Abstract
An adequate supply of nucleotides is essential for accurate DNA replication, and inappropriate deoxyribonucleotide triphosphate (dNTP) concentrations can lead to replication stress, a common source of DNA damage, genomic instability and tumourigenesis. Here, we provide evidence that Erk5 is necessary for correct nucleotide supply during erythroid development. Mice with Erk5 knockout in the haematopoietic lineage showed impaired erythroid development in bone marrow, accompanied by altered dNTP levels and increased DNA mutagenesis in erythroid progenitors as detected by exome sequencing. Moreover, Erk5-depleted leukemic Jurkat cells presented a marked sensitivity to thymidine-induced S phase stalling, as evidenced by increased H2AX phosphorylation and apoptosis. The increase in thymidine sensitivity correlated with a higher dTTP/dCTP ratio. These results indicate that Erk5 is necessary to maintain the balance of nucleotide levels, thus preventing dNTP misincorporation and DNA damage in proliferative erythroid progenitors and leukemic Jurkat T cells.
Abbreviations
BrdU | = | bromo-2-deoxyuridine |
DAPI | = | 4′,6-Diamidino-2-phenylindole |
dNTP | = | deoxyribonucleotide triphosphate |
dATP | = | deoxyadenosine triphosphate |
dCTP | = | deoxycytidine triphosphate |
dGTP | = | deoxyguanosine triphosphate |
dTTP | = | thymidine triphosphate |
HU | = | hydroxyurea |
PBS | = | phosphate-buffered saline |
PI | = | propidium iodide |
RNR | = | ribonucleotide reductase |
shRNA | = | small hairpin RNA |
TK1 | = | thymidine kinase |
UTP | = | uridine triphosphate |
Introduction
Erk5 is a member of the mammalian mitogen-activated protein kinase family with a kinase domain that shares 52% of identity with the kinase domain of ERK1/2, but possessing a unique C-terminal domain with transcriptional activity.Citation1 Deletion of Erk5 in mice leads to defective vascular and cardiac development and embryonic lethality.Citation2,Citation3,Citation4 Erk5 controls the expression of genes that regulate angiogenesis and cardiovascular development.Citation5
Erk5 is required for proliferation of epithelial cells in response to epidermal growth factor,Citation6 but cells like T lymphocytes have the capacity to proliferate independently of Erk5.Citation7 During mitosis, Erk5 is phosphorylated in a cdk-dependent manner and translocates to the nuclei.Citation8,9 Mek5/Erk5 pathway activity correlates with metastatic potential of prostateCitation10 and renal tumor cells.Citation11 Erk5 is necessary for proliferation of breast carcinoma cells,Citation12,13 where it contributes to G1/S transition by inhibiting the p21 and p27 cdk inhibitors.Citation14 The tumourigenicity of Erk5 has been related to its capacity to interact with promyelocytic leukemia protein and inhibit its tumor suppressor activity.Citation15 By blocking the interaction of promyelocytic leukemia protein and MDM2 Erk5 allows the inhibition of tumor suppressor p53 by MDM2.Citation16
In the cell, nucleotide metabolism is controlled by 2 pathways: the de novo and salvage pathways. Fuelled by extracellular deoxynucleosides imported into the cell,Citation17 the salvage pathway is necessary for proper haematopoietic development.Citation18,19 Haematopoietic tissues contain high thymidine levels, which increases the cellular dTTP levels produced by the salvage pathway.Citation19 A high dTTP concentration prevents UTP incorporation into DNA and replication stress in erythroid and lymphoid lineages.Citation18,19 Alterations in the activity of enzymes metabolising nucleotides can lead to mutagenesis and tumourigenesis in vitro and in vivoCitation20-22 and produce developmental defects in lymphoid and erythroid lineages,Citation18,23 underscoring the importance of nucleotide metabolism for proper haematopoietic development.
In this study, we uncovered important alterations in the nucleotide metabolism of Erk5-deficient cells, which were associated to impaired erythropoiesis in mice and to hypersensitivity of leukemic Jurkat cells to exogenous thymidine as evidenced by DNA damage and apoptosis.
Results
Thymidine generates lethal DNA damage in Erk5-depleted Jurkat cells
To investigate Erk5 function, we silenced its expression in Jurkat T cells with a shRNA targeting a conserved sequence in Erk5 exon 2 (Jurkat-shErk5 cells), and prepared control shCtrl cell lines with a shRNA containing a scrambled sequence (). As Erk5 activity has been associated with cell cycle progression,Citation14,24 we synchronised the cells in G1/S phase by incubating them with thymidine and then stained them with propidium iodide (PI) for cytofluorometric evaluation of the cell cycle. The PI profiles showed that a fraction of shErk5 cells were polyploid (, cells with 8N DNA and the increase of 4N cell population; see below for further details). Surprisingly, exposure to thymidine was associated with increased cell death in Erk5-depleted cells, as evidenced by the increased fraction of cells with less than 2N DNA content (, subG1 population) and annexin V binding (apoptotic and dead cells) ().
