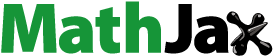
Abstract
Carbon nanotubes (CNTs) are attracting attention as catalyst carriers due to their unique combination of properties such as high mechanical strength, large aspect ratio, large surface area, outstanding optical properties, high thermal conductivity, and electrical conductivity. These properties make them suitable for electrocatalyst applications such as fuel cells, batteries, sensors, and organic synthesis. The use of CNTs as catalytic carriers for these devices improves catalytic activity and thus performance. This article presents several assemblages and incorporation practices for CNT-based composites in catalyst structuring for various applications. The structure and defects of CNTs are the main factors affecting the catalytic activity of fuel cells and batteries. CNTs synthesis methods and composite design are still active research interests in the field of electrocatalysis. This review focuses on carbon nanotubes and their composites used in the production of non-precious metal catalysts in fuel cells, batteries, sensors, oxygen reduction, oxygen evolution, hydrogen evolution, and high-performance water splitting.
1. Introduction
Carbon Nanotubes (CNTs) are a kind of tubular structure typically having nanometer scaled diameter and micrometer-scaled length. CNTs are essentially composed of pure carbon atoms that interact with each other via strong sp2 carbon-carbon chemical bonds to form the unique geometry of the carbon network. This gives CNTs attractive properties such as electronic, mechanical, and thermal properties. Carbon nanotubes are divided into two types based on the number of graphene layers: Single-Walled Carbon Nanotubes (SWCNTs) and Multi-Walled Carbon Nanotubes (MWCNTs). SWCNTs consist of a single sheet of graphene seamlessly wrapped into a cylindrical tube and MWCNTs comprising of an array of SWCNTs of different diameters that are concentrically nested into each other. The strong chemical bonding in the carbon network endows CNTs to be the most fascinating and enchant nanomaterials of the 21st century with many attractive physicochemical properties such as high mechanical (elasticity: ∼1 TPa and tensile strength: 50–500 GPa), thermal stability (>700 °C), and electrical conductivity (3000–3500 W/m K).[Citation1]
The unique properties of each type of SWCNT, in turn, determine their possible application. Many potential applications of CNTs include transparent conducting films; conductive and high-strength nanocomposites; advanced energy storage and energy conversion devices; sensors; field emission displays; nanometer-sized semiconductor devices; biological probes, and electrical interconnects. Even though, the SWCNTs that make up the concentric layers of MWCNTs can be a mixture of semiconducting and metallic shells, the dominant properties of the metallic shells make MWCNTs predominantly metallic. The electronic and structural properties of MWCNTs just like graphene can be modified by doping. Indeed, numerous studies have shown that the electronic and structural properties of MWCNTs can be modified by the use of nitrogen (N) or boron (B) containing precursors during the Chemical Vapor Deposition (CVD) synthesis technique, forming N doped or B doped CNTs.[Citation2] This review paper tries to address the synthesis and structure of CNTs, an overview of electrocatalysis, and the application of CNT-based composites for electrocatalysis purposes.
2. Synthesis methods and structure of CNTs
Since most of the material scientists are trying to produce CNTs in cheaper ways, many new techniques are coming out every day. Interestingly, most of them use common experimental parameters such as carbon feedstock, metal catalysts, and temperature. Currently used six different CNT synthesis methods, which include Arc Discharge (AD), Laser Ablation (LA), CVD, electrolysis, hydrothermal/sonochemical, and template/bottom-up methods. Laser Ablation and template/bottom-up are dedicated to the synthesis of SWCNTs, whereas, CVD, electrolysis, and hydrothermal methods are more suitable for MWCNTs. AD has been applied for the synthesis of both short SWCNTs and MWCNTs.[Citation3] Generally, the three methods (AD, LA, and CVD) are the most widely known techniques for making carbon nanotubes as presented in .
Figure 1. Carbon nanotube synthesis techniques: (A) Arc discharge method, (B) Laser ablation method, (C) chemical vapor disposition methods adopted from Ref. [Citation4].
![Figure 1. Carbon nanotube synthesis techniques: (A) Arc discharge method, (B) Laser ablation method, (C) chemical vapor disposition methods adopted from Ref. [Citation4].](/cms/asset/a8cce42d-2a93-4415-b66b-fc1480217ad2/lfnn_a_2028278_f0001_c.jpg)
The carbon atoms that lead to the formation of CNTs are released through a process using electricity (AD method), high-power lasers (LA method), and heat (CVD). Although considerable advancement has been made in controlling the properties of CNTs during growth, none of the current synthetic techniques has been able to produce identical populations of CNTs. The produced samples always contain CNTs varying in their purity (metal particles, metal oxides, amorphous carbon) and structures (diameter and chiral angle). Thus, purification to the highest purity after synthesis is another challenge.[Citation5]
The carbon atoms in the tubes are arranged in hexagonal shape, forming strong covalent bonds among themselves with a carbon (C)–carbon (C) bond distance of approximately 0.14 nm. The method of winding a graphene sheet is represented by a pair of subscript letters (n, m) called a chiral vector. The integers n and m represent the number of unit vectors along with the two directions of the graphene honeycomb lattice. When m = 0, the nanotubes are called “zigzags” and are named after the hexagonal pattern around the tube. When n = m, the nanotubes are called "armchairs" and describe the hexagon of the carbon atom as one of the two confirmers of cyclohexene. Otherwise, they are called "chiral" and the m value lies between the zigzag shape and the structure of the chair. The word chirality means handedness, which means that the tube can be rotated in either direction.[Citation3] It is possible to have metallic SWCNTs (m-SWCNTs) or semiconducting (s-SWCNTs) with different energy gaps, depending on the helicity and diameter of the tubes, i.e. (n, m) indices which is related to their geometric structure. The growing CNT nozzle cap is usually composed of pentagonal and hexagonal structures but commonly complies with the pentagonal role. Since MWCNTs are constructed by joining SWCNTs together, they can be used to describe MWCNT structures. represents the formation of a SWCNT by rolling up a single graphene sheet.
Figure 2. Chirality map of SWCNTs, in which the met- and semi-SWCNTs are denoted by hexagons, reproduced from Ref. [Citation6] with permission from the Royal Society of Chemistry.
