ABSTRACT
Despite the perception that e-cigarettes are safer than conventional cigarettes, numerous findings demonstrated that e-cigarette aerosol (EC) exposure induced compromised immune functionality, vascular changes even after acute exposure, and lung injury. Notably, altered neutrophil functionality and platelet hemodynamics have been observed post-EC exposure. It was hypothesized that EC exposure initiates an inflammatory response resulting in altered neutrophil behavior and increased neutrophil-platelet interaction in the pulmonary microvasculature. Neutrophil and platelet responses were examined up to 48 hrs following whole-body, short-term EC exposure without flavorants or nicotine in a murine model, which most closely modeled secondhand exposure. This study is the first to investigate the impact of EC exposure through lung intravital imaging. Compared to room air-exposed mice, EC-exposed mice displayed significantly increased 1.7‒1.9-fold number of neutrophils in the pulmonary microvasculature associated with no marked change in neutrophils within whole blood or bronchoalveolar lavage fluid (BALF). Neutrophil-platelet interactions were also significantly elevated 1.9‒2.5-fold in exposed mice. Plasma concentration of myeloperoxidase was markedly reduced 1.5-fold 48 hr following exposure cessation, suggesting suppressed neutrophil antimicrobial activity. Cytokine expression exhibited changes indicating vascular damage. Effects persisted for 48 hr post-EC exposure. Data demonstrated that EC exposure repeated for 3 consecutive days in 2.5 hr intervals in the absence of flavorants or nicotine resulted in modified pulmonary vasculature hemodynamics, altered immune functionality, and a pro-inflammatory state in female BALB/cJ mice.
Introduction
Although e-cigarette use is often perceived as a less risky alternative to traditional cigarette smoking, the documented presence of oncogenic, cytotoxic, and volatile organic compounds (VOCs) in e-liquid and e-cigarette aerosols as well as the recent spate of critical illnesses and deaths associated with e-cigarette exposure or vaping product use with consequent lung injury (EVALI) illustrated that such perceptions are flawed (Bracken-Clarke et al. Citation2021; Kim, Kim, and Shin Citation2022; Schick et al. Citation2017; Stefaniak et al. Citation2021). Although current evidence suggests EVALI may be linked to vitamin E acetate contamination of e-liquid and is largely a result of “misuse” of e-cigarettes as delivery devices for tetrahydrocannabinol, this link is not yet definitive. However, given the presence of other toxicants in e-liquid, the question of whether “typical” e-cigarette use generates subclinical pathologies that may facilitate chronic dysfunction remains highly relevant (Bhat et al. Citation2020; Blount et al. Citation2020; Effah et al. Citation2022; Feldman, Stanton, and Suelzer Citation2021).
Answering this question requires dissecting the physiologic effects of different components in e-liquid formulations: 1) the myriad of flavorants, 2) nicotine, which has been well characterized as a toxicant in the context of cigarette smoking, and 3) the most common base e-liquid components, propylene glycol (PG) and vegetable glycerin (VG); the volumetric ratio of VG:PG varies between commercially produced e-liquid formulations (Effah et al. Citation2022). E-cigarette aerosol (EC), typically produced as a result of heating and aerosolizing e-liquids, is known to initiate platelet activation (Hom et al. Citation2016), neutrophil dysregulation (Wang et al. Citation2019), and altered cytokine expression (Gellatly et al. Citation2020). Further, nicotine-free EC was found to increase upper airway resistance (Lechasseur et al. Citation2020), enhance bacterial respiratory colonization (Miyashita et al. Citation2018), and impair cerebrovascular function in the offspring of rats exposed during pregnancy (Olfert et al. Citation2018). These findings illustrate that EC exposure alone mediates functional tissue-level alterations that may contribute to downstream pathology.
Short-term EC exposure models afford the unique opportunity to study early stages of processes that may later spiral into chronic pathologies relevant to long-term e-cigarette users. Further, whether there are pathological consequences to transient exposure events remains poorly defined. Early-stage responses to inhaled irritants are often mediated by inflammatory processes involving the innate immune system (Wang et al. Citation2022). Reactive oxygen species (ROS) are present within EC (Lerner et al. Citation2015) and are also generated endogenously by both the epithelium lining the airways (Muthumalage et al. Citation2019; Wu et al. Citation2014) and vessel endothelium (Chatterjee et al. Citation2019), provoking immune response and pulmonary inflammation on both sides of the blood-air barrier. Platelets and neutrophils are amongst the frontline responders to an inflammatory insult, and have both been implicated in the response to EC exposure (Corriden et al. Citation2020; Hom et al. Citation2016; Nocella et al. Citation2018; Qasim et al. Citation2018; Reidel et al. Citation2018; Wang et al. Citation2019). Specifically, platelets undergo pro-thrombotic changes after vaping in both humans and mice whereby adhesion factors such as P-selectin are increased to promote clotting (Nocella et al. Citation2018; Ramirez et al. Citation2020). Activated platelets also adhere to neutrophils to form neutrophil-platelet aggregates, which were shown to be associated with pathologic outcomes in acute lung injury induced by diverse stimuli (Caudrillier and Looney Citation2012; Ortiz-Muñoz et al. Citation2014; Zarbock, Singbartl, and Ley Citation2006).
Neutrophil-platelet aggregates produce downstream pro-inflammatory functionalities such as release of web-like DNA structures called neutrophil extracellular traps (NETs) (Etulain et al. Citation2015). Originally characterized as an anti-microbial response, NETosis is now considered a hallmark of inflammation in a variety of disease states including cystic fibrosis, sepsis, and cancer (Snoderly, Boone, and Bennewitz Citation2019). Once released, NETs provoke endothelial damage, platelet activation, and additional neutrophil recruitment, thereby forming a positive feedback loop that may result in vessel occlusion (Kim and Jenne Citation2016). However, existing investigations reported conflicting results regarding the influence of EC on NETosis and neutrophil recruitment. Reidel et al. (Citation2018) noted no marked change in neutrophil % in the sputum of e-cigarette users; however, NETosis was elevated in user peripheral neutrophils upon treatment with phorbol 12-myristate 13-acetate (PMA), a NET-inducing stimulus, relative to NETosis induced by PMA in non-user neutrophils. Conversely, Corriden et al. (Citation2020) reported a decrease in the number of neutrophils recovered from the peritoneal space of EC-exposed mice challenged with P. aeruginosa, as well as suppressed PMA-induced NETosis in non-e-cigarette user peripheral neutrophils treated with EC ex vivo compared to neutrophils treated with PMA alone. The impact of EC exposure on neutrophil-platelet aggregation and neutrophil recruitment specifically within the pulmonary microvasculature in vivo as well as persistence of this inflammatory response after cessation of exposure still needs to be defined.
The aim of this study was to assess whether nicotine-free and flavorant-free secondhand EC exposure affects neutrophil and platelet recruitment to the lung, NETosis, and neutrophil-platelet aggregation in the pulmonary vasculature. It was hypothesized that short-term EC exposure provokes inflammation in the pulmonary vessels leading to increased neutrophil and platelet interaction. This study marks the first time that intravital lung imaging was applied to visualize the biological consequences following EC exposure, including neutrophil recruitment, platelet trafficking and neutrophil-platelet aggregation in the pulmonary microvasculature over multiple timepoints. Separate cohorts of mice were imaged to assess the persistence of inflammatory effects ranging from the same day to 48 hr post EC exposure for 3 consecutive days. To further characterize the vascular and lung injury responses, downstream investigations were conducted to assess lung histology, immune cell infiltration, NET markers, ROS, and cytokine expression.
Materials and methods
Mice and EC exposure model
All procedures were approved by the WVU Animal Care and Use Committee. Female BALB/cJ mice were obtained from The Jackson Laboratory. All exposures were conducted between 12 and 16 weeks of age in mice weighing approximately 21 g. Animals were randomly assigned to room air (RA) sham exposure or EC exposure. EC exposure occurred in a whole-body exposure system () over 3 consecutive days. Because nose cones were not utilized to facilitate direct inhalation of EC, this model most closely mimics secondhand exposure. Animals undergoing EC exposure were kept separated by partitions throughout the course of exposure. A Joyetech EXCEED device set to 30W was fitted with a D19 (0.5Ω) atomizer. Aerosol was generated from puffs using 1 L/min flow rate for 5 sec (approximately 83 ml) once every min for 2.5 hr daily. Between puffs, continuous airflow of 20 L/min was used to flush the chamber. Aerosol concentration was continuously assessed and recorded from the chamber’s gas outflow line; the mean aerosol concentration was determined to be 733 mg/m3 throughout the entire exposure course. A 1:1 by volume blend of VG:PG without nicotine and flavoring generated EC and was replaced approximately every 45 min. Coils were changed weekly. RA-exposed mice experienced identical ambient exposure and housing conditions as EC-exposed mice.