Figure 1. Erk5 depletion sensitizes Jurkat cells to thymidine. (A) Western blot of Erk5 in representative shCtrl and shErk5 cell lines. The blot was reprobed with anti-actin as loading control and the intensity of the protein bands was quantified using quantitative luminescence. (Right) The analysis was repeated with 10 different shErk5 cell lines and 9 different shCtrl cell lines, and the ratio Erk5:actin ± s.d. is shown, *p<0.05. (B) Erk5 mRNA expression as analyzed by reverse transcription and real-time PCR. Erk5:18S values were calculated and normalized to shCtrl, which was given an arbitrary value of 1.0. The analysis was repeated with 10 shErk5 and 9 shCtrl cell lines, and the mean ± s.d. is presented, ***p<0.001. (C) Representative cell cycle profiles of shCtrl and shErk5 cells in asynchronous culture as well as after 18 h, 24 h and 28 h treatment with 2.5 mM thymidine, as analyzed by PI staining and flow cytometry. (Right) Quantification of the subG1 fraction in each condition, n=6, **p<0.01. (D) Annexin V/DAPI analysis of cells after 18h treatment with 1.5 mM thymidine. (Right) Quantification of viability of shCtrl, shERK5 and shErk5+shErk5 cells n=3, *p<0.05 for live cells. (E) Western blot of Erk5 in cell lines shCtrl, shErk5 and shErk5 transfected with Erk5. The blot was reprobed with anti-Gapdh as loading control. (A-B) Unpaired Student's t-test, (C-D) Paired Student's t-test.
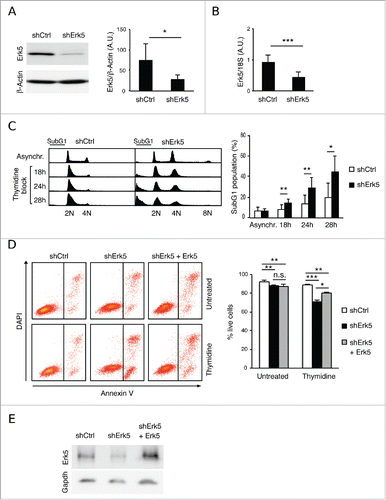
Because this finding was unexpected, we further explored the role of Erk5 knockdown in increasing susceptibility to thymidine. To prove that this was not an off-target effect of the shRNA used, Erk5 levels were reconstituted in shErk5 cells (), and the sensitivity to thymidine was partially reverted (). To ascertain whether hypersensitivity to thymidine was due to replication stalling we incubated the cells with 2 additional transcriptional inhibitors. Aphidicolin did preferentially target shErk5 cells for apoptosis, but the effect was subtle (). In contrast to thymidine, treatment with hydroxyurea (HU), a ribonucleotide reductase (RNR) inhibitor that causes depletion of dNTP pools, produced strong cell death in both cell types (). These results suggest that knockdown of Erk5 make Jurkat cells more sensitive to replication stalling but particularly sensitive to thymidine.
Figure 2. Thymidine increases DNA damage in Erk5-depleted Jurkat cells. (A) Viability of cells after 18 h treatment with 5 μg/mL aphidicolin or 2 mM HU. (Left) Annexin V/DAPI analysis of cells treated with aphidicolin or HU. The numbers inside the plot indicate the percentage of annexin V-positive cells in this particular experiment. (Right) Quantification of apoptosis, n=3, *p<0.05. (B) Representative western blot showing phospho-H2AX (p-H2AX) in shCtrl and shErk5 cell lines at the indicated times after release from an 18 h thymidine block. Histone 3 was used as loading control. (Right) Densitometric analysis of the protein gel blot, the ratio phospho-H2AX:H3 ± s.d. is shown, n=3. (C) Representative cell cycle profiles after 0 h, 8 h, 16 h or 24 h release from 18 h thymidine block, as analyzed by PI staining and flow cytometry. (Right) Quantification of subG1 fraction after release from thymidine block, n=6. A pool of 2 shCtrl or 2 shErk5 cell lines was used in these experiments (A-C). Paired Student's t-test, *p<0.05 **p<0.01.
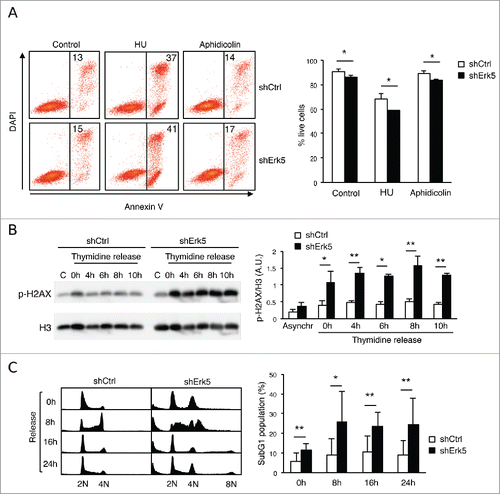
Apoptosis in shErk5 cells treated with thymidine correlated with the presence of DNA damage as determined by phosphorylation of H2AX (, 0 h-time point). After release from thymidine, shErk5 cells were defective in resolving phosphorylated H2AX, indicative of difficulty in repairing the extensive DNA damage (). Moreover, a considerable fraction of shErk5 cells, but not control cells, were committed to death after release from thymidine ().
shErk5 cells also exhibited hypersensitivity to a reduced concentration of thymidine. At 100 µM, a concentration which decreased the proliferation of shCtrl and shErk5 cells (), thymidine did increase phosphorylation of H2AX only in shErk5 cells (). These results indicate that Erk5-depleted Jurkat cells are specifically sensitized to thymidine as evidenced by cumulative DNA damage and apoptosis.
