Abstract
Both pre-gestational and gestational diabetes have an adverse impact on heart development, but little is known about the influence on the early stage of heart tube formation. Using early gastrulating chick embryos, we investigated the influence of high glucose on the process of heart tube formation, specifically during the primary heart field phase. We demonstrated that high-glucose exposure resulted in 3 types of heart tube malformation: 1) ventricular hypertrophy, 2) ventricular hypertrophy with dextrocardia and 3) ventricular hypertrophy and dextrocardia with the fusion anomaly of a bilateral primary heart tube. Next, we found that these malformation phenotypes of heart tubes might mainly originate from the migratory anomaly of gastrulating precardiac mesoderm cells rather than cell proliferation in the developmental process of bilateral primary heart field primordia. The treatment of rapamycin (RAPA), an autophagy inducer, led to a similar heart tube malformation phenotype as high glucose. Additionally, high-glucose exposure promoted the expression of the key autophagy protein LC3B in early chick tissue. Atg7 is strongly expressed in the fusion site of bilateral primary heart tubes. All of these data imply that autophagy could be involved in the process of high-glucose-induced malformation of the heart tube.
Introduction
Gestational diabetes is due to glucose intolerance during pregnancy and presents as high blood glucose levels at the beginning of pregnancy. The incidence of congenital malformations in diabetic pregnancy has been reported to be 2–5 times higher than in non-diabetic pregnancy.Citation1 Therefore, pregnant women with pre-existing diabetes mellitus or gestational diabetes are at high risk of carrying a fetus with congenital anomalies such as neural phocomelia, cardiac malformations, macrosomia and central nervous system malformations.Citation2 Macintosh et al. reported that the increased risk of congenital anomalies was predominantly for congenital heart disease (3.4 times higher risk) and anomalies of the nervous system (2.7 times higher risk) in their study.Citation3 The precise mechanism for the teratogenic effects of diabetes on a developing embryo remains unclear. Hyperglycemia is proposed to be the most important teratogen, causing an increase in reactive oxygen species (ROS) in tissuesCitation4 and particularly affecting cardiovascular formation. The proposed mechanisms for the high glucose-induced ROS increase might have nonenzymatic protein glycosylation,Citation5-7 enhanced mitochondrial electron transport chain flow,Citation8 auto-oxidation,Citation9 and changes in the redox potential. These defects include artery or pulmonary atresia, double-outlet of right ventricle, tetralogy of Fallot, and fetal cardiomyopathy.Citation10 However, the embryonic phenotypes and underlying mechanisms caused by maternal pre-gestational diabetes are not yet known. Furthermore, little is known about the cell migration and cellular changes of cardiac precursors underlying early heart tube formation in the presence of hyperglycemia. In this study, we attempted to answer these questions using an early chick embryo model because the chick embryo can be easily manipulated at the early embryonic stage.Citation11
The major chick embryonic heart contributors consist of the cardiac precursor in splanchnic mesoderm (primary and secondary heart field), cardiac neural crest and the pro-epicardium. Heart development is a very complicated process under the regulation of spatio-temporal gene expression. From the view of morphological alteration, it is chronologically composed of primary heart tube fusion, cardiac looping and accretion, cardiac septation and coronary vasculogenesis.Citation12 The primary heart field contributes to the major structures of the heart, including the atria and ventricles, while the secondary heart field gives rise to the cardiac outflow tracts.Citation13 The primary heart fields derive from lateral gastrulating precardiac cells, which migrate out of the anterior primitive streak at the gastrula stage and then migrate laterally and anteriorly to form bilateral primary heart fields.Citation14-16 Borgave et al. reported that there were sources of mesenchymal stem cells in the primary heart field of HH 4 chick embryo and that those mesenchymal stem cells were able to differentiate into various cell lineages, such as cardiomyocytes, in response to certain instructive signals from Hensens node.Citation17 If the embryological origin of heart development is abnormal, the risk of congenital heart disease must be higher. Ste´phane Zaffran, et al. also demonstrated the differences in the embryological origin of right and left ventricular myocardium, which has important implications for congenital heart disease.Citation18
Many spatiotemporally expressed genes are involved in heart development. Therefore, it is not easy to assign a particular gene for cardiac specification or a mesoderm and endoderm inducer, which in turn enhances cardiac mesoderm formation.Citation19 Fibroblast growth factor (FGF) signaling is required for the migration of pro-cardiac mesoderm cells in Drosophila and chicks.Citation15,20 Generally, cardiac specification occurs when the cells reach the anterior lateral plate mesoderm. As an important inducer of myocardium, the anterior endoderm underlies the anterior lateral plate mesoderm. In the chick embryo, Bone morphogenetic protein 2 (BMP2) plays an important role in heart-inducing activity and is derived from anterior endoderm. Moreover, Nkx-2.5, GATA factors, myocardin, and Tbx20 are well-known transcription factors that characterize and induce cardiogenic differentiation.Citation19 Therefore, Nkx-2.5 and BMP2 could be considered common cardiogenic factors.
Under physiological conditions, autophagy is considered to be the process by which energy is supplied for embryonic development through the lysosomal degradation of cellular contents.Citation21 Furthermore, autophagy also acts as a housekeeper by preventing protein accumulation in cells and removing dead or damaged organelles.Citation21 One could imagine that autophagy plays vital roles in vertebrate development. Autophagy-related genes (Atg), Atg5, Atg7, and Atg8 (LC3) participate in the various stages of the autophagy process.Citation22 Mice with an Atg5 or Atg7 mutation can survive the embryonic period but die soon after birth.Citation23,24 Three-Methyladenine (3-MA) has been widely employed as a specific inhibitor of PI3K activity to block the initial phase of the autophagic process, while rapamycin (RAPA) is reported to be the best pharmacological inducer of autophagy.
In this study, we first demonstrated the variety of phenotypes of malformed chick heart tubes induced by high glucose exposure. Next, we found that high glucose treatment could affect the migration of gastrulating precardiac cells rather than their proliferation, and we reasoned that the change might be mediated by the autophagy process, which was implied by the experiment using autophagic inducer or inhibitor. The expression of heart tube formation-related genes in embryos treated with high glucose or autophagic manipulation was determined to explore the underlying mechanism.
Materials and Methods
Avian embryos and manipulation
Fertilized chick eggs were obtained from the Avian Farm of the South China Agriculture University. The eggs were incubated until the required HH stageCitation25 in a humidified incubator (Yiheng Instrument, Shanghai, China) at 38°C and 70% humidity. The embryo culture method was chosen according to experimental requirements, i.e., EC cultureCitation26 was employed for gastrula chick embryo, while whole egg incubation was used for late stage embryos. For the high glucose-treated embryos, the chick embryos were treated with either 50 mM glucose or mannitol (control). RAPA inhibits mTOR by binding to RPTOR, thus inducing autophagy, but only provides partial inhibition;Citation27 3-MA is a commonly reagent for inhibiting autophagyCitation21 and it is commonly used to inhibit starvation- or RAPA - induced autophagyCitation28; We have demonstrated that they could induce disturbance of autophagy of early chick embryo.Citation30 Chloroquine (CQ) could prevent the fusion of autophagosomes with lysosomes, result in an accumulation of autophagosomes and inhibition of autophagy.Citation29 For the RAPA, 3-MA or CQ treated embryos, the chick embryos were treated with either 40 nM RAPA (LC Labs, USA), 5 mM 3-MA (Sigma, USA) or 10 μM CQ (Sigma, USA).Citation27 Briefly, high glucose, RAPA, 3-MA or 10 μM CQ was directly applied to one side of the gastrula-stage embryos, while the other side was exposed to mannitol, DMSO or PBS as a control, respectively. The concentration level of blood glucose in the treated embryos was equivalent up to the levels seen in human diabetes. The change of glucose concentration around the embryo was not too much within 7 d after the glucose injection.Citation31 The treated embryos were then incubated overnight before they were fixed with 4% paraformaldehyde for analysis of morphology and gene expression.
