak bags and then melted and filtered in the lab at New Harbor Camp in TV into scintillation vials with zero headspace using plastic syringes and 0.45-μm polypropylene syringe filters prerinsed with sample water.
Samples were analyzed for δ18O-H2O and δ2H-H2O on a Picarro Wavelength Scanned-Cavity Ring Down Spectroscopy Analyzer Model L2130-i at The Ohio State University. Each sample was pipetted into a 2-mL vial, and 2 μL was extracted per injection to determine the δ18O and δ2H of the sample. A total of seven injections were made per sample. The first three were discarded to avoid a memory effect, and the last four injection values were averaged to determine the sample δ18O and δ2H. Internal laboratory standards were analyzed every five samples to correct the raw data. Rotating internal laboratory standards had been calibrated to Vienna Standard Mean Ocean Water at the Institute of Arctic and Alpine Research at the University of Colorado at Boulder by a dual-inlet mass spectrometer and were run after every fifth sample. Our internal laboratory standards were as follows: Colorado (δ18O = −16.53 ‰; δ2H = −126.3 ‰), Nevada (δ18O = −14.20 ‰; δ2H = ‑104.80 ‰), Ohio (δ18O = −8.99 ‰; δ2H = −61.80 ‰), and Florida (δ18O = −2.09 ‰; δ2H = −9.69 ‰). Instrument precision was <0.025 ‰ for δ18O and <0.25 ‰ for δ2H. The in-run accuracy was 0.05 and 0.58 ‰ for δ18O and δ2H, respectively.
Results
The buried ice δ18O values (−27.7 to −37.3 ‰) and δ2H values (−210 to −295 ‰) measured in eastern TV () exhibit a large variation, spanning the range previously noted for the alpine glacier ice in TV, the modern Ross Ice Shelf, the modern EAIS adjacent to the Transantarctic Mountains (TAM), and ice-cemented permafrost near Commonwealth Glacier (). Most of our buried ice values fall along the local meteoric water line (LMWL) determined from Taylor Valley ice (Gooseff et al. Citation2006; ) and do not show evidence of fractionation via sublimation. The highest and lowest elevation samples from the Commonwealth stream channel, which are the most enriched of our samples (samples C1 and C5; ), fall slightly above the LMWL, along the global meteoric water line. Sample C1 was collected just above the Commonwealth delta near sea level, and sample C5 was collected at the highest elevations and furthest distance inland near Commonwealth Glacier (; ). Given the fine granular texture of sample C1 noted in the field and its enriched isotopic signature, it is likely buried snowpack instead of buried glacier ice and will not be considered further in the text. In the Wales stream channel, the lowest elevation samples collected near the coast, just above the deltas (W1), is more enriched than the higher elevation ice samples collected upstream. W2 is the most depleted sample of buried ice collected from any low-elevation coastal MDV site, including eastern Taylor Valley (Hall, Denton, and Hendy Citation2000) and Garwood Valley (Pollard, Doran, and Wharton Citation2002; Levy et al. Citation2013), and is in the upper range of values measured in samples from Taylor Glacier (Gooseff et al. Citation2006), Taylor Dome (Grootes et al. Citation2001), and WAIS Divide (WAIS Divide Project Members Citation2013) at the LGM.
Table 1. δ18O and δ2H of water of buried ice in eastern Taylor Valley. The three samples for C3 were collected from the same location, 1 m apart vertically; the two samples for C5 were collected from the same location several meters apart.
Figure 3. Buried ice δ18O and δ2H of water from massive buried ice in the RSD along Commonwealth and Wales streams in eastern TV (this study) and GV. TV ice and snow from previous studies are included for comparison. Commonwealth Glacier (eastern TV alpine glacier) and Taylor Glacier (western TV outlet of the EAIS) surface means and ranges are represented by a symbol and shaded ovals. Fresh (summertime) snow from Canada and Taylor Glaciers range is represented by the unshaded oval (Gooseff et al. Citation2006). Ice-cemented permafrost δ18O is the top 80 m (down to sea level) of the DVDP core 11. WAIS Divide and Taylor Dome ice cores are included, and the LGM period of the WAIS core is highlighted, the time during which the Ross Sea ice sheet was at its maximum extent in Taylor Valley, 18.7 to 12.8 ka (Hall et al. Citation2015). LMWL = local meteoric water line. GMWL = global meteoric water line. TAM = Transantarctic Mountains. Data sources: 1Gooseff et al. (Citation2006); 2Levy et al. (Citation2013); 3Pollard, Doran, and Wharton (Citation2002); 4Grootes and Stuiver (Citation1986); 5Morgan (Citation1982); 6Grootes et al. (Citation2001); 7Stuiver, Yang et al. (Citation1981); 8WAIS Divide Project Team (Citation2013); 9Gow and Epstein (Citation1972).