Figure 3. shErk5 cells are more sensitive to a low thymidine concentration. (A) Proliferation of shCtrl, shErk5 and shErk5+Erk5 cells cultured in medium containing 100 μM thymidine ± 1 μM deoxynucleosides (N). Cells were seeded at a concentration of 0.2·106/mL and counted at 24 h, 48 h and 72 h, n=3. (B) Representative western blot showing phospho-H2AX levels in shCtrl and shErk5 lines after 24 h incubation with 100 µM thymidine. (Bottom) Quantification of the phospho-H2AX levels, mean ±s.d., n=3. (C) dNTP levels in normally growing shCtrl and shErk5 cells, mean ±s .d., n=3. (D) Thymidine strongly decreases dCTP in shErk5 cells. dNTP levels in shCtrl and shErk5 cells after 18 h treatment with thymidine, aphidicolin or HU. All values are normalized to the asynchronous control condition which has been given an arbitrary value of 1.0 (dotted gray line), n=5. (E) dTTP:dCTP ratio after 18 h treatment with thymidine, aphidicolin or HU, n=5. (F) Representative protein gel blots showing phospho-Chk1 and phosphor-H2AX levels in shCtrl, shErk5 and shErk5+Erk5 lines after 18 h incubation with thymidine and deoxynucleosides as indicated. The blots were reprobed with Chk1 or GAPDH for loading control. (Bottom) Quantification of the phosphor-H2AX levels, mean ±s.d., n=3, unpaired Student's t-test, * p<0.05 referred to the control sample shCtrl + 100 μM thymidine + deoxynucleosides (basal phosphorylation of H2AX). One each shCtrl, shErk5 and shErk5+Erk5 cell lines were used in experiments (A, F). Pools of 2 shCtrl or 2 shErk5 cell lines were used in experiments (B, C, D, E). (A-E) Paired Student's t-test, *p<0.05 **p<0.01 ***p<0.001.
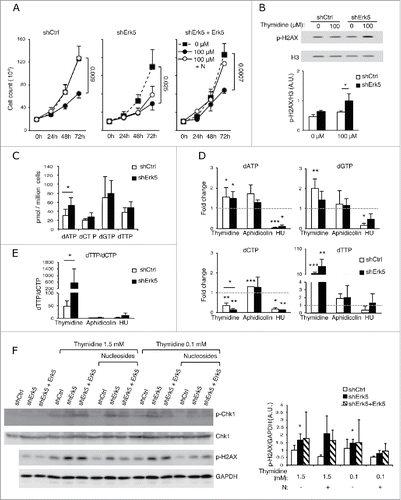
Depletion of Erk5 is synergistic with thymidine in decreasing intracellular levels of dCTP
The strong sensitivity of Erk5-depleted cells to thymidine prompted us to analyze dNTP concentration under the various treatments. Exposure of cells to thymidine leads to increased intracellular dTTP that allosterically inhibits the production of deoxycytidine diphosphate (dCDP) by RNR.Citation17 Since dCDP is the direct precursor of dCTP, the resulting starvation of dCTP slows DNA replication and activates the intra S phase checkpoint.Citation25
dNTP levels in shErk5 and control cells cultured under normal conditions were similar, with the exception of the dATP pool, which showed a higher basal level in shErk5 cells (). Incubation of cells with thymidine increased dTTP levels in both shErk5 and control cells () (the absolute dNTPs values of a similar group of experiments are shown in supplementary material S1A). However, dCTP levels in thymidine-treated shErk5 cells decreased to ~1/7 of untreated cells, whereas dCTP levels in control shCtrl cells were ~1/3 of untreated cells (, supplementary material S1A). The dTTP/dCTP ratio in thymidine-treated shErk5 cells increased more than tenfold above control cell values (, supplementary material S1B). Similarly, when cells were exposed to 0.1 mM thymidine, a subtoxic concentration, decreased dCTP levels and an increased dTTP/dCTP ratio were observed in Erk5-depleted cells but not in control cells ().
Figure 4. Low thymidine concentration decreased dCTP levels in shErk5 cells. (A) dNTP levels in shCtrl and shErk5 cells after 24 h treatment with 100 µM thymidine. All values are normalized to the asynchronous control condition which has been given an arbitrary value of 1.0 (dotted gray line), n=4. (B) dTTP:dCTP ratio after 18 h treatment with thymidine, n=4. (C) The addition of 1 µM deoxynucleosides (N: dA + dC + dG) rescues dCTP levels and decreases subG1 fraction in shERK5 cells. dNTP levels in shCtrl and shERK5 cells after 18 h treatment with 2.5 mM thymidine with or without the addition of deoxynucleosides. All values are normalized to the asynchronous control condition, which has been given an arbitrary value of 1.0 (dotted gray line), n=4. (D) dTTP:dCTP ratio after 18 h treatment with thymidine or thymidine + deoxynucleosides. (E) Cell viability after 18 h treatment with thymidine or thymidine + deoxynucleosides, n=3. Pools of 2 shCtrl or 2 shERK5 cell lines were used in experiments (A-D). One shCtrl and one shERK5 cell lines were used in experiments (E). Student's t-test *p<0.05.
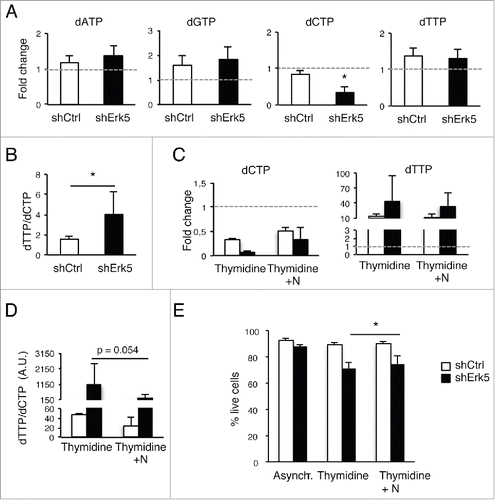
The decrease in proliferation observed when cells were incubated with 0.1 mM thymidine () could be reversed by addition of deoxynucleosides to the cell culture in shCtrl and shErk5+Erk5 cells, but only partially in shErk5 cells (), suggesting that shERK5 cells have a difficulty in processing exogenous deoxynucleosides. Reconstitution of Erk5 in shErk5 cells improved their proliferation in presence of 0.1 mM thymidine (supplementrary Fig. S1C).