Figure 1. Simplified exposure schema and representative EC delivery and generation conditions. A) a whole-body exposure system was used to expose mice to EC. B) the atomizer was fired for 5 sec every 60 sec, with corresponding particle concentration increases noted in the inhalation chamber’s outflow line at each puff (5 representative “puffs” are shown). C) the atomizer was run at 30W when fired (1 representative “puff” is shown).
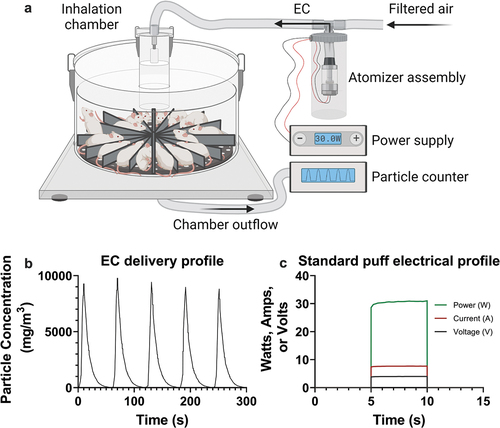
The average ventilation rate of mice (61 ml/min) was estimated using established parameters for murine tidal volume (0.2 ml) (Mitzner, Brown, and Lee Citation2001) and respiration rate (305 breaths/min) (Schaik et al. Citation1998). The inhaled concentration of the aerosol (2.2 mg/puff) was calculated using the mean ventilation rate (61 ml/min), chamber flow rate (20 L/min), and aerosol concentration in the chamber (733 mg/m3). Based upon the current literature, the concentration of aerosol deposited within the lung alveoli (550 µg/puff) was presumed to be approximately 25% of the aerosol concentration inhaled by the mice during each puff (Darquenne et al. Citation2014).
Intravital imaging of pulmonary microvasculature
EC-exposed mice (n = 5 per group) were imaged immediately following exposure (t0), 1 day after exposure (t24), or 2 days after exposure (t48). RA-exposed mice (n = 5 per group) were imaged 1 day after sham exposure. Lung intravital imaging was conducted as previously described (Bennewitz, Watkins, and Sundd Citation2014). Briefly, ketamine-xylazine anesthetized mice underwent tracheostomy ventilation with 1.5% isoflurane in oxygen to maintain anesthesia. Mice were positioned on their side and a portion of the ribcage was removed. Fluorescent labels for vasculature (FITC dextran), NETs (SYTOX Orange), neutrophils (Pacific Blue anti-mouse Ly6G mAb) and platelets (AlexaFluor647 anti-mouse CD49b mAb) were injected retro-orbitally. An intravital imaging window was affixed to exposed lung under gentle vacuum. Using a Nikon FN1 upright spinning disk confocal microscope, 15–26 random fields of view (FOVs) were recorded for 30 sec in each mouse at the interface of capillaries and arterioles or venules (Supplemental Videos 1–4). Intravital FOVs were quantified for neutrophils, platelets, neutrophil-platelet aggregates, and NETs (Figure S1). Further details of the surgery, image processing, and quantification are described in Supplemental Material.
Histology and characterization of whole blood and bronchoalveolar lavage fluid (BALF)
Separately, mice were euthanized via isoflurane overdose. Whole blood and BALF were collected by cardiac puncture and bronchoalveolar lavage of 700 μl PBS 4 times, respectively, from between n = 4 and n = 6 mice per group. Whole blood (250 µl) was added to collector tubes with heparin to prevent coagulation. Cells were pelleted (580 × g) from BALF samples and resuspended in 0.5 ml PBS. Complete blood count analysis was performed for whole blood and BALF on the IDEXX ProCyte Dx Hematology Analyzer. Lungs from RA exposed (n = 3) and each group of EC-exposed mice (n = 3) were collected and fixed in 10% buffered formalin, embedded in paraffin, sectioned, and stained with hematoxylin and eosin (H&E). Slides were assessed for immune cell infiltration and emphysematous pathology.
Myeloperoxidase (MPO), DNA, ROS, and cytokine assays
Myeloperoxidase and DNA were quantified in BALF and plasma isolated from whole blood via Mouse Myeloperoxidase DuoSet ELISA and Quant-iT™ PicoGreen™ dsDNA Assay, respectively (n = 4 or n = 5 mice per group). Similarly, plasma ROS levels were measured using OxiSelect™ In Vitro ROS/RNS Assay for H2O2 equivalency (n = 3 mice per group). Cytokine expression in EC-exposed mice was compared to RA-exposed mice by analyzing equivalent volumes of pooled plasma from n = 3 mice per group via the Proteome Profiler Mouse Cytokine Array. All assays were performed to manufacturer specifications.
Statistics
Data were plotted with GraphPad Prism 9.4. Intravital microscopy data were analyzed via nested one-way ANOVA with post-hoc Holm-Šídák correction to enable multiple comparisons. All other data were analyzed either with regular one-way ANOVA with Holm-Šídák correction when comparing multiple groups, or with two-tailed Student’s t-test when comparing all EC-exposed mice collectively only to RA-exposed mice. Significance was defined as p < 0.05.
Results
Short-term EC exposure effect on intravital imaging of neutrophil and platelet behavior in lung microvasculature
Female BALB/cJ mice between 12 and 16 weeks of age weighing approximately 21 g were exposed to room air (RA) or identical 3-day flavorant-free and nicotine-free EC exposure paradigms. Immediately following exposure (t0), 1 day post exposure (t24), or 2 days post exposure (t48), fluorescent intravital imaging was conducted on murine lungs (n = 5 per group) to determine whether EC exposure for 2.5 hr each day over 3 consecutive days altered neutrophil and platelet dynamics in the pulmonary vasculature. Representative fields of view (FOVs) showed elevated neutrophils (blue) and neutrophil-platelet aggregates (blue-pink) blocking blood flow in lung vessels of EC-exposed mice compared to mice receiving RA which exhibited robust blood flow and displayed minimal cell aggregates ().
Figure 2. Intravital confocal lung imaging reveals increased neutrophil presence, occlusive neutrophil-platelet aggregates, and thrombi in the pulmonary microvasculature of EC-exposed mice. Representative fluorescent fields of view (FOVs) from A) mice exposed to room air (RA); B) EC-exposed mice immediately after exposure (t0); C) approximately 24 hr following exposure (t24); or D) approximately 48 hr following exposure (t48) are shown. At least 15 FOVs containing venule/arteriole-capillary interfaces were randomly collected from n = 5 mice per group. Neutrophils shown in blue, platelets in pink, vasculature in green, and cell-free DNA in red. Arrows indicate blood flow direction. Red circles show neutrophil-platelet aggregates which included two or more neutrophils. Areas of SYTOX staining outside the focal plane, or SYTOX+ objects outside of the bounds of the vasculature were considered as general cell-free DNA rather than true intravascular NETs. Scale bars 20 µm. See supplementary videos 1–4 for short intravital clips of each FOV shown here.