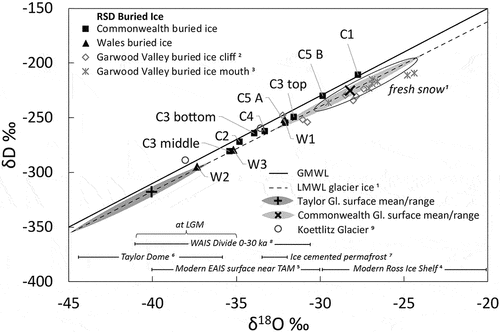
Isotopic values do not show a clear trend with distance inland from the coast and are instead highly variable at low spatial resolution. At sample site C3, three samples were collected in a vertical section, each 1 m apart, and exhibited a range of approximately 4 ‰ in δ18O and 31 ‰ in δ2H. Two samples were also collected at site C5, with a difference of 2.2 ‰ in δ18O and 24 ‰ in δ2H. The variability is similar to that of snow pits from Commonwealth and Howard glaciers (Gooseff et al. Citation2006) and an ice core collected from the Newell Glacier in the Asgard Range north of Taylor Valley (Mayewski et al. Citation1995).
Discussion
Isotopic composition of buried ice in the MDV
The massive buried ice from the eastern TV reported here and by Hall, Denton, and Hendy (Citation2000) as well as in Garwood Valley (GV; Pollard, Doran, and Wharton Citation2002; Levy et al. Citation2013) can be differentiated from the multiple sources of buried ice elsewhere in the MDVs. Coastal ice associated with the RSD generally plots along the LMWL with δ18O ranging from −24 to −37 ‰ and δ2H ranging from −242 to −292 ‰ (). Other buried ice deposits from the MDV region reported in the literature are significantly more depleted and associated with colder higher elevation alpine glaciers (Swanger et al. Citation2010) or remnants of the Taylor Glacier (Swanger Citation2017). Other subsurface ice deposits plot significantly below the LMWL, such as excess ice deposited through water vapor condensation at Table Mountain (Dickinson and Rosen Citation2003) and University Valley (Lacelle et al. Citation2013).
Hall, Denton, and Hendy (Citation2000) published the only other δ18O values of massive buried ice in eastern TV, though these values are quite enriched compared to our data from comparable locations. They measured a narrow range of values from −28.6 to −30.4 ‰ (mean = −29.6 ‰, n = 7) in an abandoned tributary of Wales Stream, which are more enriched than the ice we sampled along the Wales stream channel (−35.2 to −37.3 ‰) and comparable to the δ18O of snow the region currently receives. They also measured much more enriched δ18O values on the northeast side of Commonwealth Glacier (−12.0 ‰, n = 3) and at the Taylor Valley mouth threshold (−7.2 ‰). They posited that the more enriched values were potentially due to postdepositional fractionation via sublimation (Hall, Denton, and Hendy Citation2000), though no δ2H data or sample locations are available. We did not sample any buried ice with such enriched δ18O values; therefore, we cannot confirm this hypothesis, but none of our buried ice samples exhibit a deviation from the LMWL that is consistent with fractionation from sublimation (). As stated previously, we discarded surface samples of the exposed ice to avoid possible surface alternations.
Isotopic data are also available from GV from an ice cliff in the mid-valley delta complex (Levy et al. Citation2013) and buried ice covered in till at the valley mouth (Pollard, Doran, and Wharton Citation2002; ). Samples collected inland from the ice cliff (mean: δ18O = −29.4 ‰, δ2H = 239 ‰; Levy et al. Citation2013) and the valley mouth (mean: δ18O = −26.6 ‰, δ2H = −219 ‰; Pollard, Doran, and Wharton Citation2002) are both in the range of TV glacier ice (Gooseff et al. Citation2006) and our more enriched samples collected from the Commonwealth stream channel.