Chick embryo electroporation and transplantation
HH3 chick embryos in EC culture were transfected by electroporation (BTX-ECM399) using a home-made 2-wire parallel electrode made of platinum at 10 V voltage + 2 pulses, as previously describedCitation15; then the transfected embryos were continuously incubated at 37°C with humidity until the required stage. The transplantation approach used was previously described.Citation15 In brief, orthotropic transplantation of the primitive streak was performed from transfected donor embryos to un-transfected, same-stage (HH3+) host embryos at the same position.
Cell trace with diI
Carbocyanine dye 1,1V-dioctadecyl-3,3,3V,3V-tetramethyl indocarbocyanine perchlorate (DiI, Molecular Probes, Inc.) was used to label small groups of primitive streak cells. A 2.5% stock solution of DiI was diluted in ethanol 1:10 in 0.3 M sucrose and was injected into the anterior primitive streak of HH3 chick embryo by air pressure through a micropipette, which was pulled from a 1 mm glass capillary in a vertical micropipette puller (WD-2, Chengdu Instrument Company). In general, each labeled tissue in the anterior primitive streak contained approximately 10–30 cells.
Primary cardiomyocyte culture
The cardiomyocytes for primary culture was obtained from the hearts of 11-day old chick embryos.Citation32 Approximately, 95% of the cells in the primary culture were determined to be cardiomyocytes, which were used for immunofluorescent staining to show the presence of myosin heavy chain (MF20 antibody, data not shown). The cells were maintained in 6-well plates (1×10Citation6 cells/well) in DMEM plus 10% FBS at 37oC and 5% CO2. Each treatment was performed in triplicates.
In situ hybridization
Whole-mount in situ hybridization of chick embryos was performed according to a standard in situ hybridization protocol.Citation33 Briefly, Digoxigenin-labeled probes were synthesized for FGF8,Citation15 BMP2Citation34 and GATA5.Citation35 The Tbx5 and Tbx20 templates for in situ hybridization probes were generated by PCR amplification and labeled probes were transcribed with the T7 polymerase and dioxigenin-UTP (Roche). Primer sequences are as follows: Tbx5: AATTAACCCTCACTAAAGGAACTTCACCAGCGGAAGAGG and TAATACGACTCACTATAGGCCAATGAGTGCTCCATCCGT; Tbx20: AATTAACCCTCACTAAAGGGACCATCAAGCCTCTGGAGCAA and TAATACGACTCACTATAGGGATCGGGCGTAGGAATGCTTTT. The whole-mount stained embryos were photographed and then frozen sections were prepared on a cryostat microtome (Leica CM1900) at a thickness of 15–20 μm.
Immunofluorescent staining
Chick embryos were harvested after overnight incubation and fixed in 4% PFA overnight at 4°C. Whole-mount embryo immunostaining was performed using the following antibodies: MF-20 (1:200, DSHB, USA), HNK-1 (1:200, Sigma, USA), Atg7 (Sigma, 1:200, USA) and LC3B (1:100, Cell Signaling, USA). Briefly, the fixed embryos were then incubated with Atg7, LC3B, MF-20 or HNK-1 antibody at 4°C overnight on a shaker. Following extensive washing, the embryos were incubated with either anti-mouse IgM conjugated to Alexa Fluor 555 or anti-rabbit IgG conjugated to Alexa Fluor 488 overnight at 4°C on a rocker. All the embryos were later counterstained with DAPI (1:1000, Invitrogen) at room temperature for 1 hour.
RNA isolation and RT-PCR
Total RNA was isolated from HH8 chick primary heart field or HH11 chick heart tube tissues using a Trizol kit (Invitrogen, USA) according to the manufacturer's instructions. First-strand cDNA was synthesized to a final volume of 25 μl using SuperScript RIII first-strand (Invitrogen, USA). Following reverse transcription, PCR amplification of the cDNA was performed as described previously.Citation36,37 The sets of primers used for RT-PCR are provided in the Figure S6. The PCR reactions were performed in a Bio-Rad S1000TM Thermal cycler (Bio-Rad, USA). The final reaction volume was 50 μl composed of 1 μl of first-strand cDNA, 25 μM forward primer, 25 μM reverse primer, 10 μl PrimeSTARTM Buffer (Mg2+ plus), 4μl dNTPs Mixture (TaKaRa, Japan), 0.5 μl PrimeSTARTM HS DNA Polymerase (2.5U/μl TaKaRa, Japan), and RNase-free water. cDNA was amplified for 30 cycles. One round of amplification was performed at 94°C for 30 s, 30 s at 58°C, and 30 s at 72°C. The PCR products (20 μl) were resolved using 1% agarose gels (Biowest, Spain) in 1× TAE buffer (0.04 M Trisacetate and 0.001 M EDTA) and 10,000x GeneGreen Nucleic Acid Dye (TIANGEN, China) solution. The resolved products were visualized using a transilluminator (SYNGENE, UK), and photographs were captured using a computer-assisted gel documentation system (SYNGENE).
Still and time-lapse photography
Following immunofluorescent staining or in situ hybridization, the whole-mount embryos were photographed using a stereo-fluorescent microscope (Olympus MVX10) and associated Olympus software package Image-Pro Plus 7.0. The embryos were sectioned into 15 μm-thick slices using a cryostat microtome (Leica CM1900), and then the sections were photographed using an epi-fluorescent microscope (Olympus LX51, Leica DM 4000B) with the CN4000 FISH Olympus software package. To obtain the time-lapse photography, we incubated the GFP-transfected embryo in a home-made incubation chamber, which was heated to 37°C by blowing through humidified air with an Air Therm heating unit (World Precision Instruments) mounted on the stage of an inverted microscope (Nikon Eclipse TI-U). Fluorescent and bright-field images were continuously obtained every 3 min for 10 hours using a cooled CCD camera (Nikon DS-Fi1c). We processed the resulting images to generate movies using the VideoMach program.
Data analysis
Statistical analysis for all the experimental data generated was performed using a SPSS 13.0 statistical package program for windows. The data were presented as mean ± SD. Statistical significance were determined using paired T pest, independent samples T test or one-way analysis of variance (ANOVA). P<0.05 was considered to be significant.