The DNA damage produced by inhibition of DNA replication triggers the phosphorylation and activation of Chk1.Citation26 We analyzed the ATR-dependent Chk1 phosphorylation at Ser-317. Although both cell types, shCtrl and shErk5, showed an increase of Chk1 phosphorylation after incubation with thymidine, the increase was stronger in the latter (). These results show the genomic stress produced by the arrest of replication with thymidine.
Given that p21Waf1/Cip1 and Chk1 prevent apoptosis during replication stress by thymidine,Citation27 we next determined whether the increased death response of shErk5 cells to thymidine was due to absence of p21. We measured p21 RNA expression levels by real-time quantitative reverse transcriptase PCR because the regulation of expression of p21 (Cdkn1a) is principally transcriptional.Citation28 The increased death response of shErk5 cells to thymidine was not due to absence of p21Waf1/Cip1 (supplementary material Fig. S2A) or Chk1 (, supplementary material Fig. S2B).
During DNA replication, the presence of nucleotide imbalance leads to misincorporation, DNA damage and chromosomal instability.Citation29 Of note, there was an increase in DNA damage (H2AX phosphorylation) in Erk5-depleted cells incubated with thymidine (). In line with a role for nucleotide imbalance as the origin of DNA damage and cell death in thymidine-treated shErk5 cells, the addition of nucleosides decreased the phosphorylation of H2AX and Chk1 (), partially restored dCTP values () and partially restored cell viability ().
Thus, Erk5 downregulation in Jurkat cells increased thymidine-mediated inhibition of dCTP production, resulting in severe dCTP depletion, DNA damage and apoptosis. Additionally, whereas inhibition of RNR with HU decreased dGTP levels in control cells, it did not do so in Erk5-depleted cells (). The lesser inhibitory effect of HU on the dGTP pool in shErk5 cells as compared to control cells, and the larger decrease in dCTP in thymidine-treated shErk5 cells, indicate that dNTP metabolism is altered in these cells.
Activity of the salvage pathway is not increased in shErk5 cells
The data presented thus far suggest a role for Erk5 in dNTP metabolism. Differences in RNR2 content were not responsible for the effect of thymidine on shErk5 cells (supplementary material Fig. S2C). We thus explored whether Erk5 regulates the activity of the salvage pathway. Metabolic flux through the salvage pathway is regulated by thymidine kinase 1 (TK1), which phosphorylates thymidine, and deoxycytidine kinase, which phosphorylates deoxycytidine, deoxyadenosine and deoxyguanosine.Citation17,30,31 To test whether thymidine salvaging is increased in Erk5-depleted cells, we incubated cells with [3H]-thymidine and analyzed cellular uptake and TK activity in cell extracts. Although [3H]-thymidine uptake by shErk5 cells was slightly higher, TK activity in shErk5 and control cells was similar (supplementary material Fig. S2D,E). Moreover, no changes in deoxycytidine kinase 1 mRNA expression were observed in Erk5-depleted cells (supplementary material Fig. S2F). In addition, catabolism of dNTP by Samhd1 did not seem to be responsible for the observed differences (supplementary material Fig. S2G). These results indicate that the increase in the dTTP/dCTP ratio in shErk5 cells is independent of TK1, suggesting that other metabolic enzymes must be implicated.
Erk5 downregulation increases aneuploidy in Jurkat cells
The increased aneuploidy in Erk5-depleted cells () was investigated further. We observed that 16 out of 20 shErk5 cell lines generated in 2 independent transfections contained a higher percentage of polyploid cells (>4 n) than Jurkat lines expressing a scrambled shRNA (supplementary material Fig. S3A, B), supporting the notion that aneuploidy is promoted in Jurkat cells as a consequence of Erk5 downregulation.
Absence of PTEN or p53 in cells leads to genomic instability and polyploidisation.Citation32-34 Jurkat cells lack p53 and PTEN function,Citation35,36 and hence they are permissive for chromosomal alterations and the generation of aneuploidy. Erk5 was then downregulated in 3 additional p53-negative cell lines: U937, HL-60 and Hut-78.Citation37-38 When the stable tetraploid and PTEN-negative U937 cellsCitation39 were transfected with shErk5, a cell population with lower DNA content was observed (supplementary material Fig. S4). Erk5 downregulation in Hut-78 and HL-60 cells, which express PTEN,Citation39,40 did not substantially modify the DNA content, though tetraploidy (or cells in the G2 phase) increased slightly in the former (supplementary material Fig. S4). These results support a role for Erk5 absence in accelerating the chromosomal instability and aneuploidy brought about by absence of p53 and PTEN.