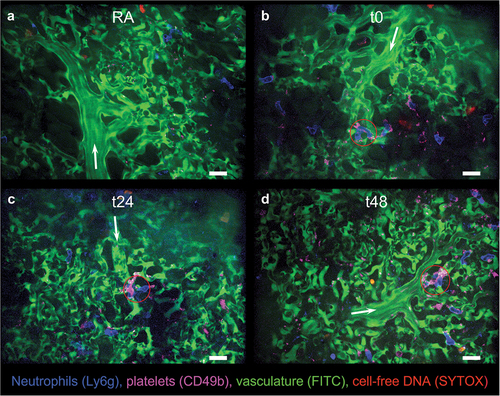
Upon image acquisition, neutrophil, platelet, and neutrophil-platelet aggregate presence were quantified within each FOV via Nikon’s NIS-Elements General Analysis 3 software, while NETs were counted manually. illustrates that neutrophils (1.7‒1.9-fold) and neutrophil-platelet aggregates (1.9‒2.5) in each FOV were significantly increased in all exposure groups compared to RA. Elevated neutrophils and neutrophil-platelet aggregates were maintained up to 2 days post EC exposure, indicating that effects of EC exposure had not been resolved. However, total platelet area and NET numbers did not appear to be markedly affected following EC exposure. Interestingly, the interquartile range of total NETs per FOV was larger in all EC-exposed groups, suggesting increased variability in NETosis within the pulmonary vasculature following EC exposure.
Figure 3. Quantification of intravital lung imaging FOVs reveals significantly and persistently increased neutrophil presence and neutrophil-platelet interaction in EC-exposed mice. A) Total neutrophil count, B) platelet area, and C) neutrophil-platelet aggregate area were measured using Nikon General Analysis software across all FOVs. D) NET analysis was conducted by randomly selecting 10 intravital FOVs per mouse across all groups and manually counting NETs. *indicates p < 0.05 using nested one-way ANOVA with Holm-Šídák correction for multiple comparisons to RA-exposed mice.
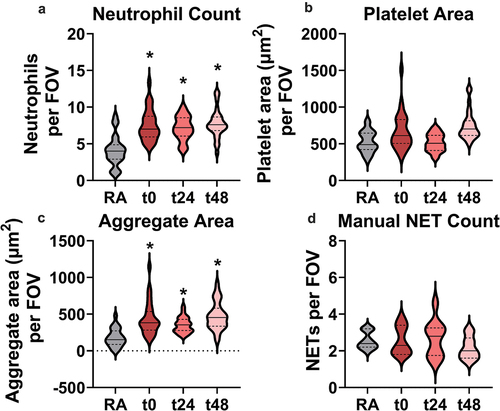
Short-term EC exposure effect on global immune cell presence in blood or alveolar space
To determine whether altered neutrophil recruitment or changes in the presence of other immune populations manifested beyond the pulmonary vasculature, immune cells were examined within whole blood and BALF collected from RA- and EC-exposed mice at each timepoint (between n = 4 and n = 6) following exposure. The presence of key cell populations, including neutrophils, lymphocytes, and monocytes were characterized using a ProCyte Dx Hematology Analyzer. In addition, platelet and total white blood cell (WBC) counts were determined for whole blood and BALF, respectively ().
Figure 4. Increases, though not statistically significant, were observed in key immune populations in blood (A-D) and BALF (E-H) from EC-exposed mice. Neutrophils (A,E), lymphocytes (B,F), monocytes (C, G), platelets (D), and total white blood cells (H) were counted with a ProCyte Dx Hematology Analyzer. No significance relative to RA-exposed mice was detected via one-way ANOVA with Holm-Šídák correction, or when comparing RA-exposed mice to all EC-exposed mice via two-tailed Student’s t-test. Error bars SEM.
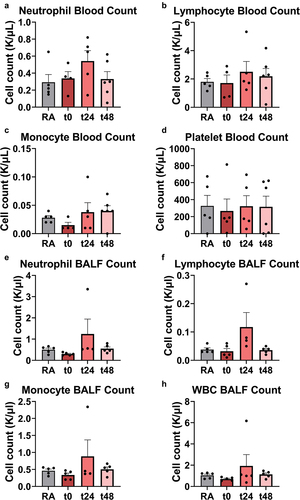
In agreement with intravital lung imaging observations, increased platelet presence was not found in whole blood. Further, monocyte count (apart from one outlier) appeared similar between all groups in blood and BALF. Most notably, quantitative elevated presence of the number of neutrophils in whole blood (1.1‒1.9-fold) and BALF (t24 and t48: 1.1‒2.5-fold) as well as lymphocytes in BALF only (t24: 3-fold), were detected, although these increases were not significant. Interestingly, localized lymphocytosis around the bronchioles was identified in 1 of n = 3 H&E-stained lung sections for each EC-exposed timepoint (Table S1, Figure S2). In addition, focal emphysematous alterations were visualized in all but one EC-exposed mouse lung. Neither of these changes occurred in the RA-exposed mice. The observed localized lymphocyte involvement after EC exposure is consistent with lung pathology acquired from cigarettes, where lymphocytes are known to mediate development of smoking induced emphysema (John-Schuster et al. Citation2014).
Influence of short-term EC exposure on neutrophil function and total ROS levels
A marked change in NETosis via DNA staining was not detected in the pulmonary microvasculature in this study. Indeed, there are conflicting reports as to whether EC exposure enhances or mitigates NETosis and other neutrophil functionality such as activation or chemotaxis (Corriden et al. Citation2020; Reidel et al. Citation2018). To complement imaging results, the presence of cell-free DNA and myeloperoxidase (MPO)- a product of neutrophil degranulation and a component of NETs- in both whole blood-derived plasma and BALF was monitored in n = 5 mice ().
Figure 5. NET component MPO, though not DNA, was significantly reduced in EC-exposed mouse plasma at t48 (A), but not in BALF (B). DNA concentration did not change significantly with EC exposure in plasma (C) or BALF (D). * indicates p < 0.05. One-way ANOVA with Holm-Šídák correction relative to RA-exposed mice was used for multiple comparisons. Error bars SEM.
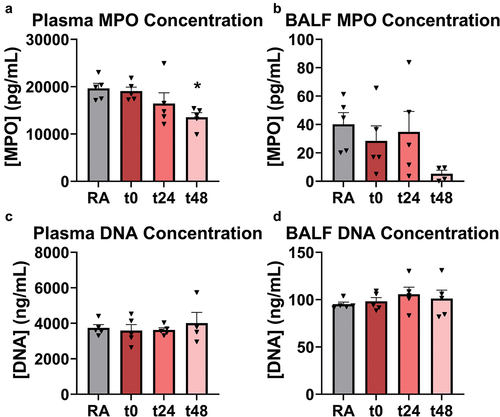
Consistent with results from the intravital imaging study, no significant alterations were observed in DNA concentration in either plasma or BALF. Interestingly, MPO was significantly reduced 1.5-fold in plasma relative to RA 2 days after EC exposure at t48. To determine whether oxidative stress mediated previously described observations of enhanced neutrophil recruitment and neutrophil-platelet aggregation in the pulmonary microvasculature, plasma ROS levels (n = 3 mice per group) were measured in RA-exposed versus EC-exposed mice at each timepoint (). However, ROS levels were not markedly different.
Figure 6. ROS was decreased in EC-exposed plasma of EC-exposed mice. This was not significant when comparing groups to RA-exposed mice using one-way ANOVA with Holm-Šídák correction. Further, no significance was detected when comparing all ECV-exposed mice to RA-exposed mice via two-tailed Student’s t-test. Error bars SEM.
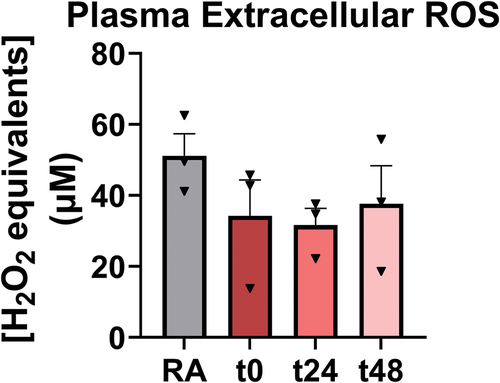
Effect of short-term EC exposure on vascular inflammation and immunomodulatory cytokine expression
To further elucidate the mechanisms underlying increased intravascular recruitment of neutrophils as well as the lymphocyte-mediated lung changes, the cytokine profiles of RA and EC-exposed mice were compared at each time point. Plasma from n = 3 mice per group was pooled to account for biological variance. Of the 40 cytokines surveyed via antibody array (blots depicted in Figure S3), 7 were altered relative to RA-exposed mice in at least one group of EC-exposed mice by ≥10% (). These included: B lymphocyte chemoattractant (BLC, or CXCL13), complement component 5 and its cleavage product (C5 and C5a, respectively), soluble intercellular adhesion molecule 1 (sICAM-1), keratinocytes-derived chemokine (KC, or CXCL1), macrophage colony stimulating factor (M-CSF), stromal cell-derived factor 1 (SDF-1, or CXCL12), and tissue inhibitor of matrix metalloproteinase 1 (TIMP-1). Of these, 4 were altered significantly relative to RA-exposed mice in at least one group of EC-exposed mice. BLC (42% increase in pixel density at t0), C5/C5a (188% rise at t24), and sICAM-1 (1,281% elevation at t0) were significantly upregulated, whereas M-CSF was significantly downregulated (47% and 33% decrease at t0 and t24, respectively). For all cytokines, the t48 timepoint values were not significantly different from those observed in RA-exposed mice, suggesting the cytokine profile begins to recover toward a normal phenotype after EC exposure ends. However, continued neutrophilia and evidence of lymphocyte-mediated lung changes at this stage suggests abnormal cell behaviors induced by EC may take longer to abate.