Results
High glucose resulted in various malformations of heart tubes in chick embryos
As in our previous report,Citation38 cardiac hypertrophy was an obvious phenotype following high glucose treatment. To further investigate the pathophysiological mechanism, we carefully checked the early developmental stage of heart tube formation using chick embryos treated with high glucose. Specifically, HH0 chick embryos were incubated in EC culture until they reached HH11, as graphically described in . Both VMHC and MF-20 are particularly expressed in developing heart tubes in early stage chick embryos, as shown in ; thus, VMHC in situ hybridization and MF-20 immunofluorescence staining were performed to highlight the developing heart tubes in the presence/absence of high glucose (). The exposure of high glucose resulted in 3 types of heart tube malformations when compared to the control in the same developmental stage. The first phenotype is hypertrophy (), in which the VMHC-labeled heart tube appeared to be larger than the control (). The walls of heart tubes in the presence of high glucose () became much thicker than the control heart tubes () when transverse sections were compared. The second of the high glucose-induced phenotypes is dextrocardia (). The C-loop stage heart tube should have bended to the left like the control heart tube (), but the MF-20-labeled heart tube remained on the right side in the presence of high glucose. The third phenotype induced by high glucose in the heart tube stage was the fusion anomaly of heart tubes, which is shown in, where the atrium and ventricle could be simultaneously observed in its corresponding transverse section due to its abnormal morphology. The highest morbidity rate observed for these phenotypes was found in the heart tubes with hypertrophy (; 5% in control group and 90% in HG group; N=20 embryos respectively); hypertrophy with dextrocardia (; 5% in control group and 40% in HG group; N=20 embryos respectively) ranked second, and the combination of heart tube formation failure and the aforementioned phenotypes occurred least often (; 0% in control group and 25% in HG group; N=20 embryos respectively). We also used additional cardiac progenitor markers to show that malformation of heart tubes occurred in the high-glucose-treated embryos (Fig. S1). The GATA5,Citation39,40 Tbx5 and Tbx20Citation41 are expressed the primary heart tube. The results showed that the hypertrophy and dextrocardia occurred in the high-glucose-treated embryos (Fig. S1A–F1). Then, the extent of VMHC and Tbx5 expression were validated by semi-quantitative RT-PCR analysis after treatment with either mannitol or high glucose, the results showed that both of VMHC and Tbx5 were increased after high glucose exposed (Fig. S1G–G1). Because HNK-1 is distinctly expressed in developing heart tube. The results also showed that malformation of HNK1-labeled heart tubes occurred in the presence of high glucose (Fig. S1H, H1–3) compared to the control (Fig. 1I, I1–3).
Figure 1. The phenotypes of abnormal heart tube formation following treatment with high glucose. (A) A cartoon illustrating that the fertilized chick eggs were incubated in the presence of mannitol (control) or 50 mM glucose (high glucose) until the embryos reached HH11 stage. (B-B2) VMHC in situ hybridization was performed in HH11 control embryos (B). A high-magnification image focused on the developing heart tube (B1). The corresponding level of transverse section was indicated by the white dotted line in B1 (B2). (C-C2) MF-20 fluorescence immunostaining was performed in HH11 control embryos. C and C1 are bright-field and fluorescence images, respectively. C2 is the high-magnification image focused on the developing heart tube. (D-D2) VMHC in situ hybridization was performed in HH11 high-glucose-treated embryos (D). D1 is the high-magnification image focused on the developing heart tube. D2 is the transverse section at the level indicated by the white dotted line in D1. (E-E2) MF-20 fluorescence immunostaining was performed in HH11 high-glucose-treated embryos. E and E1 are bright-field and fluorescence images, respectively. E2 is the high-magnification image focused on the developing heart tube. (F-F3) MF-20 fluorescence immunostaining was performed in HH11 high-glucose-treated embryos. F and F1 are bright-field and fluorescence images, respectively. F2 is the high-magnification image focused on the developing heart tube. F3 is the transverse section at the level indicated by the white dotted line in F2. (G) Bar chart showing the frequency of heart tube hypertrophy. (H) Bar chart showing the frequency of dextrocardia. (I) Bar chart showing the frequency of heart tube fusion anomaly. Abbreviations: Ctr, control and HG, high glucose. Scale bars = 500 μm in B, C-C1, D-D1, E-E1 and F-F1; 100 μm in B1, C2, D1, E2, F2; 100 μm in B2, D2, F3.
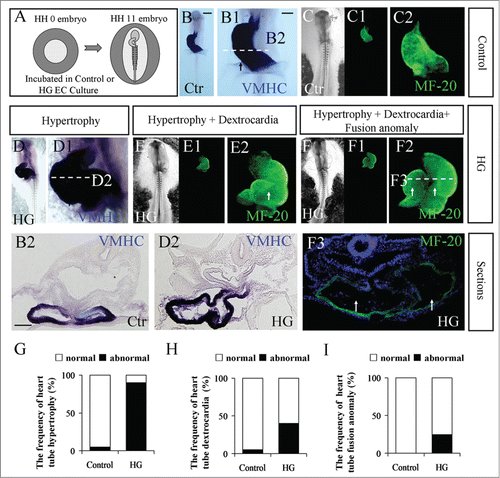
Additionally, the average size of the cardiomyocytes (MF20+ cells) was calculated by dividing the total area occupied of the cells over the total cell number in the histological sections. Using this simple method, we established that the cardiomyocytes in heart tube increased in size after high glucose treatment (Control=243 ± 19 μm, N=6, high glucose=274 ± 23 μm, N=7, p<0.05; Fig. S2). Then we detected some hypertrophy signaling moleculars of HH11 chick heart tube: IGF-1, IGF-1R,Citation42 FGF2Citation43 and Ang2.Citation44 The RT-PCR results suggested that the levels of Ang2, FGF2 and IGF-1R were not changed after high glucose treated compared with control group embryos. Only IGF-1 was inhibited (p<0.01; Fig. S3A–E). In our previous study, we found that excess ROS induced by AAPH could lead to myocardial hypertrophy during heart development.Citation45 We proved that high glucose could increase accumulation of ROS.Citation38 So we also detected SOD1, SOD2 and Gpx, we found the Gpx level was increased in both high glucose and RAPA treated embryos (p<0.001; Fig. 3A and F–H). These results suggested high glucose could increase the ROS level and then result in cell hypertrophy.
High glucose promoted the cardiac progenitor cell migration in gastrula embryo
The heart tube derives from bilateral primary heart fields, which are located in the lateral plate mesoderm. Moreover, the mesoderm cells of the bilateral primary heart fields originate from the anterior primitive streak of gastrula embryos. The cells migrate bilaterally and equally out of the primitive streak, as our previous reports demonstrate.Citation14,15 Utilizing this strategy, GFP+ anterior primitive streaks were grafted into the identical position in untransfected HH3 chick embryos. Meanwhile, high glucose was added to one side of the embryos, with the other side acting as the control. The delamination of mesoderm cells from the transplanted GFP+ anterior primitive streak is bilaterally symmetrical in normal developing embryos. The exposure of embryos on one side to experimental factors and the other side to control media has proven to be particularly effective in our previous study.Citation46 This method was used to avoid the artificial error that arises from varying rates of development in individual embryos. From the results, the majority of GFP+ cells appeared on the side of high glucose treatment compared to the control side (, A1-C1; N=9/12 HH5 and HH8 embryos). This tendency was increasingly obvious as development progressed (). More GFP+ mesoderm cells could be observed on the high glucose-treated side in the corresponding transverse sections (; N=13, P<0.01). We also recorded this process by time-lapse photography, as shown in Movie S1 (supplementary data). To confirm the aforementioned observation, we repeated the experiment with DiI labeling in the anterior primitive streak followed by analysis of the migratory trajectory of DiI-labeled cells (Fig. S4; N=4/4 in control embryos and N=7/10 in half sides exposed to HG embryos). What we found with the trace of DiI-labeled cells in the presence of high glucose was in accordance with the experimental observation in GFP+ anterior primitive streak graft, i.e., high-glucose treatment promoted mesoderm cell migration toward to the region of primary heart tube formation.