![Figure 2. Chirality map of SWCNTs, in which the met- and semi-SWCNTs are denoted by hexagons, reproduced from Ref. [Citation6] with permission from the Royal Society of Chemistry.](/cms/asset/8c1bdffc-2249-447e-b2ea-86b2739187a0/lfnn_a_2028278_f0002_c.jpg)
One-dimensional SWCNT is commonly expressed by the chiral vector (Ch) and the translation vector (T). Both vectors (Ch and T) determine CNT unit size and is commonly expressed by the Bravais lattice vectors a1 and a2 as follows:
(1)
(1)
(2)
(2)
where a1 and a2 are the unit vectors, n and m are integers and W is the ultimate common divisor of the expressions n + 2 m and 2n + m. Those nanotubes following this construction are called (n, m) nanotubes (i.e. SWCNTs). The magnitude of the vector Ch can be used to calculate CNT radius by R = Ch/2π. According to the integer value, if the carbon layer is rolled along a single symmetry axis, three types of carbon nanotubes may appear. When n = m, it takes an armchair (θ = 0°) conformation; when m = 0, it becomes zigzag type (θ = 30°); for n ≠ m, it is a chiral tube (θ = 0° and 30°). Armchair CNT is a mirror image match to zigzag and vice versa and therefore is considered a chiral. Different chirality angles and carbon nanotube diameters bring about different properties necessary for carbon nanotubes to be more effective and for emerging applications.
3. Carbon nanotube-based composites for electrocatalyst applications
The design and manufacture of electrocatalysts (electrodes for technical applications) are based on novel perceptions such as controlled surface roughness, atomic topography, defined catalytic centers, atomic redisposition, and phase changes during electrochemical reactions. One of the key features of electrocatalysis is the use of different crystal orientations on the electrode surface to generate different electrode dynamics. Electrocatalysis has many uses in technical electrochemical reactions. Some of them include organic electrosynthesis, electroforming, electrode sensors, fuel cells, and battery preparation. New developments related to electrocatalysis, such as spectroscopy or hybrid technology with new theoretical considerations, serve as the last point for the possible development of new predictions for the electrocatalytic reaction. Electrocatalysis is an interface method with a dynamic and permanent change of the electric-ion-conductor interface with practical technical applications.[Citation7]
3.1. Fuel cells
A fuel cell device generates electricity by electrochemical reactions instead of combustion. At present, there are five types of fuel cells based on the electrolyte they employ, which include alkaline, molten carbonate, phosphoric acid, solid oxide, and proton exchange membrane fuel cells. The efficiency of fuel cells (range from 40% to 80%) is better than the efficiency of an internal combustion engine, which is about 20%.[Citation8] To improve the efficiency of the fuel cell, a catalyst is needed. Noble metals such as Pt have good catalytic efficiency. However, they are expensive and are a major obstacle to the commercialization of fuel cells. Therefore, we need to reduce the consumption of costly metal catalysts and develop a new catalyst that can improve the electrode Oxidation-Reduction Reaction (ORR). On the other hand, the catalyst support can improve the performance of the catalyst in the fuel cell. Common catalyst supports include carbon, graphene, carbon nanotubes (CNT), and other forms of carbon allotropes. Nowadays, carbon nanotubes used in fuel cells are receiving widespread attention, because carbon nanotubes as a carrier can effectively improve the performance and utilization of the catalyst.[Citation9]
CNTs as support can effectively improve the performance of the catalysts of the fuel cell. The use of SWCNT catalyst support reduces the platinum loading from 0.125 mg Pt/m2 to 0.06 mg Pt/m2.[Citation10] Carbon nanotubes as a fuel cell carrier instead of carbon black as a platinum catalyst have better catalytic activity and higher current density.[Citation11] From the comparative study between Pt/MWCNTs, PtNi/MWCNTs, and PtRu/MWCNTs electrodes, it is found that PtRuNi/MWCNTs have better electrocatalytic activity.[Citation12] CNTs can also be a support for catalysts other than Pt, like Cu/CuxO[Citation13] and SnO2.[Citation14] The electrocatalytic activity of CNT as a carrier of Mo2C catalyst (16.7 wt% Mo) corresponds to the electrocatalytic activity of Pt catalyst (20 wt%).[Citation15] In addition, Pd3Co/MWCNT (2.53 mW/cm2) has better catalytic performance than Pd/MWCNT (1.64 mW/cm2) and Pt/C (1.20 mW/cm2) as fuel cell cathodes.[Citation16] Therefore, compared to the platinum-containing catalyst, non-noble metal catalysts with the CNT support for the electrode also have good catalytic effectivity.[Citation9] Ruthenium-decorated Pt nanoparticles on nitrogen-doped MWCNTs used for direct methanol fuel cells have high performance and high dispersibility.[Citation17] In this fuel cell, Pt nanoparticles charged in MWCNT also show higher electrode catalytic activity and better compatibility than the anode.[Citation18] The morphology and shape of carbon nanotubes have a profound effect on their electrocatalytic activity. Combined with Pt nanoparticles, the electrical performance of flower-shaped nanostructures is significantly higher than the conventional electrocatalytic performance of spherical nanoparticles.[Citation19] Zhang et al., introduced MWCTs into the Zeolitic Imidazolate Frameworks (ZIFs) to improve inter-particle conductivity of the Fe-C-N catalysts, by interconnecting the nanoparticles and providing electron-conducting highways. The result showed that ZIF-CNT has better electron conductivity, mass transfer, and power density in polymer-electrolyte membrane fuel cells.[Citation20]
Multi-Heteroatom-doped Defect-enriched Carbon Nanotubes (MHDCNTs), which combine bond polarization effects with lattice defects, have been developed to improve the activity of ORRs. The optimized MHDCNT-ORR cathode catalyst layer on the Anion Exchanged Membrane Fuel Cell (AEMFC) achieves a peak power density of 250 mW/cm2. This is about 70% of the power of AEMFC with traditional Pt/C catalysts.[Citation21] In most published studies on the electro-oxidation of formic acid for Direct Formic Acid Fuel Cells (DFAFC) using palladium catalysts at temperatures of 25-30 °C, the maximum cell output obtained ranges from 30–120 mW/mg Pd. After functionalization with ammonia solution, the specific battery power obtained in the Pd/MWCNTs catalyst on MWCNTs was 216 mW/mg Pd. This value is much higher than the Pd deposited on the original MWCNTs (52 mW/mg Pd).[Citation22] NiCo/Nitrogen-doped Carbon Nanotubes (NCNTs) exhibit excellent ORR performance in KOH, with a current density of 5.6 mA/cm2 and an onset potential of 0.98 V. The better electrochemical result and stability is because of the synergistic effect of NCNTs and active Ni/Co centers. Alkaline fuel cell performance with NiCo/NCNTs as the cathode catalyst is 65 mW/cm2, which is somewhat greater than the performance of commercial Pt/C as the cathode (60 mW/cm2).[Citation23] presents additional research works on fuel cells based on CNT electrocatalysts.