Mice with Erk5-deficient haematopoietic cells show ineffective erythropoiesis
Cell line experiments showed that downregulation of Erk5 synergised with thymidine in decreasing dCTP and generating replication stress. It is therefore conceivable that the elevated thymidine concentration naturally occurring in haematopoietic tissuesCitation19 could produce conditions for replication stress if Erk5 is absent. To test this notion, we bred loxP-flanked Erk5 mice (Erk5fl/fl mice) with Vav-Cre–transgenic mice to generate an animal model with excision of Erk5 in the haematopoietic lineage (here called Erk5-/-VavCre) (). The Vav-Cre transgene provides efficient excision during an early stage of haematopoietic development when many cell lineage precursors are actively proliferating.Citation7,41
Figure 5. Ineffective erythropoiesis in mice with Erk5-deficiency in haematopoietic cells. (A) Representative western blot showing the absence of Erk5 protein in a total lysate of bone marrow cells (left) and splenocytes (right) from an Erk5fl/fl mouse and an Erk5-/-VavCre mouse. (B-C) Cellularity in bone marrow (n≥6 ) (B) and spleen (n≥9 ) (C) of Erk5fl/fl and Erk5-/-VavCre mice. (D) Splenic weight normalized to body weight (n≥7 ). (E) Representative spleens of Erk5fl/fl and Erk5-/-VavCre mice. (F) Splenic erythroid population. Dot plots representing erythroid precursor distribution (EryA, EryB, EryC) based on forward scatter (FSC) and CD71 expression (top right) in the ter119 positive population (top left). Percentage of ter119+ population in Erk5fl/fl and Erk5-/-VavCre bone marrow (bottom left), and percentage of ter119+ subpopulations EryA, EryB, EryC (bottom right), n≥8 . (G) Bone marrow erythroid population. Dot plots representing erythroid precursor distribution (EryA, EryB, EryC) based on FSC and CD71 expression (top right) in the ter119+ population (top left). Percentage of ter119+ population in Erk5fl/fl and Erk5-/-VavCre bone marrow (bottom left), and percentage of ter119+ subpopulations EryA, EryB, EryC (bottom right), n≥8 . Paired Student's t-test, *p<0.05 **p<0.01 ***p<0.001.
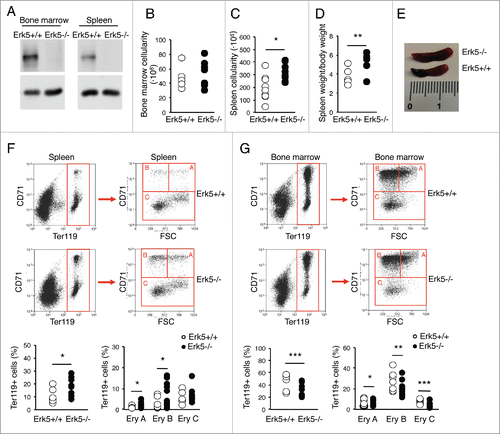
Haematopoietic excision of Erk5 resulted in enlarged spleen and increased spleen cellularity in mice aged 1–2 months old, whereas bone marrow cellularity was unaltered (). The erythroid lineage precursors were enlarged in the spleen of Erk5-/-VavCre mice (), including the highly proliferative nucleated erythroblast cells EryA (ter119+, CD71+, FSC high) and their lineage descendants EryB (ter119+, CD71+, FSC low),Citation42 indicative of increased extramedullary erythropoiesis. In contrast, the erythroid ter119+ population in Erk5-/-VavCre bone marrow was decreased, including EryA, EryB and EryC progenitor cells (). These results indicate that Erk5-depletion impairs erythroid development and causes ineffective erythropoiesis in bone marrow, promoting erythropoiesis in the spleen.
Absence of Erk5 induces a partial block in B cell development
Besides the erythroid lineage, bone marrow distribution of mature B cells (B220high IgM+), but not B cell progenitors (B220low), in Erk5-/-VavCre mice showed evidence of altered development (). The mature B220high B cells were underrepresented in Erk5-/-VavCre bone marrow (). Despite this difference, the spleen of Erk5-/-VavCre and control mice presented the same percentage of mature B cells ().Citation7 In contrast to B cell development, thymic T cell precursors in Erk5-/-VavCre mice showed a distribution similar to that in control miceCitation7,42 (data not shown).Citation43
Figure 6. Distribution of B cell progenitors in bone marrow and spleen of Erk5-/-VavCre and control Erk5fl/fl mice. (A) Bone marrow B220+ population in Erk5fl/fl (n=7) and Erk5-/-VavCre (n=10) mice. (B) Percentage of bone marrow B cell precursors in Erk5fl/fl (n=7) and Erk5-/-VavCre (n=10) mice (top). Dot plots show lymphoid precursor distribution (B220lowIgM-, B220lowIgM+, B220highIgM+) in the bone marrow population (bottom). (C) Percentage of splenic B cells (n≥7 ) (left). Dot plots show lymphoid precursor distribution (B220lowIgM-, B220lowIgM+, B220highIgM+) in the splenic population (right). Paired Student's t-test, **p<0.01.