Levy et al. (Citation2013) also noted that the more inland ice from the mid-valley ice cliff is significantly more depleted than the coastal ice collected approximately 5 km down-valley by Pollard, Doran, and Wharton (Citation2002). This general trend is also apparent in TV, where the buried ice collected above the delta from Wales Stream is more enriched than ice at higher elevation further inland (, ). Levy et al. (Citation2013) attributed this difference to the complex mixture of marine- and glacially derived ice of the modern Ross Ice Shelf described by Kellogg, Kellogg, and Stuiver (Citation1990) or intermixing of buried ice with refrozen stream water.
The only other isotopic data available for subsurface ice in eastern TV are from ice-cemented permafrost. The Dry Valleys Drilling Project (DVDP) did not report buried massive ice in core 11, 3 km west of New Harbor at 80.2 m.a.s.l. (McKelvey Citation1981; Stuiver, Yang et al. Citation1981). This region is covered by the RSD but farther west than the valley threshold at Coral Ridge, marking the furthest advance of the Ross Sea ice sheet. In DVDP core 11, ice-cemented permafrost was encountered and the δ18O of the top 80 m ranged from −32 to −33 ‰ (Stuiver, Yang et al. Citation1981), similar to the values from massive buried ice near Commonwealth Stream. Below 80 m (i.e., below sea level) the δ18O of permafrost becomes gradually more enriched to around −28 ‰ at 200 m (Stuiver, Yang et al. Citation1981). The authors presented two hypotheses of its source: first, that the permafrost was formed by connate waters at the time of sediment deposition and that the gradual trend of δ18O enrichment with depth to 200 m may reflect a cooling climate in Taylor Valley up to the time of sampling (1974). Alternatively, permafrost could have formed from the top if earlier permafrost melting occurred when the site was covered by glacial Lake Washburn, which subsequently contributed to permafrost formation (Stuiver, Yang et al. Citation1981). Recent airborne resistivity surveys concluded that paleolakes remained elevated relative to current levels after ice sheet retreat up to 81 m.a.s.l. as recently as 1.5 ka, followed by slow permafrost growth from the top down (Myers et al. Citation2021).
Isotopic composition of glacier ice and snow in Taylor Valley and regional ice cores
The δ18O of buried ice collected in eastern TV spans the range of the alpine glaciers throughout the valley (). The isotopic compositions of alpine glaciers trend from most enriched in the warmer coastal climate of eastern TV east near the buried ice samples (Commonwealth Glacier mean δ18O = −28.2 ‰) to intermediate values mid-valley (Suess Glacier mean δ18O = −31.4 ‰) to most depleted in the colder higher elevation western portion of the valley (Rhone Glacier mean δ18O = −36.6 ‰; Gooseff et al. Citation2006). The most depleted glacier values in TV are measured at Taylor Glacier (mean δ18O = −40.4 ‰; Gooseff et al. Citation2006), which has an accumulation zone at Taylor Dome approximately 80 km west on the EAIS. Commonwealth Glacier δ18O values are also close to the Holocene snow and ice values (~−30 ‰) collected at a higher elevation on Newall Glacier in the Asgard Range immediately north of Canada Glacier (Mayewski et al. Citation1995). Modern fresh snow from Canada and Taylor glaciers clusters in a relatively narrow range (δ18O = −24 to −30 ‰, δ2H = −200 to −250 ‰) and is significantly more enriched than eastern TV buried ice, whereas snow pit data from above the equilibrium lines of Commonwealth and Howard glaciers exhibited a very wide range (δ18O = −20 to −40 ‰, δ2H = −155 to −330 ‰; Gooseff et al. Citation2006).
The most enriched δ18O values in buried ice from the Commonwealth stream channel fall in the range of more depleted surface ice from the modern Ross Ice shelf (−20 to −30 ‰; Grootes and Stuiver Citation1986), whereas the majority are in the range of modern EAIS inland from the Ross Ice Shelf, on the other side of the Transantarctic Mountains (−30 to −40 ‰; Morgan Citation1982; ). Ice at Taylor Dome corresponding to the period of the grounded Ross Sea Ice Sheet at its LGM configuration (18.7–12.8 ka) records a period of warming (and potentially low accumulation) when δ18O increased from −44 to −36 ‰ (Grootes et al. Citation2001) and overlaps with the WAIS Divide record at the same time of −41 to −35 ‰ (WAIS Divide Project Members Citation2013) as well as the most depleted buried ice measured at the highest elevation along with Wales stream channel.