Table 1. Enhanced fuel cell by incorporating CNT in the electrocatalysts at the anode.
3.2. Batteries
Due to their excellent mechanical and electrical properties, CNT and CNT-based hybrid materials are excellent building blocks for various components in flexible batteries. With the recent emergence of portable and wearable electronics such as roll-up displays, smart wear, micro-sensors, medical implants, and artificial skins, flexible batteries are needed.[Citation30] Chen et al. synthesized the perovskite Lanthanum Nickel Oxide (LaNiO3)/nitrogen-doped CNT (LaNiO3/NCNT) hybrid catalyst. The catalyst was then coated on a flexible substrate made of carbon cloth as an air electrode for flexible Zinc-Air Batteries (ZAB). The ZAB constructed with this electrode catalyst showed stable discharge and charge potentials at various bending angles.[Citation31] Carbon ropes and copper wires respectively connect tiny RuO2/CNT cathode disk and the lithium anode disk in the portable lithium-air batteries. This strategy of "dividing the whole into several parts" reduces the stress on the electrode during the deformation process. As a result, even after 10,000 folding/stretching cycles, the discharge-charge curve remains almost unchanged.[Citation32]
The unique performance on electron transport, ion storage, architecture, and stability of CNTs are excellent congenital superiorities to fulfill the requirements of advanced lithium batteries. It is hopeful to overcome the battery obstacles such as cycle life, safety, cost, and environmental stability with the introduction of CNTs, and achieve high-performance lithium batteries with high energy and power density, fast ion transport, and long cycle life. CNTs in LiB can be used as a conductive material, active site, and as a support for active materials. It is found that the conductivity improvement in batteries relies on the architecture/morphology of CNTs. CNTs could exist in electrodes as discontinuous CNTs, binder-free CNT networks, and CNT Arrays (CNTAs).
The surface of CNTs can store Li+ and play a catalytic role for Li-O2 batteries. Both out-surface and the interior of CNTs can store lithium ions, thus increasing the reversible capacity. The comparison of free-standing CNTs has been studied and it is found that SWCNTs provide higher specific capacity, but with strong capacity fading, so MWCNTs are more widely used in rechargeable lithium batteries. When CNTs are used as active materials, their intrinsic characteristics, Specific Surface Area (SSA), and defects are key parameters in studying their electrochemical properties in batteries. The defects of CNTs can be considered as active sites for the absorption and storage of lithium ions. The method to introduce defects on the surface of CNTs mainly includes solvothermal process, microwave synthesis, thermal chemical reaction, and nitrogen (N) doping.[Citation33]
Nitrogen (4.32 Wt. %) doped CNT followed by KOH activation results in large SSA (2642 m2/g) and high inner pore volume (1.31 cm3/g) and shows higher specific capacity and better stability.[Citation34] Hao Wang et al. has compared the performance of CNTs and N doped CNTs. The results show that the specific capacity and the cycle stability of N-doped CNTs are high. This is because there are many nucleation sites and the discharge products are more evenly distributed.[Citation35] N-doped CNT electrodes have shown a high discharge capacity of 866 mA·h/g, about 1.5 times that of normal CNTs (590 mA·h/g).[Citation36] N-doped CNTs are potential electrode materials in future advanced Li-O2 batteries. The CNT fibril-based hybrid electrodes use the LiI redox mediator, and have a hierarchical nanoporous structure, providing effectively fast transport pathways for both reaction products and soluble catalysts.[Citation37] The introduction of microstructure and enlargement of SSA was attempted by introducing open holes on the surface of CNTs for Li-S batteries. These holes not only enhanced the dispersion of Li ions but also enabled more sulfur to fill the inside of the CNTs. Compared with the raw CNT–S composite, the composite with open holes exhibits a much higher capacity at various rates, with specific capacities of 1308, 985, 922 mA·h/g at 0.5, 1, and 2 C, respectively.[Citation38] By pyrolyzing propane with an inexpensive Fe-Al bimetallic catalyst under ambient pressure, electronically conductive MWCNT can be quickly manufactured. The effects of catalysts on the structure, surface, and defects of MWCNT were studied. MWCNTs made with an 8:2 Fe:Al ratio had a smooth surface, a large SSA, and fewer defects. The combination of MWCNTs with carbon black (super-P) improves the discharge capacity (160 mAh/g) of LiNi0.5Co0.2Mn0.3O2 cathode at a high rates, which was significantly better than those of the batteries with commercial Super P (158 mA⋅h/g).[Citation39] The new hierarchical nanostructures Co3O4 are applied to CNTs by Atomic Layer Deposition (ALD) (CNT @ Co3O4) and used as catalysts in Sodium-Air Batteries (SABs). CNT @ Co3O4 exhibits superior performance and longevity over mechanically mixed CNT/Co3O4 nanocomposites. A well-dispersed ALD-Co3O4 catalyst on the CNTs, which acts as a functionalized active center, enables fast electron transfer and high oxygen reduction/generation activity.[Citation40]
3.3. Electrochemical sensors
Compared with other instrumental analysis methods, the Electrochemical Sensor (ECS) has proven to be a cheap and simple analysis method, with significant detection, sensitivity, reproducibility, and easy miniaturization. Since CNTs have interesting electrochemical properties, which are contributed by the activity of the graphite center at the edge of the CNT ends, they can be used to construct CNT-based Electrochemical Sensors (CNTECS). CNTECS has a low detection limit, high sensitivity, and fast response behavior, which is due to signal amplification caused by large surface area, low overvoltage, and fast electrode dynamics.[Citation41]
Mohajeri et al. deposited platinum nanoparticles on the nanotubes by constant-potential and pulse-potential electrodeposition processes to improve the performance of the electrode. The catalytic activity of these two electrodes in the oxidation of methanol was determined by Cyclic Voltammetry (CV), demonstrating excellent electrode catalytic activity and poisoning tolerance produced by pulse deposition. The measurement performance of pulse-plated Pt/CNT electrodes for electrochemical sensing of dissolved sulfide ions was tested. A sensitivity of 0.632 μA/μM⋅cm2 and a detection limit of 0.26 μM have been achieved, demonstrating the improved capabilities of the sensor developed as a promising candidate for a variety of industrial and environmental applications.[Citation42]