Figure 7. EC exposure modified cytokine expression in plasma. Altered cytokines in EC-exposed mouse plasma (n = 3 mice per membrane) were plotted as % change in pixel density relative to RA-exposed mouse plasma (n = 3 mice). Only cytokines with changes ≥10% were plotted. * indicates p < 0.05, using one-way ANOVA with Holm-Šídák correction. Error bars SEM.
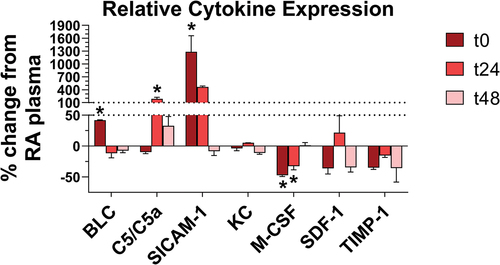
Discussion
EC exposure has been associated with increased inflammation (Glynos et al. Citation2018; Kuntic et al. Citation2020), altered neutrophil behavior (Corriden et al. Citation2020; Ghosh et al. Citation2019), impaired immune responses (Hwang et al. Citation2016; Wang et al. Citation2020), vascular changes including increased aortic stiffness and elevated endothelial oxidative stress (Chatterjee et al. Citation2019; Olfert et al. Citation2018), and enhanced platelet activation and aggregation (Hom et al. Citation2016; Qasim et al. Citation2018). Given current evidence suggesting secondhand EC-released substances initiate rapid physiological changes (McClelland et al. Citation2021) and emerging evidence that e-cigarette use may heighten susceptibility to COVID-19 complications (Wang et al. Citation2020), studies were undertaken to characterize the earliest responses to EC insult in lung tissue and pulmonary vasculature. Data indicate that short-term EC exposure results in neutrophilia and occlusive platelet-neutrophil aggregates in the lung microcirculation, persistently impaired neutrophil function, lymphocyte infiltration of the bronchioles, and altered cytokine expression.
Data surrounding the effect of EC on neutrophil behavior reports conflicting results. Some investigators indicated that EC or EC extract suppresses neutrophil NETosis, chemotaxis, phagocytosis, and antimicrobial activity (Clapp et al. Citation2017; Corriden et al. Citation2020; Hwang et al. Citation2016). Conversely, EC was also noted to promote neutrophil protease release, NETosis in response to exogenous stimuli, and neutrophil activation (Ghosh et al. Citation2019; Masso-Silva et al. Citation2021; Reidel et al. Citation2018). While this disparity may be explained by the use of different EC compositions and mode of delivery to neutrophils, it is also conceivable that not all of these changes need to be considered mutually independent.
Some elements of both an increased and suppressed inflammatory response were detected following EC exposure. Specifically, neutrophil presence and neutrophil-platelet interactions were enhanced in EC-exposed mouse lung vasculature, the latter of which is known to promote neutrophil activation (Polanowska-Grabowska et al. Citation2010). Simultaneously, significant evidence that increased vascular entrapment of neutrophils translated to higher rates of neutrophil migration out of the vasculature into the surrounding tissue was not found.
Similarly, no evidence of enhanced NETosis via either intravital imaging of EC-exposed mice or plasma cell-free DNA presence was identified, despite greater neutrophil recruitment and neutrophil-platelet interactions that are known to promote NETosis (Constantinescu-Bercu et al. Citation2020; Perdomo et al. Citation2019). Thus, these results contrast somewhat with Corriden et al. (Citation2020) in which human neutrophils were incubated with EC extract and subsequently exhibited lower rates of NETosis. Ghosh et al. (Citation2019) also found consistent results with Corriden et al. (Citation2020), where PG/VG solutions upregulated neutrophil elastase (NE) in human neutrophils only when nicotine was added. In contrast, NETosis in mice might be provoked even with the inhibition of NE (Martinod et al. Citation2016).
Interestingly, Corriden et al. (Citation2020) also found that neutrophils exposed to EC were less responsive to chemotactic stimuli in a Transwell assay. Conversely, elevated neutrophil recruitment was observed herein within the lung vasculature despite global peripheral neutrophil count remaining similar within whole blood; this finding indicated a localized rather than disseminated response. Finally, no corresponding rise in neutrophil presence was noted within BALF despite increased vascular presence. These findings are in agreement with Wang et al. (Citation2019) who noted no significant difference in neutrophil counts in BALF from mice that were exposed 2 hr daily for 3 days to PG-based EC without nicotine. Significant neutrophil elevation was only observed in mice that were treated with PG-based EC plus nicotine (Wang et al. Citation2019).
Collectively, these results suggest that EC exposure mediates neutrophil recruitment to the lung vasculature but impairs their ability to traverse the blood-air barrier to effectively respond to respiratory insult. Supporting this, the reduced presence of MPO activity in plasma suggests neutrophil azurophilic degranulation was impaired two days post-exposure, thereby diminishing effectiveness of neutrophil antimicrobial activity. These findings aid in contextualizing why EC exposure appears to enhance susceptibility to bacterial and viral respiratory infections including pseudomonas aeruginosa, influenza, and COVID-19 (Corriden et al. Citation2020; Madison et al. Citation2020; Wang et al. Citation2020). Impaired degranulation, which may result in a decreased capacity for neutrophil response to bacterial insult, coupled with the observation that neutrophils appeared to build up and undergo pro-inflammatory interactions with platelets in the lung vasculature, might account for previous observations showing neutrophils undergo both pro-inflammatory and immunosuppressive changes following EC exposure. Alternatively, elevated MPO activity may have produced an apparently lower MPO activity if the ELISA failed to detect other forms of MPO in its enzymatic cycle, which primarily generate hypochlorous acid and hydrogen peroxide to aid in antimicrobial functionality or promote inflammation (Siraki Citation2021).
In accordance with collected intravital imaging data, the hematological study that was conducted did not reveal significantly elevated platelet count in whole blood of EC-exposed mice. However, the observation that platelets aggregated more frequently with neutrophils in the vasculature of EC-exposed mice is consistent with data reporting platelets are potently activated by EC (Hom et al. Citation2016; Snoderly et al. Citation2021). Other investigators demonstrated that EC-exposed platelets express more P-selectin, which is known to mediate neutrophil-platelet interactions (Nocella et al. Citation2018; Polanowska-Grabowska et al. Citation2010).
Alveolar macrophages undergo immunosuppressive functional alterations and become lipid-laden in the lungs of e-cigarette users (Ghosh et al. Citation2021; Shields et al. Citation2020). Although macrophage presence in BALF was not directly measured, it is expected that any enhanced macrophage infiltration might occur concomitantly with higher circulating monocytes or recruited monocytes recovered from BALF; however, elevated monocyte presence was not detected. Total WBC presence was also not significantly altered in BALF. Further, it was found that M-CSF, which promotes monocyte to macrophage differentiation, was significantly reduced in plasma immediately following and one day after EC exposure, although it appeared to recover by the second day post-exposure. These findings are in contrast with Han et al. (Citation2021) who noted increased macrophage recruitment to the lungs and higher levels of M-CSF. However, Han et al. (Citation2021) utilized flavored e-liquid with nicotine over an 8-week exposure course and performed the study in a COPD mouse model. Scott et al. (Citation2018) illustrated that human alveolar macrophages exposed to nicotine-free EC condensate underwent higher rates of necrosis and apoptosis in a dose dependent manner. In addition, EC condensate exposed macrophages exhibited an inflammatory phenotype characterized by enhanced ROS production and diminished phagocytosis (Scott et al. Citation2018), indicating that even short-term EC exposure without nicotine might impact macrophage function.