Figure 2. The treatment of high glucose sped up mesoderm cell migration during gastrulation. A piece of GFP+ anterior primitive streak tissue was transplanted into the same position in un-transfected HH3+ host embryos, and the embryos were further cultured in high glucose (left-side embryo) and mannitol (right-side embryo) (see the detail in Material and Method) for 20 hours. (A–C) Fluorescence images were taken at incubation 0 hour (A), 10 hour (B) and 20 hour (C), respectively. (D) High-magnification image indicated by the white dotted line square in C. (A1-C1) The merge image of bright-field and fluorescence at incubation 0 hour (A1), 10 hour (B1) and 20 hour (C1), respectively. (D1) High-magnification image indicated by the white dotted line square in C1. (E) The sketch illustrates that there are more red dotted lines on the high-glucose side (left), indicating that high glucose promoted mesoderm cell migration. (F-G2) Transverse sections of GFP-positive cells (F and G), BrdU staining (F1 and G1) and the merge of GFP and BrdU (F2 and G2) of HH5 or HH8 embryo. (H) Bar chart showing the comparison of GFP+ mesoderm cell number between control and high-glucose-treated sides (paired T pest). (I and J) Bar chart showing the comparison of BrdU+ mesoderm cell number between control and high-glucose-treated sides of HH5 or HH8 embryo (paired T pest). (K-K2) RT-PCR showing the expression of RhoA and Cyclin D1 after treatment with high glucose. The RT-PCR result was the representative of 4 independent experiments (independent samples T test). Abbreviations: Ctr, control and HG, high glucose. Scale bars = 500 μm in A-C, A1-C1; 200 μm in D-D1; 100 μm in F-F2 and 100 μm in G-G2.
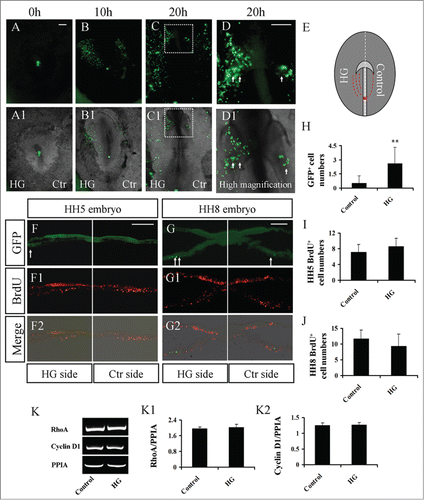
One possibility is that the increase in GFP+ mesoderm cells in unilateral embryos might be due to enhanced cell proliferation by the treatment of high glucose. Therefore, a BrdU incorporation experiment was performed, and the results showed that there was no significant difference between control and high glucose-treated groups in HH5 or HH8 embryos ( and I; N=7; and J; N = 6; P > 0.05). Then, the extent of Cyclin D1 expression was validated by semi-quantitative RT-PCR analysis after treatment with either mannitol or high glucose (). These data suggest that high glucose had no effect on the G1-S transition. These results suggest that the principal reason for the increased number of GFP mesoderm cells in the high-glucose-treated side was the promotion of mesoderm cell migration. RhoA, a member of the small GTPase family, is thought to mediate the actin cytoskeleton in the formation of stress fibers. However, we did not find that RhoA expression was affected by high-glucose treatment ().
High glucose promoted autophagy during heart tube formation
Adastra KL, et al. demonstrated that the cells responded to hyperglycemic environment by increasing activation of autophagy.Citation47 We compared the expression of LC3B in the primary culture of early chick embryos in the presence/absence of high glucose (, A1-B1). The results showed that there was little LC3B expression without the exposure to high glucose in the control primary culture (). However, LC3B expression was dramatically enhanced by exposure to high glucose (; N>3) in comparison to the control. We also checked Atg7 expression around the heart tube in HH8 and HH10 chick embryos (). At stage HH8, Atg7 was strongly expressed in medial cardiac crescents (the connection between 2 bilateral primary heart fields) (), appeared in splanchnic lateral mesoderm (weakly) and endoderm (strongly) on the transverse sections indicated, as by write arrows. When the embryo reaches stage HH10, the primordia of primary heart tubes have formed. The region of strong expression of Atg7 is shown in the high-magnification image of. In the corresponding transverse section, Atg7 was present in the fusion site of bilateral heart tubes (), as indicated by arrows. The splanchnic lateral mesoderm also weakly expressed Atg7 (red arrow in). Since the endoderm and splanchnic lateral mesoderm play important role in the normal heart tube development.Citation48 All of these data suggested that the Atg7-mediated autophagy is involved in the normal heart tube formation and high glucose could promote the autophagy.
Figure 3. Exposure of high glucose promoted autophagy in gastrula chick embryos. (A and B) The LC3B fluorescence immunostaining was performed in primary cell culture from either control (A-A1) or RAPA-treated (B-B1) embryos. A1 and B1 are merged images with DAPI staining, respectively. (C and D) Atg7 fluorescence immunostaining was performed in HH8 (C) and HH10 (D) chick embryos. High-magnification images at the cranial region are on the right corner of C and D. (C1-C2) Transverse sections at the level indicated by the white dotted line in C. C1 is Atg7 fluorescence staining only, and C2 is the merger of DAPI and C1. (D1-D2) Transverse sections at the level indicated by the white dotted line in D. D1 is Atg7 fluorescence staining only, and D2 is the merger of DAPI and D1. Abbreviations: HG, high glucose. Scale bars = 10 μm in A-B1; 500 μm in C and D; 100 μm in C1-C2; 50 μm in D1-D2.
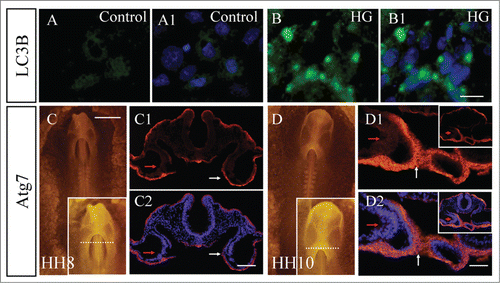
The disturbance of autophagy affected the cell migration of cardiac precursors during heart tube formation
Then, we exposed gastrulating chick embryos to RAPA, an activator of autophagy, and found a malformed heart tube phenotype () similar to that occurring with high-glucose treatment (). The RT-PCR results also suggested that the levels of Ang2, FGF2, IGF-1R, SOD1 and SOD2 were not changed after RAPA treated compared with control group embryos. But IGF-1 expression was inhibited and Gpx level was increased (p<0.01 and p<0.05 respectively; Fig. S3A–H). The results were also similar to that occurring with high-glucose treatment.