A novel 3D open network based on Nitrogen-doped Graphene-Carbon Nanotubes connected to Gold Nanoparticles (NGRCNTs/AuNPs) for glucose determination by non-enzymatic method. NGRCNTs/AuNPs modified electrodes have been proven to perform well in glucose detection. The linear range is from 2 µM to 19.6 mM, the high sensitivity is 0.9824 µA/mM·cm2, and the low detection limit is 500 nM. Due to the excellent catalytic activity of AuNPs, the high performance of NGRCNTs/AuNPs-based sensors is adopted. AuNPs are well distributed on the NGRCNTs network. Due to the improved conductivity and large surface area, it proves to be the perfect support particle Fold. The obtained results indicate that the NGRCNTs/AuNPs mixture is expected to be sensitive and selective for the detection of glucose in sensor applications.[Citation43]
A metal-organic framework based on zirconium (UiO66 NH2) and MWCNT was prepared through a solvothermal one-pot reaction. The porous structure of the UiO66NH2 framework, unreacted amine groups, and the excellent conductivity of MWCNT make this composite material an ideal electrode for lead detection. After optimizing the MWCNT content and detection settings, the oxidation highest current is proportional to the lead concentration, ranging from 0.001–0.8 μmol/L, and the detection limit is as low as 0.5 nmol/L. More importantly, the MWCNTs@UiO 66NH2 maintains crystallinity even after 80 cycles and is immersed in aqueous solutions of different pH values, indicating that the sensor has a broad application prospect in actual samples.[Citation44]
Nitrogen-doped Carbon Quantum Dots (NCQD) loaded with Hexagonal Porous Copper Oxide (NCQD/HPCu2O) composite material was synthesized using a simple hydrothermal method. The load of NCQD in the NCQD/HPCu2O composite material plays a decisive role in the formation of the structure. The composite material is anchored with MWCNT using ultrasonic methods to construct a NCQD/HPCu2O/MWCNT hybrid composite material, which is used as an electrode material for effective electrochemical detection of Caffeic Acid (CA). Cyclic voltammetry, differential pulse voltammetry, and electrochemical impedance spectroscopy were used to study the detection behavior. Therefore, the developed NCQD/HPCu2O/MWCNT/GCE sensor has a detection limit of 0.004 μM and excellent sensitivity of 31.85 μA/μM⋅cm2 for the detection of CA. Therefore, hybrid composites may be a promising electrode modifier for improving electrocatalytic applications.[Citation45]
Due to its excellent chemical inertness and excellent dielectric strength, Sulfur Hexafluoride (SF6) is commonly used as an arc-extinguishing agent in electrical insulators and Gas-Insulated Switchgear (GIS) for power systems. When insulation fails, decomposition of SF6 into SO2, H2S, SO2F2, CF4, and SOF2 results in. This could be attributed to the discharge-induced SF6 decomposition as well as the succeeding reactions with contaminants such as air or water vapor. Certain functional oxygen groups such as OH and COOH are deliberately modified on the surface of SWCNTs during the calculation to improve adsorption performance. Functional groups modify the wettability of the SWCNT surface, making it more hydrophilic and suitable for the adsorption of low molecular weight and polar compounds. They found that the sensitivity of carboxyl modified SWCNT to the following gases keeps to the order SO2 > SOF2 > SO2F2 > CF4; thereby, COOH-doped CNTs sensor is capable to estimate the insulation state of GIS.[Citation46] presents some of electrochemical sensors made of CNTs.
Table 2. CNT based catalyst on the working electrode of electrochemical sensors.
3.4. Organic synthesis
At room temperature and atmospheric pressure, ammonia is directly synthesized from water and N2 in an electrochemical flow cell (half-cell for NH3 synthesis) working in the gas phase. Iron on CNTs is used as the electrocatalyst in the half-cell. The formation rate of ammonia in the N2 flow is 2.2 × 10−3 g NH3/m2h, and it has a stable behavior for a reaction time of at least 60 hours at an applied potential of 2.0 V. This value is higher than the rate of ammonia production (Ru/C) by precious metals under similar reaction settings. In addition, the overall Faraday efficiency of hydrogen is 95.1%. The data also shows that the active sites in electrocatalytic NH3 synthesis may be related to specific carbon centers, which are designed at the interface between iron particles and CNTs and can activate N2, making it more reactive toward hydrogenation.[Citation53] Yang et al. reported a Metal-Organic Framework (MOF) template carbonization route to prepare a Co-based hybrid electrocatalyst with superior performance for both Hydrogen Evolution Reaction (HER) and Oxygen Evolution Reaction (OER) in alkaline solution. Starting from CNT inserted Co MOF, the Co-NC/CNT hybrid with N-doped carbon nano frame composed of hollow Co nanoparticles interlinked by CNTs. This unique 3D structure is shown to have highly exposed active sites and good conductivity. Most importantly, the Co-NC/CNT hybrid can act as bifunctional catalysts on both anode and cathode for electrochemical water splitting, to deliver a current density of 10 mA/cm2 under a cell voltage as low as 1.625 V.[Citation54] Bimetallic (Fe, Co) P nanoparticles are decorated in a carbon nanotube network (FCPCN) and synthesized through a simple and scalable spray drying and successive phosphating process. FCPCN hybrid has an excellent performance in HER, OER, and all water decomposition in acidic and alkaline media conditions. It possesses, Pt-like hydrogen-evolution reaction activity with an ultralow onset over-potential of 18 mV in acid; remarkably, it shows an ultra-small Tafel slope of 38 mV/dec in oxygen evolution reaction; being employed as both cathode and anode, this catalyst demonstrates the promising performance of overall water splitting with high long-term stability. This work shows a scalable and inexpensive synthesis strategy for the synthesis of base and multifunction transition metal catalysts with a unique nano-architecture and excellent catalytic performance for water splitting.[Citation55]
A composite of palladium, SWCNT, and MOF199 (Pd/SWCNTs@MOF199) was prepared by hydrothermal method and its application as an electrocatalyst with Carbon Paste Electrode (CPE) for hydrogen production was investigated. Compared with CPE and MOF199 modified CPE, the Pd/SWCNTs@MOF199 modified CPE for hydrogen production in aqueous solution shows better catalytic performance, such as the highest catalytic activity and the lowest onset potential.[Citation56] Converting carbon dioxide into useful chemicals is one of the methods of recycling waste. There are many ways to convert carbon dioxide into useful chemicals, fuels, and fuel precursors known as carbon monoxide. Carbon dioxide can also be converted into formic acid, formate, ethylene, ethanol, and methanol.[Citation57] Several researchers have reported carbon nanotube-based electrocatalysts for carbon dioxide reduction. As it can be seen in , Bismuth, ferrous, cobalt, and copper nanoparticles were loaded in to CNTs for CO2 reduction and results in different faradic efficiencies. Nitrogen and Phthalocyanine (Pc) doping with such metals and support on the carbon nanosheets (CNS) could be good mechanism to improve the reduction efficiency.