Sources and interpretation
Though there is substantial variation in the isotopic composition of buried ice in eastern TV and it cannot be directly dated to the LGM, several lines of evidence suggest that it is likely glacial ice from the Ross Sea ice sheet incursion. The presence of massive buried in the RSD drift alone is suggestive of LGM ice, as hypothesized by Hall, Denton, and Hendy (Citation2000). Buried ice in the Coral Ridge moraine at Commonwealth Stream and along the Wales stream channel in the current study are substantially more depleted than the ice measured by Hall, Denton, and Hendy (Citation2000), and our addition of δ2H data places the ice along the LMWL, which is not indicative of an evaporative signal that would suggest a previously open water source, such as a lake or pond. The isotopic signature of the buried ice we sampled is more depleted than modern snow in TV, suggestive of a colder/higher source of precipitation than the region currently receives (). This source is more depleted than the modern Ross Ice Shelf, and the most depleted samples from the Wales stream channel overlap the range of ice collected from WAIS Divide dated to the LGM (WAIS Divide Project Members Citation2013). The δ18O values of buried ice in eastern TV are similar to ice-cemented permafrost from DVDP core 11 near Commonwealth Glacier, which has been recently interpreted as sourced from water infiltration from proglacial Lake Washburn (Myers et al. Citation2021). This possibility is indicative of the isotopic composition of meteoric water at the time. Buried ice from the TV RSD is more depleted and less altered than ice from GV (Pollard, Doran, and Wharton Citation2002; Levy et al. Citation2013), indicating a potential difference in precipitation source and/or ice flow lines between the two valleys at the time of deposition.
Ice flow lines in the McMurdo Sound region reconstructed from geologic data, including kenyte erratics only found on Ross Island (Hall et al. Citation2015), trace Ross Sea ice sheet flow during the last glacial advance from Ross Island into both Garwood and Taylor Valleys. Given the similar ice source between the two valleys, the considerable difference in the isotopic compositions of ice deposits in the two valleys may represent a climate signal from different times during the last glaciation or different mixtures of locally sourced and distally sourced ice. For example, buried ice in Garwood Valley might reflect a mixture of proximally sourced ice and additional inputs from Ross Island distally sourced from the EAIS interior. In contrast, the comparatively more depleted ice deposited in TV may represent nearly entirely unaltered and minimally mixed ice from the Ross Sea ice sheet with smaller inputs from glacial flow off Ross Island.
Fate of the coastal buried ice in Taylor Valley
Lidar measurements have shown extensive thermokarst driven subsidence and erosion in the MDVs between the 2001–2002 and 2014–2015 austral summers (Levy et al. Citation2018). The largest gross volume losses were in regions with massive buried ice associated with RSD deposits, especially in the coastal thaw zones <300 m.a.s.l. Both Commonwealth and Wales streams are in the coastal thaw zone and have experienced gross volume losses of approximately 1 × 105 and 0.6 × 105 m3 over a thirteen-year period, respectively, and were incised over this period over reaches hundreds of meters long due to the erosion of massive buried ice and bank collapse (Levy et al. Citation2018). These changes are also significant to the biogeochemistry of the region, because erosion of permafrost in the MDV has also been shown to increase fine suspended loads and solute flux in impacted streams, affecting nutrient loads to closed basin lakes (Gooseff et al. Citation2016) and, in the case of Commonwealth and Wales streams, to McMurdo Sound (Olund et al. Citation2018).
It has been suggested that as the climate continues to warm these landscape features in the MDV’s coastal and valley bottom regions will be at risk of ice melt and thermokarst subsidence. These changes could then lead to the development of new landscapes similar to what is currently observed in arctic periglacial areas (Levy et al. Citation2013; Fountain et al. Citation2014). Because changes in polar desert ecosystems are driven by the timing of ice melt and the amount of water generated during the austral summer, these landscape changes will not only generate geomorphological changes but also modify biogeochemical fluxes and alter the structure and extent of these ecosystems (Fountain et al. Citation1999; Barrett et al. Citation2008). The melting of buried ice in TV will also threaten this potential climatic archive and inhibit our ability to understand past and future ice movement.