Figure 4. Interference of autophagy obstructed chick heart tube fusion. (A-A1) MF-20 fluorescence immunostaining was performed in control and RAPA-treated embryos. A is the image of bright-field, and A1 is fluorescence immunostaining. A high-magnification image focused on a heart tube is in the right corner of A1. (B) A cartoon illustrating that autophagy was graphically activated by RAPA or inhibited by 3-MA in gastrula chick embryos. (C–H) The combination of GFP+ anterior primitive streak grafts with the exposure of either RAPA or 3-MA in gastrula chick embryos. (C–E) Fluorescence images were taken at hour 0 (C), hour 12 (B) and hour 20 (C) of incubation after GFP+ anterior primitive streak transplantation. The left sides of embryos were exposed to 40 nM RAPA, while the right sides were untreated (control). (C1-E1) The merged images of bright-field and A-C, respectively. (F-H) Fluorescence images were taken at hour 0 (C), hour 12 (B) and hour 20 (C) of incubation after GFP+ anterior primitive streak transplantation. The left sides of embryos were exposed to 5 mM 3-MA, while the right sides were untreated (control). (F1-H1) The merged images of bright-field and F-H, respectively. (I) The sketch illustrates that there are less red dotted lines on the 3-MA+HG-treated side (left) in comparison to the PBS+HG-treated side of embryos. (J-L) Fluorescence images were taken at hour 0 (C), hour 12 (B) and hour 20 (C) of incubation after DiI-labeled primitive streak transplantation. The left sides of embryos were exposed to 3-MA+HG, while the right sides were to PBS+HG. (J1-L1) The merged images of bright-field and F-H, respectively. (M-P) Fluorescence images were taken at hour 0 (C), hour 12 (B) and hour 20 (C) of incubation after DiI-labeled primitive streak transplantation. The left sides of embryos were exposed to CQ+HG, while the right sides were to PBS+HG. (N1-P1) The merged images of bright-field and N-P, respectively. Abbreviations: Ctr, control and HG, high glucose. Scale bars =500 μm in A-A1, C-K1, L-L1 and N-P1.
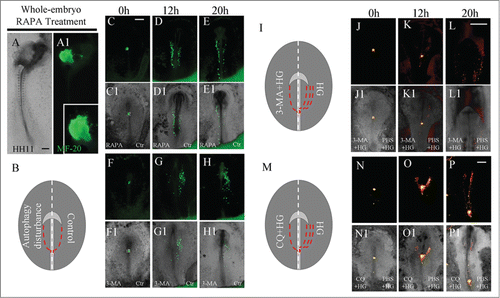
Next, the same strategy as the above experiment was employed to disturb one side of early chick embryos with an autophagy activator (RAPA) or inhibitor (3-MA;). Again, we performed the xenograft of GFP+ anterior primitive streak before incubating these embryos with either RAPA or 3-MA (). There were more migratory GFP+ mesoderm cells in the RAPA-treated sides in comparison to the control sides of embryos after 12- or 20-hour incubations (, C1-E1; N=5/8 embryos). Conversely, fewer migratory GFP+ mesoderm cells were found in the 3-MA-treated sides in comparison to the control sides of embryos after 12- and 20-hour incubations (, F1-H1; N=4/6 embryos). To confirm that the autophagy is involved in high glucose-induced the abnormal migration of cardiac progenitor cells, we applied 3-MA+HG directly to one side of the HH3 chick embryos, while the another side was exposed to PBS+HG as a control (). There were less migratory DiI+ mesoderm cells in the 3-MA+HG-treated sides in comparison to the PBS+HG-treated sides of embryos after 12- or 20-hour incubations (, J1-L1; N=8/12 embryos). Furthermore, we employed another autophogy inhibitor-CQ to confirm the 3-MA+HG-treated results. There were also less migratory DiI+ mesoderm cells in the CQ+HG-treated sides in comparison to the PBS+HG-treated sides of embryos after 12- or 20-hour incubations (, N1-P1; N=5/6 embryos). These experimental evidences clearly suggested that autophagy participates in the cell migration of cardiac precursors toward to the site of heart tube formation.
High glucose impact on cardiogenesis-related gene expression during heart tube formation
The expression of the cardiogenesis-related genes Nkx-2.5, BMP2, GATA4 and GATA5 was determined on the side of embryos treated with high glucose using in situ hybridization or RT-PCR. BMP2 expression was weaker on the high glucose-treated sides of embryos compared to the control sides (, A1–2, and B; N=4/6 embryos). A similar phenotype was found for the expression of GATA5. High glucose could reduce the expression of GATA5, which was reflected by weaker expression of GATA5 on the high-glucose-treated side of embryos (, C1–2, D; N=7/10 embryos). Additionally, RT-PCR data showed that BMP2, Nkx-2.5 and GATA4 expression was also reduced following treatment with high glucose (). FGF8 expression did not appear to be affected by high-glucose treatment (Fig. S5; N=5/6 embryos). Furthermore, GATA5 expression was also detected using in situ hybridization after the activation of autophagy by RAPA (). In the presence of RAPA, GATA5 expression was reduced on the RAPA-treated side of embryos (, F1–2, G; N=10/12 embryos), which is in accordance with the response to high-glucose treatment.
Figure 5. The exposure of embryos to high glucose or RAPA blocked gene expression in progenitors of the heart tube. (A and B): BMP2 in situ hybridization was performed in embryos exposed on one side to high glucose (A). A1 and A2 are high-magnification images from the sites indicated by dotted squares in A. (B) is the transverse section at the level indicated by the dotted line in A. (C and D) GATA5 in situ hybridization was performed in embryos exposed on one side to high glucose (C). C1 and C2 are high-magnification images from the sites indicated by dotted squares in C. (D) is the transverse section at the level indicated by the dotted line in C. (E–E3) RT-PCR data showing the mRNA expression of BMP2, Nkx-2.5 and GATA4 after treatment with high glucose. The RT-PCR result was the representative of 4 independent experiments. **p<0.01 and ***p<0.001 indicating statistically significant differences between high-glucose-treated and control embryos (independent samples T test). (F and G) GATA5 in situ hybridization was performed in the embryos with one side exposed to RAPA (F). F1 and F2 are high-magnification images from the sites indicated by dotted squares in F. G is the transverse section at the level indicated by dotted line in F. Abbreviations: Ctr, control and HG, high glucose. Scale bars = 200 μm in A, C, F; 100 μm in A1-A2, C1-C2, F1-F2; 100 μm in B and D, G.
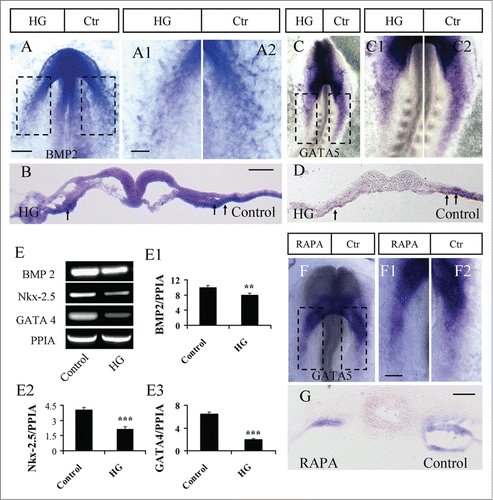
Discussion
There is no doubt that fetal cardiac defects are closely associated with maternal hyperglycemia because they occur up to 5 times more often in pre-gestational diabetic mothers compared to those without diabetes.Citation1 There are many types of rodent animal models that have been employed to date to explore the mechanism of diabetic teratogenesis. Based on the characteristics of type 1 and 2 diabetes, these animal models include genetically and chemically induced models to examine the influence of hyperglycemia on fetal development. Early gastrulating chick embryos were employed as an experimental model in this study. As an avian model, it is a rather simple procedure to treat chick embryos with glucose or other compounds in vivoCitation31 without the influence of maternal factors.