The findings that circulating sICAM-1 and C5a are significantly increased after EC exposure may aid in explaining why neutrophilia occurred in the lung vasculature. Elevated levels of sICAM-1 were detected following a single session of e-cigarette use in naïve subjects, and are a known marker of inflammation and endothelial damage (Chatterjee et al. Citation2021), which may drive initial neutrophil recruitment. C5a, in addition to its role in the complement response, also aids in neutrophil chemoattraction (Guo and Ward Citation2005). In addition, SDF-1, KC, and TIMP-1 are all known to play a role in neutrophil chemoattraction with the latter two also involved in NETosis (De Filippo et al. Citation2013; Snoderly et al. Citation2022). However, EC-induced changes in the expression of each of these was not significant, suggesting EC-induced cytokine release did not markedly influence neutrophils via these mechanisms. Further, because these cytokines were measured in plasma isolated from whole blood, additional work is needed to confirm whether such changes occur specifically in the lung.
Beyond platelets and neutrophils in the vasculature, data suggest that lymphocytes may also play a role in the earliest stages of EC-induced inflammation and tissue damage. Notably, in the context of cigarette smoke exposure, increased BLC expression contributes to the progression of COPD by enhancing B-lymphocyte recruitment; the B cells then activate macrophages via IL-10 secretion (John-Schuster et al. Citation2014). In fact, IL-10 levels in this study were tested via cytokine panel, but no marked changes were detected in its expression within plasma. Importantly however, cytokine expression in BALF was not tested. Coupled with other findings that lymphocytes were transiently increased in BALF and some EC-exposed mice displayed sporadic peribronchial lymphocytosis, these findings suggest that lymphocyte recruitment to the lungs occurs immediately following even short-term EC-exposure. Although elevated BLC expression appears to recover immediately following cessation of short-term exposure, chronic exposure may promote lymphocyte recruitment and subsequent macrophage-induced COPD-like tissue damage to the lungs. Quantitative analysis of lymphocyte and macrophage involvement in EC-exposed lung tissue would serve to better elucidate whether long-term e-cigarette users are more prone to lung disease and if so, the mechanisms by which this occurs.
Data demonstrated that plasma ROS levels were numerically (though not significantly) lower in EC-exposed mice compared to RA-exposed animals. Considering pro-inflammatory processes were also observed, this is perhaps counterintuitive and may conflict with the robust body of evidence suggesting that EC promotes oxidative stress (Glynos et al. Citation2018; Kuntic et al. Citation2020; Snoderly et al. Citation2021). It is possible that the decrease in extracellular ROS may be a by-product of sampling from plasma. Suppressed nitric oxide (NO) production is a known hallmark of endothelial damage which may have resulted from neutrophil buildup within the pulmonary microvasculature, as are increased levels of sICAM-1 (Chatterjee et al. Citation2021; Constans and Conri Citation2006). Therefore, further investigation is merited as to whether plasma ROS levels following EC exposure are driven by a reduction in NO levels due to EC-induced endothelial damage. Further, although the effects of EC on the presence of reducing equivalents was not examined in the current investigation, other studies showed that e-cigarette use diminishes antioxidant capacity (Canistro et al. Citation2017). However, given that the model utilized in this study only covers the earliest stages of EC-induced dysfunction, the antioxidant defense system may not have yet been depleted and was able to compensate for enhanced ROS production at the timepoints examined, thereby depleting measurable plasma ROS levels at higher rates in EC-exposed mice. This may have resulted in apparently “lower” ROS in plasma.
Alternatively, Corriden et al. (Citation2020) showed that EC-exposed neutrophils appear less responsive to ROS-mediated NETosis. Thus, if neutrophil responses to ROS were also impaired in the model utilized in the current study, it might explain why no increase was detected in NET-like structures in EC-exposed mice. Neutrophils themselves may produce fewer ROS as a consequence of apparent reduction in azurophilic granule release as evidenced by the observed reduced MPO activity presence. In any case, it should be noted that the measured ROS levels in plasma may not correlate reliably with the oxidative functionality of the neutrophils found which may be being stimulated toward a pro-inflammatory phenotype within the pulmonary vasculature. In addition, aside from neutrophils, monocytes and macrophages are also potent producers of ROS; however, ROS production within specific subsets of cells was not conducted. Assessing this might better explain the observed results. Further investigations examining 1) total antioxidant capacity, 2) presence of specific oxidative species, 3) activities of antioxidative enzymes, and 4) oxidative capacity of neutrophils and macrophages isolated specifically from lung tissue might better contextualize these data in relation to the altered redox state noted in e-cigarette users.
To the authors’ knowledge, this is the first time the aftermath of EC exposure has been visualized in the living mouse lung. In addition, changes to immune cell population recruitment and behavior in mice after only 3 days of unflavored, non-nicotine EC exposure was presented for the first time. Further, the use of a whole-body exposure system coupled with a short-term exposure model involving otherwise naïve mice makes these findings relevant in defining the risks of directly inhaled secondhand EC exposure. Short-term EC exposure produced altered neutrophil recruitment, neutrophil-platelet aggregation, neutrophil granule release, and blood oxidative stress that persisted for days after exposure had ceased.
Limitations
However, this study has key limitations that need to be further explored in future investigations. For one, the role of gender differences was not investigated between male and female mice. Such differences were documented, whether in behavioral patterns such as e-cigarette use rates (Mayer et al. Citation2020) or in gender-dependent neutrophil responses, which were reported in mice. Specifically, elevated neutrophil presence was observed in BALF from female, but not male, mice following exposure to EC generated from PG alone (Wang et al. Citation2020). Conversely, gender-dependent neutrophil responses to EC have yet to be detected in humans. Indeed, elevated NE and matrix metalloproteinase levels, both associated with neutrophil activity, were found to be conserved across gender in BALF from e-cigarette users (Ghosh et al. Citation2019). Gender-dependent differences in murine immunologic response to EC merit thorough investigation, as do the questions of whether and to what extent such differences might occur in humans.
Reduction in particle size due to aerosol evaporation was not accounted for in this study. Knowing that PG/VG exhibit different evaporation rates, the impact on the aerosol mass concentration in the chamber, if any, was not tested. However, mice consistently received identical EC exposure with 1:1 PG:VG mixture, suggesting no differential influence of particle size on the biological findings observed within the animals at the time points assessed post exposure. Further, the impact of different ratios of PG:VG was not investigated herein and it is unclear which component contributes more to observed inflammatory responses and cellular toxicity. However, non-nicotine and non-flavored mixtures with higher PG content than used in this study were reported to exhibit greater toxicity in human gingival epithelial cells post 24 hr EC exposure (Beklen and Uckan Citation2021). Further, due to their different thermal properties, PG was found to be dominant in 1:1 PG/VG solutions during low-power EC exposure (Talih et al. Citation2017). As the power approached 16 W, increased contribution of PG to overall EC composition diminished, indicating that wattage settings may also play a role in the biological effects noted following EC exposure.
Finally, few of the current studies were able to isolate neutrophils from the surrounding environment, which speaks to the need for reliable in vitro EC exposure paradigms which mimic “physiologic” use. Models mimicking 1) structure of the vasculature including the blood-air barrier, 2) immune composition of pulmonary peripheral blood, and 3) actual EC exposure of cells (as opposed to current models incubating cellular populations of interest with EC condensate or e-liquid directly) are needed to determine the consequences of EC exposure in the complex pulmonary microenvironment. Such models might enable further dissection of the effect of EC on neutrophils’ axis of inflammatory versus anti-microbial activity.
Conclusions
Overall, data presented in the current study supported the overarching hypothesis that EC exposure leads to 1) modified hemodynamics in the pulmonary vasculature, 2) altered immune functionality, and 3) a pro-inflammatory state. This study is the first to examine the impact of EC exposure through the lens of intravital imaging and adds to the growing body of evidence that e-cigarette use rapidly leads to dysfunctions at the cellular level. It is critical to determine whether these patterns occur as subclinical pathologies in human pulmonary vasculature and lung tissue in order to understand potential pathologies that may emerge in patients that use e-cigarettes long term.