Table 3. Some CNT based electrocatalysts for CO2 reduction reactions.
4. Conclusions
Electrocatalysts are a type of catalyst that takes part in electrochemical processes and is a special type of catalyst that works on the electrode surfaces or as the electrode surface itself. The electrocatalyst aids in the transfer of electrons between the electrode and the reactants, and/or facilitates a chemical transformation that is defined by an overall half-reaction. Transition metals, noble metals, and their alloys are used as efficient electrocatalysts. CNTs have capacious attention as catalyst support because they have high electrical conductivity, a high surface area, a crystalline structure, and high electrochemical stability, which are important for the fuel cells, batteries, and sensors to have a high performance and durability. We summarize and discuss the application of CNTs in fuel cells, batteries, sensors, and organic synthesis methods. The focus is on the effect of CNTs on the above devices in which the ways are used to reduce the need for noble metals and to improve the performance of devices catalysts, as well as the interaction with catalyst and CNT support and the synthesis conditions of carbon nanotube supported catalyst. Research into the support of CNTs and catalyst performance has yielded several results, allowing us to better understand the impacts of CNTs in electrocatalysts. The microscopic transport mechanism in CNTs, however, has to be investigated further. Precise fabrication, mass production, integration at high volume are still the challenges and issues we face. The production cost CNTs are extremely high and researchers are encouraged to reduce this cost.
Disclosure statement
No potential conflict of interest was reported by the authors.
References
- Qiu, H.; Yang, J. Structure and Properties of Carbon Nanotubes, Elsevier Inc., 2017; 2. DOI: 10.1016/B978-0-323-41481-4.00002-2.
- Bepete, G.; Coleman, K. S. Carbon Nanotubes: Electronic Structure and Spectroscopy. Compr. Nanosci. Nanotechnol. 2019, 1–5, 205–218. DOI: 10.1016/B978-0-12-803581-8.11401-8.
- Das, R.; Das Tuhi, S. Carbon Nanotubes Synthesis. In Carbon Nanotubes for Clean Water – Book, 2018; Vol. 1, pp 27–84.
- Dervishi, E.; Li, Z.; Xu, Y.; Saini, V.; Biris, A. R.; Lupu, D.; Biris, A. S. Carbon Nanotubes: Synthesis, Properties, and Applications. In 21st Century Surface Science – A Handbook, 2009; Vol. 27, pp 107–125. DOI: 10.1080/02726350902775962.
- Venkataraman, A.; Amadi, E. V.; Chen, Y.; Papadopoulos, C. Carbon Nanotube Assembly and Integration for Applications. Nanoscale Res. Lett. 2019, 14. DOI: 10.1186/s11671-019-3046-3.
- Liu, C. H.; Liu, Y. Y.; Zhang, Y. H.; Wei, R. R.; Zhang, H. L. Tandem Extraction Strategy for Separation of Metallic and Semiconducting SWCNTs Using Condensed Benzenoid Molecules: Effects of Molecular Morphology and Solvent. Phys. Chem. Chem. Phys. 2009, 11, 7257–7267. DOI: 10.1039/b901517e.
- Zinola, C. F.; Martins, M. E.; Tejera, E. P.; Neves, N. P. Electrocatalysis: Fundamentals and Applications. Int. J. Electrochem. 2012, 2012, 1–2. DOI: 10.1155/2012/874687.
- Salameh, Z. Energy Storage. In Renewable Energy System Design, Elsevier, 2014; Vol. chapter 4, pp 201–298.
- Luo, C.; Xie, H.; Wang, Q.; Luo, G.; Liu, C. A Review of the Application and Performance of Carbon Nanotubes in Fuel Cells. J. Nanomater. 2015, 2015, 1–10. DOI: 10.1155/2015/560392.
- Jha, N. R.; Bekyarova, P.; Tian, E.; Wang, X.; Itkis, F.; Haddon, M. E.; Robert, C. Functionalized Single-Walled Carbon Nanotube-Based Fuel Cell Benchmarked against US DOE 2017 Technical Targets. Sci. Rep. 2013, 3, 1–7. DOI: 10.1038/srep02257.
- Girishkumar, G.; Rettker, M.; Underhile, R.; Binz, D.; Vinodgopal, K.; McGinn, P.; Kamat, P. Single-Wall Carbon Nanotube-Based Proton Exchange Membrane Assembly for Hydrogen Fuel Cells. Langmuir 2005, 21, 8487–8494. DOI: 10.1021/la051499j.
- Zhao, Y.; Fan, L.; Ren, J.; Hong, B. Electrodeposition of Pt-Ru and Pt-Ru-Ni Nanoclusters on Multi-Walled Carbon Nanotubes for Direct Methanol Fuel Cell. Int. J. Hydrogen Energy 2014, 39, 4544–4557. DOI: 10.1016/j.ijhydene.2013.12.202.