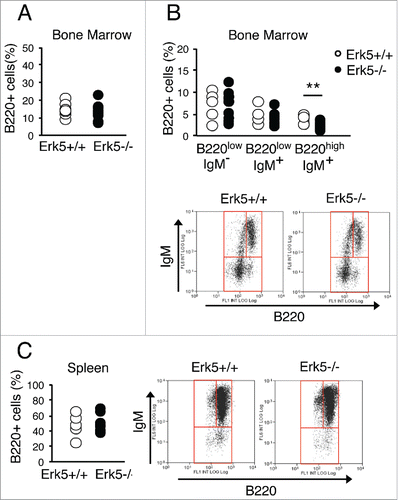
Cell cycle deceleration in bone marrow ter119+ cells of Erk5-/-VavCre mice
We further investigated the mechanism responsible for the alterations in erythroid development. The deficit of bone marrow EryA, EryB and EryC cells could be secondary to proliferation defects in Erk5-deficient cells, or to increased apoptosis. We analyzed the cell cycle profile of Erk5-/-VavCre mice. No differences in cell cycle distribution of total bone marrow cells were observable (). However in ter119+ erythroid progenitors we found that in comparison to control mice, the percentage of Erk5-/-VavCre cells in S phase shifted slightly toward G1 (). In contrast, ter119+ cell cycle distributions in the spleen of Erk5-/-VavCre and control mice were indistinguishable (), indicating that the decreased proliferation of ter119+ cells was a bone marrow-specific condition of Erk5-/-VavCre mice. A small increase in apoptosis levels of bone marrow ter119+ cells was detected in Erk5-/-VavCre mice ().
Figure 7. Absence of Erk5 alters cell cycle progression and increases mutation rate in erythroid precursors in vivo. (A-D) Percentage of bone marrow cells (A, n≥8 ), bone marrow ter119+ cells (B, n≥8 ), spleen cells (C, n≥8 ) and spleen ter119+ cells (D, n≥8 ) in each cell cycle phase of control Erk5fl/fl and Erk5-/-VavCre mice. (E) Percentage of cells in S/G2-phase in the spleen (top) and in the ter119+ fraction of the spleen (bottom). The numbers inside the plot indicate the percentage of cells BrdU+/DAPI+ (cells in S/G2-phase) in this particular experiment. The BrdU-positive cells were detected by incubation of permeabilized cells with mouse anti-BrdU antibody, followed by staining with Alexa 488-conjugated F(ab')2 anti-mouse antibody and analysis by flow cytometry. (Right) Mean of 3 mice +/-SD. (F) Analysis of apoptosis in bone marrow by Annexin V and PI staining (n=2 for each genotype). Paired t-test *p<0.05. (G) Intracellular dNTP levels in bone marrow ter119+ cells, n=3. dATP was undetectable in the samples. (H-I) Exome sequencing in ter119+ cells from the bone marrow. Number and type of mutations (H) and number and percentage (I) of single nucleotide variation (SNV) of a control heterozygous Erk5+/-VavCre and Erk5-/-VavCre mouse. Paired Student's t-test, *p<0.05.
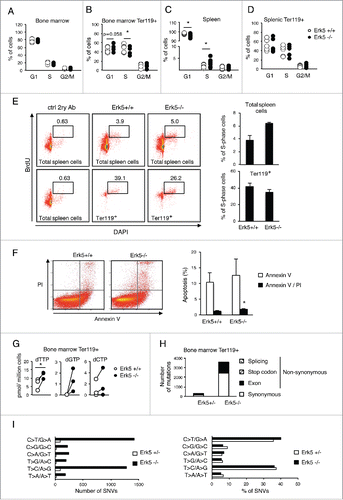
In spleen of Erk5-/-VavCre mice, the amount of cycling cells was significantly higher than in control mice as evidenced by cell cycle profile analysis with PI () and by in vivo BrdU incorporation (). This contrasts with no increase of S-phase cells in the splenic ter119+ population (). This suggests that the expansion of ter119+ erythroid precursors in the spleen of Erk5-/-VavCre mice (), rather than a faster cycling of these cells (), was responsible for the increase in total cycling cells.
These data indicate that the lower amount of erythroid precursors in bone marrow of Erk5-/-VavCre mice is mainly produced by their lesser proliferation and increased apoptosis.
Erk5-/-VavCre mice show altered dNTP levels in ter119+ population
Results from shErk5 cell lines showed that Erk5-depletion rendered Jurkat cells sensitive to thymidine, evidenced by further decreasing intracellular dCTP and leading to DNA damage and apoptosis. We surmised that the reduction in the ter119+ immature erythroid population in bone marrow of Erk5-/-VavCre mice was related to changes in dNTP metabolism. Interestingly, dTTP levels in the ter119+ population in Erk5-/-VavCre marrow increased (), whereas no significative differences in the other dNTP were observed (dATP could not be detected), suggesting that Erk5-depleted cells have increased salvaging of thymidine and/or decreased catabolism of dTTP. The total dNTP content in bone marrow (as well as that in thymus and spleen) revealed no differences between genotypes, suggesting that the dTTP differences found in bone marrow ter119+ population are cell-specific (data not shown).
Erk5-/-VavCre mice show increased mutagenesis in ter119+ population
Changes in cellular dNTP concentration generate proliferative stress and increase mutagenesis by reducing the fidelity of DNA synthesis.Citation17 One of the mechanisms explaining increased mutagenesis induced by imbalanced dNTP is misincorporation of incorrect dNTP by DNA polymerase.Citation17,44 To test whether the higher thymidine concentration present in haematopoietic tissues could cause mutations in Erk5-/-VavCre mice, we sorted bone marrow ter119+ cells from Erk5-/-VavCre and Erk5+/-VavCre mice and performed exome sequencing to compare the single-base mutations in the coding regions of erythroid genomes. The results show that the mutation rate in Erk5-/-VavCre was 14-fold higher than that in the Erk5+/-VavCre mice (, , supplementary material Table S1). Classification of the mutated bases showed an increase for all types of mutations without preference for any single-nucleotide variation in comparison to control (, , supplementary material Table S1). These data indicate that erythroid precursors from Erk5-/-VavCre mice display a higher rate of mutagenesis, and suggest that increased intracellular dTTP in Erk5-/-VavCre marrow could be the causative agent.