Author contributions
Conceptualization- H.T.S., H.A., I.M.O, T.R.N., and M.F.B.; methodology- H.T.S., H.A., K.L.W., I.M.O., and M.F.B.; formal analysis- H.T.S., H.A., D.M.P, K.L.W., J.N.V., and S.P.S.; investigation- H.T.S., H.A., D.M.P., K.L.W., and K.A.F.; writing – original draft preparation- H.T.S., H.A., D.M.P., and M.F.B.; writing – review and editing- H.T.S., H.A., D.M.P., K.L.W, I.M.O., T.R.N., M.F.B.; visualization- H.T.S., H.A., D.M.P, K.L.W., S.P.S., and M.F.B.; supervision- M.F.B.; project administration- H.T.S. and M.F.B; funding acquisition- H.T.S., T.R.N., and M.F.B. All authors have read and agreed to the published version of the manuscript.
Supplemental Material
Download MS Word (14.5 MB)Supplemental Material
Download MP4 Video (259.1 MB)Supplemental Material
Download MP4 Video (282.1 MB)Supplemental Material
Download MP4 Video (90.6 MB)Supplemental Material
Download MP4 Video (89.8 MB)Acknowledgments
The authors would like to thank Dr. Elizabeth Bowdridge for assisting in inhalation equipment scheduling and providing consumables. The authors would also like to thank the West Virginia University Microscope Imaging Facility for use of their equipment including the Olympus Whole Slide Scanner and the NIS Elements General Analysis software, as well as the West Virginia University Histopathology and Tissue Bank Core for their assistance in paraffin embedding, sectioning, and hematoxylin and eosin staining fixed lung samples. Finally, the authors acknowledge that the original diagram illustrated in was created with BioRender.com.
Disclosure statement
No potential conflict of interest was reported by the authors.
Data availability statement
All supportive data are available in the supplementary material. Raw data files should be requested from the corresponding author ([email protected]).
Supplementary data
Supplemental data for this article can be accessed online at https://doi.org/10.1080/15287394.2023.2184738
Additional information
Funding
References
- Beklen, A., and D. Uckan. 2021. Electronic cigarette liquid substances propylene glycol and vegetable glycerin induce an inflammatory response in gingival epithelial cells. Hum. Exp. Toxicol. 40 (1):25–34. doi:10.1177/0960327120943934.
- Bennewitz, M. F., S. C. Watkins, and P. Sundd. 2014. Quantitative intravital two-photon excitation microscopy reveals absence of pulmonary vaso-occlusion in unchallenged sickle cell disease mice. Intravital 3 (2):e29748. doi:10.4161/intv.29748.
- Bhat, T. A., S. G. Kalathil, P. N. Bogner, B. C. Blount, M. L. Goniewicz, and Y. M. Thanavala. 2020. An animal model of inhaled vitamin E acetate and EVALI-like lung injury. N. Engl. J. Med. 382 (12):1175–77. doi:10.1056/NEJMc2000231.
- Blount, B. C., M. P. Karwowski, P. G. Shields, M. Morel-Espinosa, L. Valentin-Blasini, M. Gardner, M. Braselton, C. R. Brosius, K. T. Caron, D. Chambers, et al. 2020. Vitamin E acetate in bronchoalveolar-lavage fluid associated with EVALI. N. Engl. J. Med. 382 (8):697–705. doi:10.1056/NEJMoa1916433.
- Bracken-Clarke, D., D. Kapoor, A. M. Baird, P. J. Buchanan, K. Gately, S. Cuffe, and S. P. Finn. 2021. Vaping and lung cancer–a review of current data and recommendations. Lung Cancer 153:11–20. doi:10.1016/j.lungcan.2020.12.030.
- Canistro, D., F. Vivarelli, S. Cirillo, C. Babot Marquillas, A. Buschini, M. Lazzaretti, L. Marchi, V. Cardenia, M. T. Rodriguez-Estrada, M. Lodovici, et al. 2017. E-cigarettes induce toxicological effects that can raise the cancer risk. Sci Rep 7 (1):1–9. doi:10.1038/s41598-017-02317-8.
- Caudrillier, A., and M. R. Looney. 2012. Platelet-neutrophil interactions as a target for prevention and treatment of transfusion-related acute lung injury. Curr. Pharm. Des. 18 (22):3260–66. doi:10.2174/1381612811209023260.
- Chatterjee, S., A. Caporale, J. Q. Tao, W. Guo, A. Johncola, A. A. Strasser, F. T. Leone, M. C. Langham, and F. W. Wehrli. 2021. Acute e-cig inhalation impacts vascular health: A study in smoking naïve subjects. American J. Physiol. Heart Circ. Physiol. 320 (1):H144–58. doi:10.1152/ajpheart.00628.2020.
- Chatterjee, S., J. -Q. Tao, A. Johncola, W. Guo, A. Caporale, M. C. Langham, and F. W. Wehrli. 2019. Acute exposure to e-cigarettes causes inflammation and pulmonary endothelial oxidative stress in nonsmoking, healthy young subjects. American J. Physiol. Lung Cell. Mol. Physiol. 317 (2):L155–66. doi:10.1152/ajplung.00110.2019.
- Clapp, P. W., E. A. Pawlak, J. T. Lackey, J. E. Keating, S. L. Reeber, G. L. Glish, and I. Jaspers. 2017. Flavored e-cigarette liquids and cinnamaldehyde impair respiratory innate immune cell function. American J. Physiol. Lung Cell. Mol. Physiol. 313 (2):L278–92. doi:10.1152/ajplung.00452.2016.
- Constans, J., and C. Conri. 2006. Circulating markers of endothelial function in cardiovascular disease. Clin. Chimica Acta 368 (1–2):33–47. doi:10.1016/j.cca.2005.12.030.
- Constantinescu-Bercu, A., L. Grassi, M. Frontini, I. I. Salles-Crawley, K. Woollard, and J. T. Crawley. 2020. Activated αIIbβ3 on platelets mediates flow-dependent NETosis via SLC44A2. Elife 9:e53353. doi:10.7554/eLife.53353.
- Corriden, R., A. Moshensky, C. M. Bojanowski, A. Meier, J. Chien, R. K. Nelson, and L. E. Crotty Alexander. 2020. E-cigarette use increases susceptibility to bacterial infection by impairment of human neutrophil chemotaxis, phagocytosis, and NET formation. American J. Physiol. Cell Physiol. 318 (1):C205–14. doi:10.1152/ajpcell.00045.2019.
- Darquenne, C., M. G. Borja, J. M. Oakes, E. C. Breen, I. M. Olfert, M. Scadeng, and G. K. Prisk. 2014. Increase in relative deposition of fine particles in the rat lung periphery in the absence of gravity. J. Appl. Physiol. 117 (8):880–86. doi:10.1152/japplphysiol.00298.2014.
- De Filippo, K., A. Dudeck, M. Hasenberg, E. Nye, N. van Rooijen, K. Hartmann, M. Gunzer, A. Roers, and N. Hogg. 2013. Mast cell and macrophage chemokines CXCL1/CXCL2 control the early stage of neutrophil recruitment during tissue inflammation. Blood 121 (24):4930–37. doi:10.1182/blood-2013-02-486217.
- Effah, F., B. Taiwo, D. Baines, A. Bailey, and T. Marczylo. 2022. Pulmonary effects of e-liquid flavors: A systematic review. J. Toxicol. Environ. Health Part B 25 (7):343–71. doi:10.1080/10937404.2022.2124563.
- Etulain, J., K. Martinod, S. L. Wong, S. M. Cifuni, M. Schattner, and D. D. Wagner. 2015. P-selectin promotes neutrophil extracellular trap formation in mice. Blood 126 (2):242–46. doi:10.1182/blood-2015-01-624023.
- Feldman, R., M. Stanton, and E. M. Suelzer. 2021. Compiling evidence for EVALI: A scoping review of in vivo pulmonary effects after inhaling vitamin E or vitamin E acetate. J. Med. Toxicol. 17 (3):278–88. doi:10.1007/s13181-021-00823-w.