Additional climate signals may be encapsulated in ice deposits along valley openings in the MDV. For example, the highest elevation ice and sediment cover preserved in eastern TV may represent peak ice thickness conditions of the Ross Sea ice sheet 18.7 to 12.8 ka. We hypothesize that higher elevation ice is the most depleted, falling in the range of the WAIS core during this time (WAIS Divide Project Members Citation2013), with progressive enrichment toward lower elevations, representing ice deposited sequentially during deglaciation and ice drainage. Likewise, to the extent that the RSD is a recessional deposit, buried ice in TV to the north may be the oldest, with younger ice deposits to the south in Garwood and Miers Valleys. It is also noteworthy that the ice remnants in the RSD in TV from the LGM were potentially deposited tens of thousands of years earlier near Ross Island before ice sheet movement brought it to its finally resting point in eastern TV. Regardless, ice sheet remnants present as buried ice within the RSD and its northern Victoria land analogs may help constrain the timing of glacial retreat in the MDV.
Conclusions
Massive buried ice was collected from eastern Taylor Valley, Antarctica, and analyzed for δ18O and δ2H of water. The surficial geology of the region is characterized by the Ross Sea Drift, located in the coastal thaw zone of the McMurdo Dry Valleys, deposited during the retreat of the West Antarctic ice sheet approximately 8 ka. The δ18O values (−27.7 to to −37.3 ‰) and δ2H values (−210 to −295 ‰) collected from the Wales and Commonwealth stream channels exhibit a large variation, but their location along the local meteoric water line indicates that the temperatures were colder and precipitation was more depleted than the region currently receives, and evaporation and sublimation have not significantly altered their isotopic compositions. We suggest that this ice is likely a remnant from the advance of the Ross Sea ice sheet during the Last Glacial Maximum before approximately 8 ka. The large range of δ18O and δ2H values across deposits in Taylor and Garwood Valleys suggests that this buried ice represents an unstudied but accessible climate record. Because active erosion in the McMurdo Dry Valleys is most pronounced in areas of thermokarst-driven subsidence due to the melting of buried massive ice such as the deposits measured here, this potential climate record may be lost due to future warming.
Acknowledgments
We are grateful to the USAP, Antarctic Support Contract staff, and Air Center Helicopters, Inc. for field support. We also thank A. Calero for assistance collecting samples.
Disclosure statement
No potential conflict of interest was reported by the authors.
Additional information
Funding
References
- Barrett, J. E., R. A. Virginia, D. H. Wall, P. T. Doran, A. G. Fountain, K. A. Welch, and W. B. Lyons. 2008. Persistent effects of a discrete warming event on a polar desert ecosystem. Global Change Biology 14 (10):2249–61. https://doi.org/10.1111/j.1365-2486.2008.01641.x
- Bockheim, J. G., I. B. Campbell, and M. McLeod. 2007. Permafrost distribution and active-layer depths in the McMurdo Dry Valleys, Antarctica. Permafrost and Periglacial Processes 18:217–27. doi:10.1002/ppp.588.
- Denton, G. H., J. G. Bockheim, S. C. Wilson, and M. Stuiver. 1989. Late Wisconsin and early Holocene glacial history, inner Ross embayment, Antarctica. Quaternary Research 31:151–82. doi:10.1016/0033-5894(89)90004-5.
- Denton, G. H., and D. R. Marchant. 2000. The geologic basis for a reconstruction of a grounded ice sheet in McMurdo Sound, Antarctica, at the last glacial maximum. Geografiska Annaler, Series A: Physical Geography 82:167–211. doi:10.1111/j.0435-3676.2000.00121.x.
- Dickinson, W. W., and M. R. Rosen. 2003. Antarctic permafrost: An analogue for water and diagenetic minerals on Mars. Geology 31:199. doi:10.1130/0091-7613(2003)031<0199:APAAFW>2.0.CO;2.
- Doran, P. T., J. C. Priscu, W. B. Lyons, J. E. Walsh, A. G. Fountain, D. M. McKnight, D. L. Moorhead, et al. 2002. Antarctic climate cooling and terrestrial ecosystem response. Nature 415:517–20. doi:10.1038/nature710.