Table 1. Summary of the number and type of mutations identified in Erk5-/-VavCre compared to a heterozygote control Erk5+/-VavCre mouse.
Discussion
The preservation of balanced dNTP pools in cells is necessary to avoid replication stress and genomic instability. dNTPs pools are generated by 2 cellular pathways, the de novo and salvage pathways. Deoxynucleoside salvaging during B cell, T cell and erythroid development is necessary to fine tune dNTP levels and avoid replication stress in their highly proliferative progenitors.Citation18,19
The results presented here show that erythroid progenitors without Erk5 have higher dTTP levels, associated with enhanced mutagenesis and inefficient erythropoiesis in bone marrow, but not in the spleen. On average, bone marrow contains twice the amount of thymidine than spleen.Citation19 Thymidine salvaging in bone marrow could generate replication stress in highly proliferative haematopoietic precursors of Erk5−/−VavCre mice if dNTP metabolism is not properly geared, and these are subsequently forced to immigrate to more permissive niches such as the spleen.Citation18 In addition to erythroid progenitors, B cell development also showed signs of stress, as the mature B220high B cell population was underrepresented in Erk5−/−VavCre marrow. Of note, while this manuscript was being submitted the alterations of B-cell development reported here have been confirmed in another paper.Citation45 During B cell development Erk5 is necessary to link BAFF-dependent signals to expression of anti-apoptotic genes and cell survival.Citation45
We also explored the effect of Erk5 depletion in human leukemic cells. Interestingly, Erk5 knockdown in Jurkat cells led to increased basal dATP level and to hypersensitivity to thymidine, increasing the dTTP/dCTP ratio and resulting in DNA damage and apoptosis. The hypersensitivity of human leukemic shErk5 cells to thymidine correlated with the data obtained for Erk5−/−VavCre mice showing higher dTTP levels, and mutagenesis in bone marrow erythroid precursors.
Furthermore, depletion of Erk5 in human leukemic cells led to increased aneuploidy. The leukemic cells assayed here were p53−/− and/or pten−/−, which have intrinsic genomic instabilityCitation32,33,46 and are permissive for polyploidisation.Citation34 The observed increase in aneuploidy induced by Erk5 silencing could result from initial dNTP misincorporation due to nucleotide imbalance, which may produce DNA damage and chromosomal instability,Citation29 presumably through mitotic nondisjunction.Citation47 An initial dNTP imbalance during the generation of shErk5 cells could later be compensated for by additional modifications to allow cell growth.
Curiously, yeast Slt2, the functional homolog of human Erk5, is required for appropriate regulation of RNR genes in response to DNA damage,Citation48 and is necessary for resistance to dNTP deficiency induced by HU.Citation49,50 These data indicate different but related roles of Erk5 in mammals and yeast.
In summary, our study has established a central role of Erk5 in controlling DNA replication during murine haematopoiesis and in thymidine-stressed human T leukemia cells, linking Erk5 to the regulation of erythroid development as well as to the preservation of haematopoietic homeostasis. The Erk5 contribution to proper DNA replication was achieved by control of dNTP balance, although the connection linking Erk5 to the activity of key dNTP metabolic enzymes has not been ascertained in this study. These results identify for the first time a necessary role of Erk5 in control of dNTP metabolism in the haematopoietic system.
Materials and Methods
Mice
Erk5fl/fl mice were kindly provided by Cathy Tournier (University of Manchester, Manchester, UK).Citation7 Erk5fl/fl mice were bred to Vav-Cre mice kindly provided by Thomas Graf (CRG, Universitat Pompeu Fabra, Barcelona, Spain).Citation41 All mice were treated in accordance with institutional guidelines and national laws and policies, and procedures were approved by the Departament d'Agricultura, Ramaderia, Pesca, Alimentació i Medi Natural of Generalitat de Catalunya. Experiments were conducted on 4 and 8 week-old mice. To genotype mice, the following oligonucleotides were used: to detect floxed Erk5, forward 5′- tccatgctgttagtcctttgg -3′, reverse 5′- agcggctgtgaagagtgaat -3′ (floxed amplicon = 260bp, wild-type amplicon = 200bp). Presence of Vav-Cre was analyzed with the oligonucleotides: forward 5′- cgagtgatgaggttcgcaag-3′, reverse 5′- atcttcaggttctgcgggaa -3′.
Cell culture and reagents
The cell lines used in this study were obtained from the European Collection of Cell Cultures. Human cell lines Jurkat E6.1, U937 and Hut 78 were grown in RPMI 1640 medium (ref. BE12–702F, Lonza) supplemented with 9% FBS, penicillin/streptomycin, and 2 mM L-glutamine. HL-60 cells were grown in RPMI 1640 medium supplemented with 20% FBS and 2 mM L-glutamine. For thymidine/aphidicolin/HU block experiments, Jurkat E6.1 transfectant pools were used (2 shCtrl lines + 2 shERK5 lines).
For murine cell preparations, single cell suspensions were isolated from spleen and bone marrow of the mice. The suspension was counted to determine the cellularity of the organ, and then mature red blood cells were discarded from the suspension by overlaying cells on a solution of 16% iodixanol, 0.63% NaCl and 10 mM Hepes and centrifuged at 900x g for 30 minutes. The mononuclear fraction of the density gradient was collected, washed with PBS, counted and stained or fixed.