- Sheng, X.; Wouters, B.; Breugelmans, T.; Hubin, A.; Vankelecom, I. F. J.; Pescarmona, P. P. Cu/CuxO and Pt Nanoparticles Supported on Multi-Walled Carbon Nanotubes as Electrocatalysts for the Reduction of Nitrobenzene. Appl. Catal. B Environ. 2014, 147, 330–339. DOI: 10.1016/j.apcatb.2013.09.006.
- Mehdinia, A.; Ziaei, E.; Jabbari, A. Multi-Walled Carbon Nanotube/SnO2 Nanocomposite: A Novel Anode Material for Microbial Fuel Cells. Electrochim. Acta 2014, 130, 512–518. DOI: 10.1016/j.electacta.2014.03.011.
- Wang, C. T.; Chen, Y. M.; Qi, Z. Q.; Yang, Y. C. Carbon Nanotube Planted on Ni-Based Alloy in Microbial Fuel Cell. J. Nanomater. 2013, 2013, 1–5. vol. DOI: 10.1155/2013/435960.
- Banerjee, J.; Dutta, K.; Rana, D. Carbon Nanomaterials in Renewable Energy Production and Storage Applications. Emerging Nanostructured Materials for Energy and Environmental Science, 2019; pp 51–104. DOI: 10.1007/978-3-030-04474-9_2.
- Johansson, A. C.; Yang, R. B.; Haugshøj, K. B.; Larsen, J. V.; Christensen, L. H.; Thomsen, E. V. Ru-Decorated Pt Nanoparticles on N-Doped Multi-Walled Carbon Nanotubes by Atomic Layer Deposition for Direct Methanol Fuel Cells. Int. J. Hydrogen Energy 2013, 38, 11406–11414. DOI: 10.1016/j.ijhydene.2013.06.089.
- Mu, Y.; Liang, H.; Hu, J.; Jiang, L.; Wan, L. Pt–Co Supported on Single-Walled Carbon Nanotubes as an Anode Catalyst for Direct Methanol Fuel Cells. Electrochim. Acta 2005, 109, 7276–7280. DOI: 10.1021/jp0555448.
- Ghosh, S.; Raj, C. R. Facile in Situ Synthesis of Multiwall Carbon Nanotube Supported Flowerlike pt Nanostructures: An Efficient Electrocatalyst for Fuel Cell Application. J. Phys. Chem. C 2010, 114, 10843–10849. DOI: 10.1021/jp100551e.
- Zhang, C.; Wang, Y. ‐C.; An, B.; Huang, R.; Wang, C.; Zhou, Z.; Lin, W. Networking Pyrolyzed Zeolitic Imidazolate Frameworks by Carbon Nanotubes Improves Conductivity and Enhances Oxygen-Reduction Performance in Polymer-Electrolyte-Membrane Fuel Cells. Adv. Mater. 2017, 29, 1604556. DOI: 10.1002/adma.201604556.
- Van Pham, C.; Britton, B.; Böhm, T.; Holdcroft, S.; Thiele, S. Doped, Defect-Enriched Carbon Nanotubes as an Efficient Oxygen Reduction Catalyst for Anion Exchange Membrane Fuel Cells. Adv. Mater. Interfaces 2018, 5, 1800184–1800189. DOI: 10.1002/admi.201800184.
- Mazurkiewicz-Pawlicka, M.; Malolepszy, A.; Mikolajczuk-Zychora, A.; Mierzwa, B.; Borodzinski, A.; Stobinski, L. Applied Surface Science a Simple Method for Enhancing the Catalytic Activity of Pd Deposited on Carbon Nanotubes Used in Direct Formic Acid Fuel Cells. Appl. Surf. Sci. 2019, 476, 806–814. DOI: 10.1016/j.apsusc.2019.01.114.
- Hanif, S.; Iqbal, N.; Shi, X.; Noor, T.; Ali, G.; Kannan, A. M. NiCo e N-Doped Carbon Nanotubes Based Cathode Catalyst for Alkaline Membrane Fuel Cell. Renew. Energy 2020, 154, 508–516. DOI: 10.1016/j.renene.2020.03.060.
- Zhao, N.; Ma, Z.; Song, H.; Xie, Y.; Zhang, M. Electrochimica Acta Enhancement of Bioelectricity Generation by Synergistic Modi fi Cation of Vertical Carbon Nanotubes/Polypyrrole for the Carbon fi Bers Anode in Microbial Fuel Cell. Electrochim. Acta 2019, 296, 69–74. DOI: 10.1016/j.electacta.2018.11.039.
- Cai, T.; Huang, M.; Huang, Y.; Zheng, W. ScienceDirect Enhanced Performance of Microbial Fuel Cells by Electrospinning Carbon Nanofibers Hybrid Carbon Nanotubes Composite Anode. Int. J. Hydrogen Energy 2019, 44, 3088–3098. DOI: 10.1016/j.ijhydene.2018.11.205.
- Bagher, M.; Salarizadeh, P.; Sei, M.; Mohammad, S. Ni/NiO Coated on Multi-Walled Carbon Nanotubes as a Promising Electrode for Methanol Electro-Oxidation Reaction in Direct Methanol Fuel Cell. Solid State Sci. 2019, 97. DOI: 10.1016/j.solidstatesciences.2019.106012.
- Qiu, M.; Zhang, B.; Wu, H.; Cao, L.; He, X.; Li, Y.; Li, J.; Xu, M.; Jiang, Z. Preparation of Anion Exchange Membrane with Enhanced Conductivity and Alkaline Stability by Incorporating Ionic Liquid Modified Carbon Nanotubes. J. Membr. Sci. 2019, 573, 1–10. DOI: 10.1016/j.memsci.2018.11.070.
- Devrim, Y.; Arıca, E. D. ScienceDirect Multi-Walled Carbon Nanotubes Decorated by Platinum Catalyst for High Temperature PEM Fuel Cell. Int. J. Hydrogen Energy 2019, 44, 18951–18966. DOI: 10.1016/j.ijhydene.2019.01.051.
- Zhang, P.; Liu, J.; Qu, Y.; Zhang, J.; Zhong, Y.; Feng, Y. Enhanced Performance of Microbial Fuel Cell with a Bacteria/Multi-Walled Carbon Nanotube Hybrid Biofilm. J. Power Sources 2017, 361, 318–325. DOI: 10.1016/j.jpowsour.2017.06.069.