- Gellatly, S., N. Pavelka, T. Crue, K. S. Schweitzer, B. J. Day, E. Min, M. Numata, D. R. Voelker, A. Scruggs, and I. Petrache. 2020. Nicotine-free e-cigarette vapor exposure stimulates IL6 and mucin production in human primary small airway epithelial cells. J. Inflamm. Res. 13:175. doi:10.2147/JIR.S244434.
- Ghosh, A., S. Ahmad, R. D. Coakley, M. F. Sassano, N. E. Alexis, and R. Tarran. 2021. Lipid-laden macrophages are not unique to patients with E-cigarette or vaping product use–associated lung injury. Am. J. Respir. Crit. Care Med. 203 (8):1030–33. doi:10.1164/rccm.202009-3507LE.
- Ghosh, A., R. D. Coakley, A. J. Ghio, M. S. Muhlebach, C. R. Esther Jr, N. E. Alexis, and R. Tarran. 2019. Chronic e-cigarette use increases neutrophil elastase and matrix metalloprotease levels in the lung. Am. J. Respir. Crit. Care Med. 200 (11):1392–401. doi:10.1164/rccm.201903-0615OC.
- Glynos, C., S. -I. Bibli, P. Katsaounou, A. Pavlidou, C. Magkou, V. Karavana, S. Topouzis, I. Kalomenidis, S. Zakynthinos, and A. Papapetropoulos. 2018. Comparison of the effects of e-cigarette vapor with cigarette smoke on lung function and inflammation in mice. American J. Physiol. Lung Cell. Mol. Physiol. 315 (5):L662–72. doi:10.1152/ajplung.00389.2017.
- Guo, R. -F., and P. A. Ward. 2005. Role of C5a in inflammatory responses. Annu. Rev. Immunol. 23 (1):821–52. doi:10.1146/annurev.immunol.23.021704.115835.
- Han, H., G. Peng, M. Meister, H. Yao, J. J. Yang, M. -H. Zou, Z. -R. Liu, and X. Ji. 2021. Electronic cigarette exposure enhances lung inflammatory and fibrotic responses in COPD mice. Front Pharmacol 12. doi:10.3389/fphar.2021.726586.
- Hom, S., L. Chen, T. Wang, B. Ghebrehiwet, W. Yin, and D. A. Rubenstein. 2016. Platelet activation, adhesion, inflammation, and aggregation potential are altered in the presence of electronic cigarette extracts of variable nicotine concentrations. Platelets 27 (7):694–702. doi:10.3109/09537104.2016.1158403.
- Hwang, J. H., M. Lyes, K. Sladewski, S. Enany, E. McEachern, D. P. Mathew, S. Das, A. Moshensky, S. Bapat, D. T. Pride, et al. 2016. Electronic cigarette inhalation alters innate immunity and airway cytokines while increasing the virulence of colonizing bacteria. J. Mol. Med. 94 (6):667–79. doi:10.1007/s00109-016-1378-3.
- John-Schuster, G., K. Hager, T. M. Conlon, M. Irmler, J. Beckers, O. Eickelberg, and A. Ö. Yildirim. 2014. Cigarette smoke-induced iBALT mediates macrophage activation in a B cell-dependent manner in COPD. American J. Physiol. Lung Cell. Mol. Physiol. 307 (9):L692–706. doi:10.1152/ajplung.00092.2014.
- Kim, S. -J., and C. N. Jenne. 2016. Role of platelets in neutrophil extracellular trap (NET) production and tissue injury. Semin. Immunol. 28 (6):546–54. doi:10.1016/j.smim.2016.10.013.
- Kim, Y. -Y., M. -K. Kim, and H. -S. Shin. 2022. Determination of volatile organic compounds (VOCs) levels from various smoking cessation aids by using gas chromatography-mass spectrometry methodology. J. Toxicol. Environ. Health Part A 85 (3):110–20. doi:10.1080/15287394.2021.1979436.
- Kuntic, M., M. Oelze, S. Steven, S. Kröller-Schön, P. Stamm, S. Kalinovic, K. Frenis, K. Vujacic-Mirski, M. T. Bayo Jimenez, M. Kvandova, et al. 2020. Short-term e-cigarette vapour exposure causes vascular oxidative stress and dysfunction: Evidence for a close connection to brain damage and a key role of the phagocytic NADPH oxidase (NOX-2). Eur. Heart J. 41 (26):2472–83. doi:10.1093/eurheartj/ehz772.
- Lechasseur, A., C. -A. Huppé, M. Talbot, J. Routhier, S. Aubin, M. -J. Beaulieu, C. Duchaine, D. Marsolais, and M. C. Morissette. 2020. Exposure to nicotine-free and flavor-free e-cigarette vapors modifies the pulmonary response to tobacco cigarette smoke in female mice. American J. Physiol. Lung Cell. Mol. Physiol. 319 (4):L717–27. doi:10.1152/ajplung.00037.2020.
- Lerner, C. A., I. K. Sundar, H. Yao, J. Gerloff, D. J. Ossip, S. McIntosh, R. Robinson, I. Rahman, and M. F. Khan. 2015. Vapors produced by electronic cigarettes and e-juices with flavorings induce toxicity, oxidative stress, and inflammatory response in lung epithelial cells and in mouse lung. PloS One 10 (2):e0116732. doi:10.1371/journal.pone.0116732.
- Madison, M. C., C. T. Landers, B. -H. Gu, C. -Y. Chang, H. -Y. Tung, R. You, M. J. Hong, N. Baghaei, L. -Z. Song, and P. Porter. 2020. Electronic cigarettes disrupt lung lipid homeostasis and innate immunity independent of nicotine. J. Clin. Invest. 129 (10):4290–304. doi:10.1172/JCI128531.
- Martinod, K., T. Witsch, K. Farley, M. Gallant, E. Remold-o’donnell, and D. Wagner. 2016. Neutrophil elastase-deficient mice form neutrophil extracellular traps in an experimental model of deep vein thrombosis. J. Thromb. Haemostasis 14 (3):551–58. doi:10.1111/jth.13239.
- Masso-Silva, J. A., A. Moshensky, J. Shin, J. Olay, S. Nilaad, I. Advani, C. M. Bojanowski, S. Crotty, W. T. Li, W. M. Ongkeko, et al. 2021. Chronic E-cigarette aerosol inhalation alters the immune state of the lungs and increases ACE2 expression, raising concern for altered response and susceptibility to SARS-CoV-2. Front Physiol 12:563. doi:10.3389/fphys.2021.649604.
- Mayer, M., C. Reyes-Guzman, R. Grana, K. Choi, and N. D. Freedman. 2020. Demographic characteristics, cigarette smoking, and e-cigarette use among US adults. JAMA Netw. Open 3 (10):e2020694. doi:10.1001/jamanetworkopen.2020.20694.
- McClelland, M. L., C. S. Sesoko, D. A. MacDonald, L. M. Davis, and S. C. McClelland. 2021. The immediate physiological effects of e-cigarette use and exposure to second hand e-cigarette vapor. Respir Care 66 (6):943–50. doi:10.4187/respcare.08596.
- Mitzner, W., R. Brown, and W. Lee. 2001. In vivo measurement of lung volumes in mice. Physiol. Genomics 4 (3):215–21. doi:10.1152/physiolgenomics.2001.4.3.215.
- Miyashita, L., R. Suri, E. Dearing, I. Mudway, R. E. Dove, D. R. Neill, R. Van Zyl-Smit, A. Kadioglu, and J. Grigg. 2018. E-cigarette vapour enhances pneumococcal adherence to airway epithelial cells. Eur. Respir. J. 51 (2):1701592. doi:10.1183/13993003.01592-2017.
- Muthumalage, T., T. Lamb, M. R. Friedman, and I. Rahman. 2019. E-cigarette flavored pods induce inflammation, epithelial barrier dysfunction, and DNA damage in lung epithelial cells and monocytes. Sci Rep 9 (1):1–11. doi:10.1038/s41598-019-51643-6.
- Nocella, C., G. Biondi-Zoccai, S. Sciarretta, M. Peruzzi, F. Pagano, L. Loffredo, P. Pignatelli, C. Bullen, G. Frati, and R. Carnevale. 2018. Impact of tobacco versus electronic cigarette smoking on platelet function. Am. J. Cardiol. 122 (9):1477–81. doi:10.1016/j.amjcard.2018.07.029.