Cardiac defects are among the most common congenital defects induced by pre-gestational diabetes. Here, we initially characterized the phenotypes of heart tube malformation after treatment with high glucose in HH0 chick embryos (). The first type of malformed-heart tube phenotype is an enlarged heart tube compared to a normal heart tube at the same stage (; Fig. S1). The enlarged heart tube typically presented with a thickened ventricular wall and a large heart tube cavity. This is in accordance with the cardiac hypertrophy induced by pre-gestational diabetes at later developmental stages. The first phenotype was present in the highest percent of total heart tube malformations (). The second phenotype, dextrocardia, accounted for approximately 40 percent of total malformations. The third-most common phenotype is the failure of fusion of bilateral primary heart tubes. The latter 2 phenotypes are presumed to be related to the appearance of double-outlets in the right ventricle and cardia bifida in the last stage of heart development.
The aforementioned malformations of heart tubes most likely originate from abnormal primordial bilateral primary heart fields. The bilateral primary heart fields originate symmetrically from lateral-anterior migration of precardiac mesoderm cells, which delaminate from the anterior primitive streak of gastrulating embryos.Citation14,15 Therefore, we observed the migration of those GFP+ precardiac mesoderm cells in the presence of high glucose and discovered that high glucose significantly promoted cell migration toward to the primary heart field (). BrdU incorporation experiments indicated that augmented GFP+ precardiac mesoderm cells were irrelevant to cell proliferation (). To differentiate into cardiomyocytes, embryonic precardiac precursors need to appear at the site of primary heart fields at the right time because the embryonic microenvironment is indispensable for appropriate differentiation. Thus, inappropriate velocity of cell migration certainly would result in dysplasia during development. It was reported that high-glucose-mediated oxidative stress impairs cell migration and activates RhoA in vitro.Citation49 In the present study, we found that RhoA expression was not altered in high-glucose-treated embryos. This suggested that RhoA was not necessary in the process of high-glucose-induced heart tube malformation.
To study the effect of autophagy on the pre-implantation murine embryo, Adastra KL, et al. employed both in vitro and in vivo models to demonstrate that the cells responded to hyperglycemic environment by increasing activation of autophagy in a differential pattern within the embryo.Citation47 We also found that LC3B expression was dramatically enhanced by exposure to high glucose in comparison to the control. This result was similar with the high glucose can increase the autophagy in human retinal pigment epithelium cell line.Citation50 Additionally, we found that Atg7 was distinctly expressed in the cardiac crescent, the site of bilateral primary heart fields fusion (). In our previous study, we demonstrated that Atg7- mediated autophagy is involved in the development of avian gastrula.Citation30 These suggest that autophagy participates in the heart tube formation. Although mTOR might not be the major signaling pathway involved in the regulation of high-glucose-induced autophagy, and it was actually achieved through ROS mediated ER stress signaling.Citation50 Interestingly, the activation of autophagy by RAPA treatment (mTOR signaling inhibitor) in gastrulating chick embryos also led to a heart tube malformation phenotype similar to that induced by high glucose (). Therefore, we suggested that heart tube malformation would occur as long as excess autophagy was generated during early embryogenesis. In another word, it is speculated which pathway leads to excess autophagy generation is not very important.
Then we explored the correlation between autophagy and the primordial formation of primary heart fields. The migration assay of GFP+ precardiac mesoderm cells in the presence of RAPA showed that cell migration was accelerated () as well. Conversely, GFP+ precardiac mesoderm cell migration was slowed by treatment with 3-MA or CQ, inhibitors of autophagy ( and N-P1). At last, there were less migratory DiI+ mesoderm cells in the 3-MA+HG-treated or CQ+HG-treated sides in comparison to the PBS+HG-treated sides of embryos, indicating that the autophagy was involved in high glucose-induced the abnormal migration of cardiac progenitor cells. Our data showed that autophagy is involved in the regulation of precardiac cell migration toward the primary heart field, but we do not yet know how autophagy affects cell migration. The effect could possibly occur via cellular cytoskeleton modulation or relevant cytokine release and remains an open question.
It is reported that cardiomyocyte fate is determined in the gastrulating chick HH3-stage embryo. These cells are located within the anterior one-third of the primitive streak, excluding Hensen's node.Citation19 The cell fate of cardiomyocytes is specialized due to the specific expression of cardiac-related genes such as BMP2, GATA5 and Nkx-2.5 at the gastrulation stage. As zinc-finger transcription factors, the GATA family perform vital functions in heart formation. Bilateral heart tubes (cardia bifida) and fewer cardiomyocytes were present in GATA4 knock-out mice. Similar to GATA4, a lack of GATA5 also leads to cardia bifida, while the over-expression of GATA5 results in ectopic Nkx-2.5 expression with ectopic beating cells in the fish embryo.Citation19 In this study, we also found that GATA4 and GATA5 expression was reduced in the presence of high glucose and RAPA (), as was the expression of BMP2 and Nkx-2.5 (, E-E3). FGF8, another key gene in heart tube formation, was not influenced by high-glucose treatment (Fig. S5). These alterations in heart tube-relevant gene expression could be the molecular biological basis for the observed phenotypes.
Overall, the influence of high-glucose exposure on heart tube formation in early embryos was briefly investigated. The earlier phenotypes of heart tube malformation in the presence of high glucose could last until the dysplasia of mature heart tissue. More interestingly, heart tube malformation occurring in the presence of high glucose was closely associated with autophagy level in gastrulating embryos and the migration of precardiac mesoderm cells toward primary heart fields. In other words, autophagy might be involved in the process of high-glucose-induced heart tube malformation, which might be achieved by affecting cardiac-relevant gene (Nkx-2.5, GATA4, GATA5, and BMP2) expression (). There is no doubt that further experimentation is required to explore the precise molecular biological mechanism.
Figure 6. Model depicting the hypothesis for high-glucose-induced malformations of heart tubes. High glucose might cause the autophagic disturbance, abnormal cell migration, promoted ROS of the cardiac progenitors, heart tube formation-related gene expression, including BMP2, Nkx-2.5, GATA4 and GATA5, was also adversely influenced. It is well known that the excess ROS also can induce the autophagy even we did not prove it in this study. In sum, we suggested that excess cell autophagy induced by high glucose is the key factor for the heart tube malformation development.
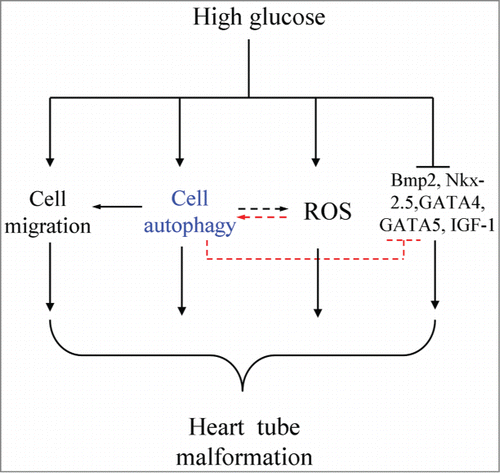
Disclosure of Potential Conflicts of Interest
No potential conflicts of interest were disclosed.
Author Contributions
GW, WH, SC, XW, SL YL and JL performed the experiments and collected the data; GW, MC, LC, DL, and XY designed the study and analyzed the data; XY wrote manuscript.
1000170_Supplementary_Materials.zip
Download Zip (10 MB)Acknowledgments
We would like to thank Prof. Maurice van den Hoff for the BMP2 plasmid and Dr. Thomas M. Schultheiss for the GATA5 plasmid.