- Hou, R. G.; Qi, G. S.; Nakhanivej, K.; Liu, P.; Li, H.; Xia, F.; Park, B. Y.; Seok, H. Hybridization Design of Materials and Devices for Flexible Electrochemical Energy Storage. Energy Storage Mater 2019, 19, 212–241. DOI: 10.1016/j.ensm.2019.03.002.
- Fu, J.; Lee, D. U.; Hassan, F. M.; Yang, L.; Bai, Z.; Park, M. G.; Chen, Z. Flexible High-Energy Polymer-Electrolyte-Based Rechargeable Zinc-Air Batteries. Adv. Mater. 2015, 27, 5617–5622. DOI: 10.1002/adma.201502853.
- Liu, T. X.; Liu, J. J.; Chang, Q. C.; Yin, Z. W.; Yang, Y. B.; Zhang, X. Y.; Bo, X. Ultrathin, Lightweight, and Wearable Li-O2 Battery with High Robustness and Gravimetric/Volumetric Energy Density. Small 2017, 13, 1602952–1602956. DOI: 10.1002/smll.201602952.
- Yuan, W.; Zhang, Y.; Cheng, L.; Wu, H.; Zheng, L.; Zhao, D. The Applications of Carbon Nanotubes and Graphene in Advanced Rechargeable Lithium Batteries. J. Mater. Chem. A 2016, 4, 8932–8951. DOI: 10.1039/C6TA01546H.
- Yang, J.; Xie, J.; Zhou, X.; Zou, Y.; Tang, J.; Wang, S.; Chen, F.; Wang, L. Functionalized n-Doped Porous Carbon Nanofiber Webs for a Lithium-Sulfur Battery with High Capacity and Rate Performance. J. Phys. Chem. C 2014, 118, 1800–1807. DOI: 10.1021/jp410385s.
- Mi, R.; Liu, H.; Wang, H.; Wong, K.-W.; Mei, J.; Chen, Y.; Lau, W.-M.; Yan, H. Effects of Nitrogen-Doped Carbon Nanotubes on the Discharge Performance of Li-Air Batteries. Carbon N. Y. 2014, 67, 744–752. DOI: 10.1016/j.carbon.2013.10.066.
- Li, Y.; Wang, J.; Li, X.; Liu, J.; Geng, D.; Yang, J.; Li, R.; Sun, X. Nitrogen-Doped Carbon Nanotubes as Cathode for Lithium-Airbatteries. Electrochem. Commun. 2011, 13, 668–672. DOI: 10.1016/j.elecom.2011.04.004.
- Lim, H.-D.; Song, H.; Kim, J.; Gwon, H.; Bae, Y.; Park, K.-Y.; Hong, J.; Kim, H.; Kim, T.; Kim, Y. H.; Lepró.; et al. Superior Rechargeability and Efficiency of Lithium-Oxygen Batteries: Hierarchical Air Electrode Architecture Combined with a Soluble Catalyst. Angew. Chem. 2014, 126, 4007–4012. DOI: 10.1002/ange.201400711.
- Xiao, Z.; Yang, Z.; Nie, H.; Lu, Y.; Yang, K.; Huang, S. Porous Carbon Nanotubes Etched by Water Steam for High-Rate Large-Capacity Lithium-Sulfur Batteries. J. Mater. Chem. A 2014, 2, 8683–8689. DOI: 10.1039/C4TA00630E.
- Tian, F.; Zhong, S.; Nie, W.; Zeng, M.; Chen, B.; Liu, X. Multi-Walled Carbon Nanotubes Prepared with Low-Cost Fe-Al Bimetallic Catalysts for High-Rate Rechargeable Li-Ion Batteries. J. Solid State Electrochem. 2020, 24, 667–674. DOI: 10.1007/s10008-020-04502-8.
- Sun, Q.; Liu, J.; Li, X.; Wang, B.; Yadegari, H.; Lushington, A.; Banis, M. N.; Zhao, Y.; Xiao, W.; Chen, N.; et al. Atomic Layer Deposited Non-Noble Metal Oxide Catalyst for Sodium–Air Batteries: Tuning the Morphologies and Compositions of Discharge Product. Adv. Funct. Mater. 2017, 27, 1606662., DOI: 10.1002/adfm.201606662.
- Mazloum-Ardakani, M.; Sheikh-Mohseni, M. A. Carbon Nanotubes in Electrochemical Sensors. Carbon Nanotub. – Growth Appl. 2011. DOI: 10.5772/20604.
- Mohajeri, S.; Dolati, A.; Rezaie, S. S. Electrochemical Sensors Based on Functionalized Carbon Nanotubes Modified with Platinum Nanoparticles for the Detection of Sulfide Ions in Aqueous Media. J. Chem. Sci. 2019, 131. DOI: 10.1007/s12039-019-1595-8.
- Jeong, H.; Nguyen, D. M.; Lee, M. S.; Kim, H. G.; Ko, S. C.; Kwac, L. K. N-Doped Graphene-Carbon Nanotube Hybrid Networks Attaching with Gold Nanoparticles for Glucose Non-Enzymatic Sensor. Mater. Sci. Eng. C Mater. Biol. Appl. 2018, 90, 38–45. DOI: 10.1016/j.msec.2018.04.039.
- Sun, X.; Chen, Y.; Xie, Y.; Wang, L.; Wang, Y.; Hu, X. Preparation of a Chemically Stable Metal-Organic Framework and Multi-Walled Carbon Nanotube Composite as a High-Performance Electrocatalyst for the Detection of Lead. Analyst 2020, 145, 1833–1840. DOI: 10.1039/c9an02299f.
- Muthusankar, G.; Sethupathi, M.; Chen, S.-M.; Devi, R. K.; Vinoth, R.; Gopu, G.; Anandhan, N.; Sengottuvelan, N. N-Doped Carbon Quantum Dots @ Hexagonal Porous Copper Oxide Decorated Multiwall Carbon Nanotubes: A Hybrid Composite Material for an Efficient Ultra-Sensitive Determination of Caffeic Acid. Compos. Part B Eng. 2019, 174, 106973. DOI: 10.1016/j.compositesb.2019.106973.