- Fountain, A. G., W. B. Lyons, M. B. Burkins, G. L. Dana, T. Peter, K. J. Lewis, D. M. Mcknight, et al. 1999. Physical controls on the Taylor Valley ecosystem, Antarctica. BioScience 49:961–71. doi:10.2307/1313730.
- Fountain, A. G., T. H. Nylen, A. Monaghan, H. J. Basagic, and D. Bromwich. 2010. Snow in the McMurdo Dry Valleys, Antarctica. International Journal of Climatology 30:633–42. doi:10.1002/joc.1933.
- Fountain, A. G., J. S. Levy, M. N. Gooseff, and D. Van Horn. 2014. The McMurdo Dry Valleys: A landscape on the threshold of change. Geomorphology 225:25–35. doi:10.1016/j.geomorph.2014.03.044.
- Fountain, A. G., B. Glenn, and T. A. Scambos. 2017. The changing extent of the glaciers along the western Ross Sea, Antarctica. Geology 45:927–30. doi:10.1130/G39240.1.
- Gooseff, M. N., W. B. Lyons, D. M. McKnight, B. H. Vaughn, A. G. Fountain, and C. Dowling. 2006. A stable isotopic investigation of a polar desert hydrologic system, McMurdo Dry Valleys, Antarctica. Arctic, Antarctic, and Alpine Research 38:60–71. doi:10.1657/1523-0430(2006)038[0060:ASIIOA]2.0.CO;2.
- Gooseff, M. N., D. Van Horn, Z. Sudman, D. M. McKnight, K. A. Welch, and W. B. Lyons. 2016. Stream biogeochemical and suspended sediment responses to permafrost degradation in stream banks in Taylor Valley, Antarctica. Biogeosciences 13:1723–32. doi:10.5194/bg-13-1723-2016.
- Gow, A. J., and S. Epstein. 1972. On the use of stable isotopes to trace the origins of ice in a floating ice tongue. Journal of Geophysical Research 77:6552–57. doi:10.1029/JC077i033p06552.
- Grootes, P. M., and M. Stuiver. 1986. Ross Ice Shelf oxygen isotopes and West Antarctic climate history. Quaternary Research 26:49–67. doi:10.1016/0033-5894(86)90083-9.
- Grootes, P. M., E. J. Steig, M. Stuiver, E. D. Waddington, D. L. Morse, and M. J. Nadeau. 2001. The Taylor Dome Antarctic 18O record and globally synchronous changes in climate. Quaternary Research 56:289–98. doi:10.1006/qres.2001.2276.
- Guglielmin, M., and H. M. French. 2004. Ground ice in the Northern Foothills, northern Victoria Land, Antarctica. Annals of Glaciology 39:495–500. doi:10.3189/172756404781814726.
- Hagedorn, B., R. S. Sletten, and B. Hallet. 2007. Sublimation and ice condensation in hyperarid soils: Modeling results using field data from Victoria Valley, Antarctica. Journal of Geophysical Research: Earth Surface 112. doi:10.1029/2006JF000580.
- Hall, B. L., G. H. Denton, and C. H. Hendy. 2000. Evidence from Taylor Valley for a grounded ice sheet in the Ross Sea, Antarctica. Geografiska Annaler, Series A: Physical Geography 82:275–303. doi:10.1111/j.0435-3676.2000.00126.x.
- Hall, B. L., and G. H. Denton. 2000. Radiocarbon chronology of Ross Sea drift, eastern Taylor Valley, Antarctica: Evidence for a grounded ice sheet in the Ross Sea at the last glacial maximum. Geografiska Annaler, Series A: Physical Geography 82:305–36. doi:10.1111/j.0435-3676.2000.00127.x.
- Hall, B. L., G. H. Denton, J. O. Stone, and H. Conway. 2013. History of the grounded ice sheet in the Ross Sea sector of Antarctica during the last glacial maximum and the last termination. Geological Society Special Publication 381:167–81. doi:10.1144/SP381.5.
- Hall, B. L., G. H. Denton, S. L. Heath, M. S. Jackson, and T. N. B. Koffman. 2015. Accumulation and marine forcing of ice dynamics in the western Ross Sea during the last deglaciation. Nature Geoscience 8:625–28. doi:10.1038/ngeo2478.