- Olfert, I. M., E. DeVallance, H. Hoskinson, K. W. Branyan, S. Clayton, C. R. Pitzer, D. P. Sullivan, M. J. Breit, Z. Wu, P. Klinkhachorn, et al. 2018. Chronic exposure to electronic cigarettes results in impaired cardiovascular function in mice. J. Appl. Physiol. 124 (3):573–82. doi:10.1152/japplphysiol.00713.2017.
- Ortiz-Muñoz, G., B. Mallavia, A. Bins, M. Headley, M. F. Krummel, and M. R. Looney. 2014. Aspirin-triggered 15-epi-lipoxin A4 regulates neutrophil-platelet aggregation and attenuates acute lung injury in mice. Blood 124 (17):2625–34. doi:10.1182/blood-2014-03-562876.
- Perdomo, J., H. H. Leung, Z. Ahmadi, F. Yan, J. J. Chong, F. H. Passam, and B. H. Chong. 2019. Neutrophil activation and NETosis are the major drivers of thrombosis in heparin-induced thrombocytopenia. Nat. Commun. 10 (1):1–14. doi:10.1038/s41467-019-09160-7.
- Polanowska-Grabowska, R., K. Wallace, J. J. Field, L. Chen, M. A. Marshall, R. Figler, A. R. Gear, and J. Linden. 2010. P-selectin–mediated platelet-neutrophil aggregate formation activates neutrophils in mouse and human sickle cell disease. Arterioscler. Thromb. Vasc. Biol. 30 (12):2392–99. doi:10.1161/ATVBAHA.110.211615.
- Qasim, H., Z. A. Karim, J. C. Silva-espinoza, F. T. Khasawneh, J. O. Rivera, C. C. Ellis, S. L. Bauer, I. C. Almeida, and F. Z. Alshbool. 2018. Short-term E-cigarette exposure increases the risk of thrombogenesis and enhances platelet function in mice. J Am Heart Assoc 7 (15):e009264. doi:10.1161/JAHA.118.009264.
- Ramirez, J. E. M., Z. A. Karim, A. B. Alarabi, K. R. Hernandez, Z. B. Taleb, J. O. Rivera, F. T. Khasawneh, and F. Z. Alshbool. 2020. The JUUL e-cigarette elevates the risk of thrombosis and potentiates platelet activation. J. Cardiovasc. Pharmacol. Ther. 25 (6):578–86. doi:10.1177/1074248420941681.
- Reidel, B., G. Radicioni, P. W. Clapp, A. A. Ford, S. Abdelwahab, M. E. Rebuli, P. Haridass, N. E. Alexis, I. Jaspers, and M. Kesimer. 2018. E-cigarette use causes a unique innate immune response in the lung, involving increased neutrophilic activation and altered mucin secretion. Am. J. Respir. Crit. Care Med. 197 (4):492–501. doi:10.1164/rccm.201708-1590OC.
- Schaik, S. M. V., G. Enhorning, I. Vargas, and R. C. Welliver. 1998. Respiratory syncytial virus affects pulmonary function in BALB/c mice. J. Infect. Dis. 177 (2):269–76. doi:10.1086/514208.
- Schick, S. F., B. C. Blount, P. Jacob, N. A. Saliba, J. T. Bernert, A. El Hellani, P. Jatlow, R. S. Pappas, L. Wang, J. Foulds, et al. 2017. Biomarkers of exposure to new and emerging tobacco delivery products. American J. Physiol. Lung Cell. Mol. Physiol. 313 (3):L425–52. doi:10.1152/ajplung.00343.2016.
- Scott, A., S. T. Lugg, K. Aldridge, K. E. Lewis, A. Bowden, R. Y. Mahida, F. S. Grudzinska, D. Dosanjh, D. Parekh, R. Foronjy, et al. 2018. Pro-inflammatory effects of e-cigarette vapour condensate on human alveolar macrophages. Thorax 73 (12):1161–69. doi:10.1136/thoraxjnl-2018-211663.
- Shields, P. G., M. -A. Song, J. L. Freudenheim, T. M. Brasky, J. P. McElroy, S. A. Reisinger, D. Y. Weng, R. Ren, T. Eissenberg, M. D. Wewers, et al. 2020. Lipid laden macrophages and electronic cigarettes in healthy adults. EBioMedicine 60:102982. doi:10.1016/j.ebiom.2020.102982.
- Siraki, A. G. 2021. The many roles of myeloperoxidase: From inflammation and immunity to biomarkers, drug metabolism and drug discovery. Redox Biol 46:102109. doi:10.1016/j.redox.2021.102109.
- Snoderly, H. T., B. A. Boone, and M. F. Bennewitz. 2019. Neutrophil extracellular traps in breast cancer and beyond: Current perspectives on NET stimuli, thrombosis and metastasis, and clinical utility for diagnosis and treatment. Breast Cancer Res. 21 (1):1–13. doi:10.1186/s13058-019-1237-6.
- Snoderly, H. T., K. A. Freshwater, C. Martinez de la Torre, D. M. Panchal, J. N. Vito, and M. F. Bennewitz. 2022. Pegylation of metal oxide nanoparticles modulates neutrophil extracellular trap formation. Biosensors 12 (2):123. doi:10.3390/bios12020123.
- Snoderly, H. T., T. R. Nurkiewicz, E. C. Bowdridge, and M. F. Bennewitz. 2021. E-cigarette use: Device market, study design, and emerging evidence of biological consequences. Int J Mol Sci 22 (22):12452. doi:10.3390/ijms222212452.
- Stefaniak, A. B., R. F. LeBouf, A. C. Ranpara, and S. S. Leonard. 2021. Toxicology of flavoring-and cannabis-containing e-liquids used in electronic delivery systems. Pharmacol. Ther. 224:107838. doi:10.1016/j.pharmthera.2021.107838.
- Talih, S., Z. Balhas, R. Salman, R. El-Hage, N. Karaoghlanian, A. El-Hellani, M. Baassiri, E. Jaroudi, T. Eissenberg, N. Saliba, et al. 2017. Transport phenomena governing nicotine emissions from electronic cigarettes: Model formulation and experimental investigation. Aerosol Sci. Technol. 51 (1):1–11. doi:10.1080/02786826.2016.1257853.
- Wang, Q., N. Ahmad Khan, T. Muthumalage, G. R. Lawyer, S. R. McDonough, T. -D. Chuang, M. Gong, I. K. Sundar, V. K. Rehan, and I. Rahman. 2019. Dysregulated repair and inflammatory responses by e-cigarette-derived inhaled nicotine and humectant propylene glycol in a sex-dependent manner in mouse lung. FASEB Bioadv. 1 (10):609–23. doi:10.1096/fba.2019-00048.
- Wang, L., M. Rajavel, C. -W. Wu, C. Zhang, M. Poindexter, C. Fulgar, T. Mar, J. Singh, J. K. Dhillon, J. Zhang, et al. 2022. Effects of life-stage and passive tobacco smoke exposure on pulmonary innate immunity and influenza infection in mice. J. Toxicol. Environ. Health Part A 85 (11):439–56. doi:10.1080/15287394.2022.2032518.
- Wang, Q., I. K. Sundar, D. Li, J. H. Lucas, T. Muthumalage, S. R. McDonough, and I. Rahman. 2020. E-cigarette-induced pulmonary inflammation and dysregulated repair are mediated by nAchr α7 receptor: Role of nAchr α7 in SARS-CoV-2 Covid-19 ACE2 receptor regulation. Respir. Res. 21 (1):1–17. doi:10.1186/s12931-020-01396-y.
- Wu, Q., D. Jiang, M. Minor, H. W. Chu, and S. Jeyaseelan. 2014. Electronic cigarette liquid increases inflammation and virus infection in primary human airway epithelial cells. PloS One 9 (9):e108342. doi:10.1371/journal.pone.0108342.
- Zarbock, A., K. Singbartl, and K. Ley. 2006. Complete reversal of acid-induced acute lung injury by blocking of platelet-neutrophil aggregation. J. Clin. Invest. 116 (12):3211–19. doi:10.1172/JCI29499.