- Zhang, X.; Cui, H.; Gui, Y.; Tang, J. Mechanism and Application of Carbon Nanotube Sensors in SF6 Decomposed Production Detection: A Review. Nanoscale Res. Lett. 2017, 12. DOI: 10.1186/s11671-017-1945-8.
- Luo, X.; Shi, W.; Yu, H.; Xie, Z.; Li, K.; Cui, Y. Wearable Carbon Nanotube-Based Biosensors on Gloves for Lactate. Wearable Carbon Nanotube-Based Biosensors on 2018, 18, 3398. DOI: 10.3390/s18103398.
- Zhao, K.; Veksha, A.; Ge, L.; Lisak, G. Near Real-Time Analysis of Para-Cresol in Wastewater via a Laccase-Carbon Nanotubebased Biosensor. ECSN 2020, 128699. DOI: 10.1016/j.chemosphere.2020.128699.
- Zhao, Y.; Zhang, J.; Wang, Y.; Chen, Z. A Highly Sensitive and Room Temperature CNTs/SnO2/CuO Sensor for H2S Gas Sensing Applications. 2020, 40. DOI: 10.1186/s11671-020-3265-7.
- Wang, C.; Chan, C. K. Carbon Nanotube – Based Electrodes for Detection of Low – Ppb Level Hexavalent Chromium Using Amperometry. ECS J. Solid State Sci. Technol. 2016, 5, 3026–3031. DOI: 10.1149/2.0051608jss.
- Khalaf, A. L.; Arasu, P. T.; Lim, H. N.; Paiman, S.; Yusof, N. A.; Mahdi, M. A.; Yaacob, M. H. Modified Plastic Optical Fiber with CNT and Graphene Oxide Nanostructured Coatings for Ethanol Liquid Sensing. Opt. Exp. 2017, 25, 196–204.
- Shabbir, S. A.; Shamaila, S.; Bokhari, A.; Sabah, A. Nonenzymatic Glucose Sensor with High Performance Electrodeposited Nickel/Copper/Carbon Nanotubes Nanocomposite Electrode. J. Phys. Chem. Solids 2018. DOI: 10.1016/j.jpcs.2018.04.015.
- Chen, S.; Perathoner, S.; Ampelli, C.; Mebrahtu, C.; Su, D.; Centi, G. Electrocatalytic Synthesis of Ammonia at Room Temperature and Atmospheric Pressure from Water and Nitrogen on a Carbon-Nanotube-Based Electrocatalyst. Angew. Chem. Int. Ed. Engl. 2017, 56, 2699–2703. DOI: 10.1002/anie.201609533.
- Yang, F.; Zhao, P.; Hua, X.; Luo, W.; Cheng, G.; Xing, W.; Chen, S. A Cobalt-Based Hybrid Electrocatalyst Derived from a Carbon Nanotube Inserted Metal-Organic Framework for Efficient Water-Splitting. J. Mater. Chem. A 2016, 4, 16057–16063. DOI: 10.1039/C6TA05829A.
- Yang, D.; Hou, W.; Lu, Y.; Wang, X.; Zhang, W.; Chen, Y. Scalable Synthesis of Bimetallic Phosphide Decorated in Carbon Nanotube Network as Multifunctional Electrocatalyst for Water Splitting. ACS Sustain. Chem. Eng. 2019, 7, 13031–13040. DOI: 10.1021/acssuschemeng.9b02142.
- Ghiamaty, Z.; Ghaffarinejad, A.; Faryadras, M.; Abdolmaleki, A.; Kazemi, H. Synthesis of Palladium–Carbon Nanotube–Metal Organic Framework Composite and Its Application as Electrocatalyst for Hydrogen Production. J. Nanostruct. Chem. 2016, 6, 299–308. DOI: 10.1007/s40097-016-0203-4.
- Viswanathan, B. In Electro-Catalytic Reduction of Carbon Dioxide, Elsevier B.V., 2013; pp 275–295. DOI: 10.1016/B978-0-444-53882-6.00011-5.
- Li, Q.; Zhang, X.; Zhou, X.; Li, Q.; Wang, H.; Yi, J.; Liu, Y.; Zhang, J. Simply and Effectively Electrodepositing Bi-MWCNT-COOH Composite on Cu Electrode for Efficient Electrocatalytic CO2 Reduction to Produce HCOOH. J. CO2 Util. 2020, 37, 106–112. DOI: 10.1016/j.jcou.2019.12.003.
- Zhang, X.; Fu, J.; Liu, Y.; Zhou, X.; Qiao, J. Bismuth Anchored on MWCNTs with Controlled Ultrafine Nanosize Enables High-Efficient Electrochemical Reduction of Carbon Dioxide to Formate Fuel. ACS Sustain. Chem. Eng. 2020, 6–11. DOI: 10.1021/acssuschemeng.0c00099.
- Latiff, N. M.; Fu, X.; Mohamed, D. K.; Veksha, A.; Handayani, M.; Lisak, G. Carbon Based Copper(II) Phthalocyanine Catalysts for Electrochemical CO2 Reduction: Effect of Carbon Support on Electrocatalytic Activity. Carbon N. Y. 2020, 168, 245–253. DOI: 10.1016/j.carbon.2020.06.066.
- Pan, F.; Zhao, H.; Deng, W.; Feng, X.; Li, Y. A Novel N,Fe-Decorated Carbon Nanotube/Carbon Nanosheet Architecture for Efficient CO2 Reduction. Electrochim. Acta 2018, DOI: 10.1016/j.electacta.2018.04.047.
- Jiang, K. B.; Akey, S.; Xia, A. J.; Hu, C.; Liang, Y.; Schaak, W.; Stavitski, D.; Nørskov, E.; Siahrostami, J. K.; Haotian, S. W. Highly Selective and Active CO2 Reduction Electro-Catalysts Based on Cobalt Phthalocyanine/Carbon Nanotube Hybrid Structures. Nat. Commun. 2017, 1–8. DOI: 10.1038/ncomms14675.
- Zhang, Z.; Yang, Y.; Li, W.; Zhang, W.; Liu, M.; Weng, Z.; Huo, S.; Zhang, J. Boosting Carbon Monoxide Production during CO2 Reduction Reaction via Cu-Sb2O3 Interface Cooperation. J. Colloid Interface Sci. 2021, 601, 661–668. DOI: 10.1016/j.jcis.2021.05.118.