- Harris, K. J., A. E. Carey, W. B. Lyons, K. A. Welch, and A. G. Fountain. 2007. Solute and isotope geochemistry of subsurface ice melt seeps in Taylor Valley, Antarctica. Geological Society of America Bulletin 119:548–55. doi:10.1130/B25913.1.
- Heldmann, J. L., M. Marinova, K. E. Williams, D. Lacelle, C. P. McKay, A. Davila, W. Pollard, and D. T. Andersen. 2012. Formation and evolution of buried snowpack deposits in Pearse Valley, Antarctica, and implications for Mars. Antarctic Science 24:299–316. doi:10.1017/S0954102011000903.
- Kellogg, T. B., D. F. Kellogg, M. Stuiver. 1990. Late Quaternary history of the southwestern Ross Sea: Evidence from debris bands on the McMurdo Ice Shelf, Antarctica. In Contributions to Antarctic Research 1, and C. R. Bentley, 73–76. Washington, DC: American Geophysical Union.
- Lacelle, D., A. F. Davila, W. H. Pollard, D. Andersen, J. Heldmann, M. Marinova, and C. P. McKay. 2011. Stability of massive ground ice bodies in University Valley, McMurdo Dry Valleys of Antarctica: Using stable O-H isotope as tracers of sublimation in hyper-arid regions. Earth and Planetary Science Letters 301:403–11. doi:10.1016/j.epsl.2010.11.028.
- Lacelle, D., A. F. Davila, D. Fisher, W. H. Pollard, R. DeWitt, J. Heldmann, M. M. Marinova, and C. P. McKay. 2013. Excess ground ice of condensation-diffusion origin in University Valley, Dry Valleys of Antarctica: Evidence from isotope geochemistry and numerical modeling. Geochimica et Cosmochimica Acta 120:280–97. doi:10.1016/j.gca.2013.06.032.
- Lapalme, C., D. Lacelle, W. Pollard, D. Fisher, A. Davila, and C. P. McKay. 2017. Distribution and origin of ground ice in University Valley, McMurdo Dry Valleys, Antarctica. Antarctic Science 29:183–98. doi:10.1017/S0954102016000572.
- Levy, J. S., A. G. Fountain, M. N. Gooseff, K. A. Welch, and W. B. Lyons. 2011. Water tracks and permafrost in Taylor Valley, Antarctica: Extensive and shallow groundwater connectivity in a cold desert ecosystem. Geological Society of America Bulletin 123:2295–311. doi:10.1130/B30436.1.
- Levy, J. S., A. G. Fountain, J. E. O’Connor, K. A. Welch, and W. B. Lyons. 2013. Garwood Valley, Antarctica: A new record of Last Glacial Maximum to Holocene glaciofluvial processes in the McMurdo Dry Valleys. Bulletin of the Geological Society of America 125:1484–502. doi:10.1130/B30783.1.
- Levy, J. S., and L. M. Schmidt. 2016. Thermal properties of Antarctic soils: Wetting controls subsurface thermal state. Antarctic Science 28:361–70. doi:10.1017/S0954102016000201.
- Levy, J. S., A. G. Fountain, M. K. Obryk, J. Telling, C. Glennie, R. Pettersson, M. Gooseff, and D. J. Van Horn. 2018. Decadal topographic change in the McMurdo Dry Valleys of Antarctica: Thermokarst subsidence, glacier thinning, and transfer of water storage from the cryosphere to the hydrosphere. Geomorphology 323:80–97. doi:10.1016/j.geomorph.2018.09.012.
- Marchant, D. R., A. R. Lewis, W. M. Phillips, E. J. Moore, R. A. Souchez, G. H. Denton, D. E. Sugden, J. Potter, and G. P. Landis. 2002. Formation of patterned ground and sublimation till over Miocene glacier ice in Beacon Valley, southern Victoria Land, Antarctica. Bulletin of the Geological Society of America 114:718–30. doi:10.1130/0016-7606(2002)114<0718:FOPGAS>2.0.CO;2.
- Marchant, D. R., and J. W. Head. 2007. Antarctic dry valleys: Microclimate zonation, variable geomorphic processes, and implications for assessing climate change on Mars. Icarus 192:187–222. doi:10.1016/j.icarus.2007.06.018.