Funding
This study was supported by NSFC grant (31401230; 31071054; 30971493); Guangdong Natural Science Foundation (S2013010013392, S2011010001593); the Fundamental Research Funds for the Central Universities (21614319); Guangdong Province Medical Research Foundation (B2014217); China Postdoctoral Science Foundation (2014M560694); Major Program of Guangdong College students technology innovation project; Students Research Training Program Fund (CX14263); Outstanding doctors' training program of Ministry of Education.
References
- Ejdesjo A, Wentzel P, Eriksson UJ. Influence of maternal metabolism and parental genetics on fetal maldevelopment in diabetic rat pregnancy. Am J Physiol Endocrinol Metab 2012; 302:E1198-209; PMID:22374754; http://dx.doi.org/10.1152/ajpendo.00661.2011
- Gheorman L, Iliescu D, Ceausu I, Paulescu D, Plesea IE, Gheorman V. Importance of early complex evaluation in high-risk pregnancy associated to diabetes mellitus. case presentation and review of the literature. Rom J Morphol Embryol 2011; 52:1127-32; PMID:22119836
- Macintosh MC, Fleming KM, Bailey JA, Doyle P, Modder J, Acolet D, Golightly S, Miller A. Perinatal mortality and congenital anomalies in babies of women with type 1 or type 2 diabetes in England, wales, and northern Ireland: population based study. BMJ 2006; 333:177; PMID:16782722; http://dx.doi.org/10.1136/bmj.38856.692986.AE
- Zangen SW, Yaffe P, Shechtman S, Zangen DH, Ornoy A. The role of reactive oxygen species in diabetes-induced anomalies in embryos of cohen diabetic rats. Int J Exp Diabetes Res 2002; 3:247-55; PMID:12546278; http://dx.doi.org/10.1080/15604280214933
- Gillery P, Monboisse JC, Maquart FX, Borel JP. Does oxygen free radical increased formation explain long term complications of diabetes mellitus? Med Hypotheses 1989; 29:47-50; PMID:2664434; http://dx.doi.org/10.1016/0306-9877(89)90167-9
- Hunt JV, Dean RT, Wolff SP. Hydroxyl radical production and autoxidative glycosylation. glucose autoxidation as the cause of protein damage in the experimental glycation model of diabetes mellitus and ageing. Biochem J 1988; 256:205-12; PMID:2851978
- Baynes JW. Role of oxidative stress in development of complications in diabetes. Diabetes 1991; 40:405-12; PMID:2010041; http://dx.doi.org/10.2337/diab.40.4.405
- Yang X, Borg LA, Eriksson UJ. Altered metabolism and superoxide generation in neural tissue of rat embryos exposed to high glucose. Am J Physiol 1997; 272:E173-80; PMID:9038867
- Wolff SP. Diabetes mellitus and free radicals. free radicals, transition metals and oxidative stress in the aetiology of diabetes mellitus and complications. Br Med Bull 1993; 49:642-52; PMID:8221029
- Corrigan N, Brazil DP, McAuliffe F. Fetal cardiac effects of maternal hyperglycemia during pregnancy. Birth Defects Res 2009; 85:523-30; PMID:19180650; http://dx.doi.org/10.1002/bdra.20567
- Rashidi H, Sottile V. The chick embryo: hatching a model for contemporary biomedical research. Bioessays 2009; 31:459-65; PMID:19274658; http://dx.doi.org/10.1002/bies.200800168
- Martinsen BJ. Reference guide to the stages of chick heart embryology. Dev Dyn 2005; 233:1217-37; PMID:15986452; http://dx.doi.org/10.1002/dvdy.20468
- Waldo KL, Kumiski DH, Wallis KT, Stadt HA, Hutson MR, Platt DH, Kirby ML. Conotruncal myocardium arises from a secondary heart field. Development 2001; 128:3179-88; PMID:11688566
- Yang X, Chrisman H, Weijer CJ. PDGF signalling controls the migration of mesoderm cells during chick gastrulation by regulating N-cadherin expression. Development 2008; 135:3521-30; PMID:18832396; http://dx.doi.org/10.1242/dev.023416
- Yang X, Dormann D, Munsterberg AE, Weijer CJ. Cell movement patterns during gastrulation in the chick are controlled by positive and negative chemotaxis mediated by FGF4 and FGF8. Dev Cell 2002; 3:425-37; PMID:12361604; http://dx.doi.org/10.1016/S1534-5807(02)00256-3
- Yue Q, Wagstaff L, Yang X, Weijer C, Munsterberg A. Wnt3a-mediated chemorepulsion controls movement patterns of cardiac progenitors and requires rhoA function. Development 2008; 135:1029-37; PMID:18256196; http://dx.doi.org/10.1242/dev.015321
- Borgave S, Ghodke K, Ghaskadbi S. The heart forming region of early chick embryo is an alternative source of embryonic stem cells. Int J Dev Biol 2009; 53:91-9; PMID:19123130; http://dx.doi.org/10.1387/ijdb.082677sb
- Zaffran S, Kelly RG, Meilhac SM, Buckingham ME, Brown NA. Right ventricular myocardium derives from the anterior heart field. Circ Res 2004; 95:261-8; PMID:15217909; http://dx.doi.org/10.1161/01.RES.0000136815.73623.BE
- Brand T. Heart development: molecular insights into cardiac specification and early morphogenesis. Dev Biol 2003; 258:1-19; PMID:12781678; http://dx.doi.org/10.1016/S0012-1606(03)00112-X
- Beiman M, Shilo BZ, Volk T. Heartless, a drosophila FGF receptor homolog, is essential for cell migration and establishment of several mesodermal lineages. Genes Dev 1996; 10:2993-3002; PMID:8957000; http://dx.doi.org/10.1101/gad.10.23.2993
- Aburto MR, Sanchez-Calderon H, Hurle JM, Varela-Nieto I, Magarinos M. Early otic development depends on autophagy for apoptotic cell clearance and neural differentiation. Cell Death Dis 2012; 3:e394; PMID:23034329; http://dx.doi.org/10.1038/cddis.2012.132
- Chen N, Karantza V. Autophagy as a therapeutic target in cancer. Cancer Biol Ther 2011; 11:157-68; PMID:21228626; http://dx.doi.org/10.4161/cbt.11.2.14622
- Kuma A, Hatano M, Matsui M, Yamamoto A, Nakaya H, Yoshimori T, Ohsumi Y, Tokuhisa T, Mizushima N. The role of autophagy during the early neonatal starvation period. Nature 2004; 432:1032-6; PMID:15525940; http://dx.doi.org/10.1038/nature03029
- Komatsu M, Waguri S, Ueno T, Iwata J, Murata S, Tanida I, Ezaki J, Mizushima N, Ohsumi Y, Uchiyama Y, et al. Impairment of starvation-induced and constitutive autophagy in atg7-deficient mice. J Cell Biol 2005; 169:425-34; PMID:15866887; http://dx.doi.org/10.1083/jcb.200412022