- Mayewski, P. A., W. B. Lyons, G. Zielinski, M. Twickler, S. Whitlow, J. Dibb, P. Grootes, et al. 1995. An ice-core-based, Late Holocene history for the Transantarctic Mountains, Antarctica. In Contributions to Antarctic Research IV, ed. D. H. Elliot and G. L. Blaisdell, 33–45. Washington, D. C: American Geophysical Union. doi:10.1002/9781118668207.ch4.
- McKelvey, B. C. 1981. The lithologic logs of DVDP cores 10 and 11, eastern Taylor Valley. In Dry Valley drilling project, ed. L. D. McGinnis, 63–94. Washington, D. C: American Geophysical Union.
- Morgan, V. I. 1982. Antarctic ice sheet surface oxygen isotope values. Journal of Glaciology 28:315–23. doi:10.1017/S0022143000011655.
- Myers, K. F., P. T. Doran, S. M. Tulaczyk, N. T. Foley, T. S. Bording, E. Auken, H. A. Dugan, et al. 2021. Thermal legacy of a large paleolake in Taylor Valley, East Antarctica, as evidenced by an airborne electromagnetic survey. The Cryosphere 15:3577–93. doi:10.5194/tc-15-3577-2021.
- Obryk, M. K., P. T. Doran, A. G. Fountain, M. Myers, and C. P. McKay. 2020. Climate from the McMurdo Dry Valleys, Antarctica, 1986–2017: Surface air temperature trends and redefined summer season. Journal of Geophysical Research: Atmospheres 125. doi:10.1029/2019JD032180.
- Olund, S., W. B. Lyons, S. A. Welch, and K. A. Welch. 2018. Fe and nutrients in coastal Antarctic streams: Implications for primary production in the Ross Sea. Journal of Geophysical Research: Biogeosciences 123:3507–22. doi:10.1029/2017JG004352.
- Péwé, T. L. 1960. Multiple glaciation in the McMurdo Sound region, Antarctica: A progress report. The Journal of Geology 68:498–514. doi:10.1086/626684.
- Pollard, W., P. Doran, and R. Wharton. 2002. The nature and significance of massive ground ice in Ross Sea Drift, Garwood Valley, McMurdo Sound. New Zealand: Royal Society of New Zealand.
- Stuiver, M., G. H. Denton, T. J. Hughes, and J. Fastook. 1981a. History of the marine ice sheet in West Antarctica during the last glaciation: A working hypothesis. In The last great ice sheets, ed. G. H. Denton and T. J. Hughes, 319–62. New York, NY: John Wiley & Sons.
- Stuiver, M., I. C. Yang, G. H. Denton, and T. B. Kellogg. 1981b. Oxygen isotope ratios on Antarctic permafrost and glacier ice. American Geophysical Union, Antarctic Research Series 33:131–39. doi:10.1029/ar033p0131.
- Sugden, D. E., D. R. Marchant, N. Potter Jr, R. A. Souchez, G. H. Denton, C. C. Swisher III, and J.-L. Tison. 1995. Preservation of Miocene glacier ice in East Antarctica. Nature 376:412. doi:10.1038/376412a0.
- Swanger, K. M., D. R. Marchant, D. E. Kowalewski, and J. W. Head. 2010. Viscous flow lobes in central Taylor Valley, Antarctica: Origin as remnant buried glacial ice. Geomorphology 120:174–85. doi:10.1016/j.geomorph.2010.03.024.
- Swanger, K. M. 2017. Buried ice in Kennar Valley: A late Pleistocene remnant of Taylor Glacier. Antarctic Science 29:239–51. doi:10.1017/S0954102016000687.
- Toner, J. D., R. S. Sletten, and M. L. Prentice. 2013. Soluble salt accumulations in Taylor Valley, Antarctica: Implications for paleolakes and Ross Sea Ice Sheet dynamics. Journal of Geophysical Research: Earth Surface 118:198–215. doi:10.1029/2012JF002467.
- WAIS Divide Project Members. 2013. Onset of deglacial warming in West Antarctica driven by local orbital forcing. Nature 500:440–44. doi:10.1038/nature12376.
- Walther, T. L. 2020. Reconstruction of Koettlitz Glacier, Southern McMurdo Sound, Antarctica, during the Last Glacial Maximum and Termination. Electronic Theses and Dissertations. https://digitalcommons.library.umaine.edu/etd/3318.