- Hamburger V, Hamilton HL. A series of normal stages in the development of the chick embryo. 1951. Dev Dyn 1992; 195:231-72; PMID:1304821; http://dx.doi.org/10.1002/aja.1001950404
- Chapman SC, Collignon J, Schoenwolf GC, Lumsden A. Improved method for chick whole-embryo culture using a filter paper carrier. Dev Dyn 2001; 220:284-9; PMID:11241836; http://dx.doi.org/10.1002/1097-0177(20010301)220:3%3c284::AID-DVDY1102%3e3.0.CO;2-5
- Klionsky DJ, Abdalla FC, Abeliovich H, Abraham RT, Acevedo-Arozena A, Adeli K, Agholme L, Agnello M, Agostinis P, Aguirre-Ghiso JA, et al. Guidelines for the use and interpretation of assays for monitoring autophagy. Autophagy 2012; 8:445-544; PMID:22966490; http://dx.doi.org/10.4161/auto.19496
- Seglen PO, Gordon PB. Three-Methyladenine: specific inhibitor of autophagic/lysosomal protein degradation in isolated rat hepatocytes. Proc Natl Acad Sci U S A 1982; 79:1889-92; PMID:6952238; http://dx.doi.org/10.1073/pnas.79.6.1889
- Rubinsztein DC, Gestwicki JE, Murphy LO, Klionsky DJ. Potential therapeutic applications of autophagy. Nat Rev 2007; 6:304-12; PMID:17396135
- Lu WH, Wang G, Li Y, Li S, Song XY, Wang XY, Chuai M, Lee KK, Cao L, Yang X. Autophagy functions on EMT in gastrulation of avian embryo. Cell Cycle 2014; 13:2752-64; PMID:25486362; http://dx.doi.org/10.4161/15384101.2015.945850
- Larger E, Marre M, Corvol P, Gasc JM. Hyperglycemia-induced defects in angiogenesis in the chicken chorioallantoic membrane model. Diabetes 2004; 53:752-61; PMID:14988261
- McElhinny AS, Perry CN, Witt CC, Labeit S, Gregorio CC. Muscle-specific RING finger-2 (MURF-2) is important for microtubule, intermediate filament and sarcomeric M-line maintenance in striated muscle development. J Cell Sci 2004; 117:3175-88; PMID:15199100; http://dx.doi.org/10.1242/jcs.01158
- Henrique D, Adam J, Myat A, Chitnis A, Lewis J, Ish-Horowicz D. Expression of a delta homologue in prospective neurons in the chick. Nature 1995; 375:787-90; PMID:7596411; http://dx.doi.org/10.1038/375787a0
- Somi S, Buffing AA, Moorman AF, Van Den Hoff MJ. Dynamic patterns of expression of BMP isoforms 2, 4, 5, 6, and 7 during chicken heart development. Anat Rec 2004; 279:636-51; PMID:15224405; http://dx.doi.org/10.1002/ar.a.20031
- Kamei CN, Kempf H, Yelin R, Daoud G, James RG, Lassar AB, Tabin CJ, Schultheiss TM. Promotion of avian endothelial cell differentiation by GATA transcription factors. Dev Biol 2011; 353:29-37; PMID:21354132; http://dx.doi.org/10.1016/j.ydbio.2011.02.016
- Maroto M, Reshef R, Munsterberg AE, Koester S, Goulding M, Lassar AB. Ectopic pax-3 activates MyoD and Myf-5 expression in embryonic mesoderm and neural tissue. Cell 1997; 89:139-48; PMID:9094722; http://dx.doi.org/10.1016/S0092-8674(00)80190-7
- Dugaiczyk A, Haron JA, Stone EM, Dennison OE, Rothblum KN, Schwartz RJ. Cloning and sequencing of a deoxyribonucleic acid copy of glyceraldehyde-3-phosphate dehydrogenase messenger ribonucleic acid isolated from chicken muscle. Biochemistry 1983; 22:1605-13; PMID:6303388; http://dx.doi.org/10.1021/bi00276a013
- Jin YM, Zhao SZ, Zhang ZL, Chen Y, Cheng X, Chuai M, Liu GS, Lee KK, Yang X. High glucose level induces cardiovascular dysplasia during early embryo development. Exp Clin Endocrinol Diabetes 2013; 121:448-54; PMID:23864493; http://dx.doi.org/10.1055/s-0033-1349080
- Wei L, Roberts W, Wang L, Yamada M, Zhang S, Zhao Z, Rivkees SA, Schwartz RJ, Imanaka-Yoshida K. Rho kinases play an obligatory role in vertebrate embryonic organogenesis. Development 2001; 128:2953-62; PMID:11532918
- Laverriere AC, MacNeill C, Mueller C, Poelmann RE, Burch JB, Evans T. GATA-4/5/6, a subfamily of three transcription factors transcribed in developing heart and gut. J Biol Chem 1994; 269:23177-84; PMID:8083222
- Plageman TF Jr., Yutzey KE. Differential expression and function of Tbx5 and Tbx20 in cardiac development. J Biol Chem 2004; 279:19026-34; PMID:14978031; http://dx.doi.org/10.1074/jbc.M314041200
- Troncoso R, Ibarra C, Vicencio JM, Jaimovich E, Lavandero S. New insights into IGF-1 signaling in the heart. Trends Endocrinol Metab 2014; 25:128-37; PMID:24380833; http://dx.doi.org/10.1016/j.tem.2013.12.002
- Itoh N, Ohta H. Pathophysiological roles of FGF signaling in the heart. Front Physiol 2013; 4:247; PMID:24046748; http://dx.doi.org/10.3389/fphys.2013.00247
- Matsumoto E, Sasaki S, Kinoshita H, Kito T, Ohta H, Konishi M, Kuwahara K, Nakao K, Itoh N. Angiotensin II-induced cardiac hypertrophy and fibrosis are promoted in mice lacking Fgf16. Genes Cells 2013; 18:544-53; PMID:23600527; http://dx.doi.org/10.1111/gtc.12055
- Li Y, Wang XY, Zhang ZL, Cheng X, Li XD, Chuai M, Lee KK, Kurihara H, Yang X. Excess ROS induced by AAPH leads to myocardial hypertrophy during heart development Int J Cardiol 2014; 176:62-73; PMID: 25037699; http://dx.doi.org/10.1159/000356654
- Li Y, Wang XY, Wu T, Chuai M, Lee KK, Wang LJ, Yang X. PTEN is involved in modulation of vasculogenesis in early chick embryos. Biol Open 2013; 2:587-95; PMID:23789109; http://dx.doi.org/10.1242/bio.20133988
- Adastra KL, Chi MM, Riley JK, Moley KH. A differential autophagic response to hyperglycemia in the developing murine embryo. Reproduction 2011; 141:607-15; PMID:21367963; http://dx.doi.org/10.1530/REP-10-0265
- Li Y, Wang XY, Ma ZL, Chuai M, Munsterberg AE, Lee KK, Yang X. Endoderm contributes to endocardial composition during cardiogenesis. Chin Sci Bull 2014; 59:2749-55; http://dx.doi.org/10.1007/s11434-014-0366-7
- Lamers ML, Almeida ME, Vicente-Manzanares M, Horwitz AF, Santos MF. High glucose-mediated oxidative stress impairs cell migration. PloS one 2011; 6:e22865; PMID:21826213; http://dx.doi.org/10.1371/journal.pone.0022865
- Yao J, Tao ZF, Li CP, Li XM, Cao GF, Jiang Q, Yan B. Regulation of autophagy by high glucose in human retinal pigment epithelium. Cell Physiol Biochem 2014; 33:107-16; PMID:24481000; http://dx.doi.org/10.1159/000356654