Thymidine, aphidicolin, hydroxyurea (HU), deoxyadenosine, deoxycytosine, deoxyguanosine and roscovitine were all from (Sigma-Aldrich, Madrid Spain).
Stable transfections
In order to obtain stably transfected cell lines, Jurkat, HL60, U937 and Hut78 cell lines were transfected with a vector expressing either a scrambled shRNA (shCtrl) or a shRNA directed to a conserved sequence of the exon 2 of Erk5 (shErk5) (ref. TRCN0000001354; Sigma-Aldrich). For each transfection, 1·106 cells were electroporated (Multiporator, Eppendorf) and seeded in 10 wells of a 24-well plate. Selection of the lines expressing the shRNA was carried out by adding 0.3 μg/mL puromycin to the medium.
To replenish normal levels of Erk5 in shErk5 cells, one shErk5 cell line was stably transfected with a plasmid coding for Erk5 (a kind gift of Dr. Simon Arthur, MRC Protein Phosphorylation Unit) generating several “shErk5+Erk5 cell” lines that were tested for Erk5 content.
Western blot
Cells were lysed in Laemmli buffer and loaded on acrylamide gels (8 to 12%, depending on the mass of the protein of interest). Transfer to nitrocellulose membranes and immunoblotting was performed as previously described.Citation51 Incubation with primary antibody (goat anti-RNR2 diluted 1:1000, Santa Cruz Biotechnology #sc-10844; mouse anti-H2AX phosphorylated (S139) diluted 1:1500, Biolegend #613401; rabbit anti-Chk1 phosphorylated (S317) diluted 1:1000, Bethyl #A304–673A; goat anti-Chk1 diluted 1:1000, Bethyl #A300–162A; rabbit anti-H3 diluted 1:1500, Biolegend #601901) was performed overnight at 4°C under agitation. Next day, after washing, the membrane was incubated with the secondary antibody (anti-mouse IgG HRP conjugated1:2000 Sigma; anti-rabbit HRP conjugated 1:2500 Dako; anti-Goat HRP conjugated Dako) for 1 hour, under agitation at room temperature. Western blots were quantified on a Luminescent Image Analyzer LAS-3000 (Fujifilm). Densitometry analysis was performed using Fiji software.
Quantitative RT-PCR
Cells were lysed in RNA lysis buffer and RNA was extracted using the PureLink→ RNA Mini Kit (Life Technologies), according to the manufacturer's instructions. Total RNA was reverse-transcribed to cDNA (SuperScriptIII, Invitrogen) and real-time PCR was performed using Sybr-green for detection (Promega). The oligonucleotides used in this study were the following: for quantitative determination of human Erk5 cDNA, forward 5′-gcctatggagtggtgtcctc-3′; reverse 5′-ggtcgctttccatcaggtc-3′; for human p21 cDNA, forward 5′-cagcagaggaagaccatgtg-3′; reverse 5′-ggcgtttggagtggtagaaa-3′; for human ChK1, forward 5′-caggggtggtttatctgcat-3′; reverse 5′-ttcaacaaacgctcacgatt-3′; for human deoxycytidine kinase 1, forward 5′-aagaggttctgcccttctcc-3′; reverse 5′-gaaccacttcccaatcctca-3′; for human Samhd1, forward 5′-aggggtggggtatctagcag-3′; reverse 5′-tgttcatgcgtccatttcac-3′.
Immunostaining
For extracellular labeling, splenocytes and bone marrow cells were stained with saturating amounts of primary antibodies conjugated to fluorochromes (anti-Ter119 FITC diluted 1:600, Biolegend; anti-CD71 PE diluted 1:600, Becton Dickinson; anti-B220 FITC diluted 1:25, Immunotools; anti-IgM diluted 1:500, Life Technologies) in PBS 2% FBS for 30 minutes at 4°C. After 30 minutes, cells were washed with PBS and analyzed using an FC500MPL or a Gallios flow cytometer (Beckman Coulter, Inc., Fullerton, CA). 4′,6-Diamidino-2-phenylindole (DAPI) was added to the samples in order to discard the dead cells. Fluorescence was collected on the logarithmic scale. The cell population was selected by gating in a forward scatter vs. side scatter dot plot, excluding aggregates and cell debris, and by excluding the DAPI positive population.
Cell cycle analysis
In order to determine the cell cycle of Jurkat, Hut 78, U937 and HL-60 cell lines, 1·106 cells were fixed in 70% ethanol and stored at −20°C. Samples were washed once with PBS and incubated in 0.1% triton X100, 0.02% RNAse A, 0.0005% PI in PBS for 30 minutes at room temperature in the dark. For cell cycle analysis of primary murine splenocytes and bone marrow cells, 1·106 cells were fixed in 2% paraformaldehyde in PBS, washed with PBS and stained with PI as above. Samples were analyzed in a Coulter XL cytometer and a subsequent cell cycle profile analysis was performed using cytomation summit or FlowJo software. We used 5-bromo-2-deoxyuridine (BrdU) incorporation to study DNA synthesis. For this purpose, mice were given BrdU (17 mg/kg b.w. ip) 1 h prior to harvest of spleen tissue. Cells were permeabilized with 0.2% saponin and the DNA denatured with HCl according to.Citation52
Apoptosis assay
For apoptosis assessment in Jurkat cells, these were incubated with annexin V FITC (diluted 5:100, Immunotools) for 15 minutes. Then, PI or DAPI was added and cells were analyzed by flow cytometry. In order to determine the presence of apoptosis in the murine Ter119+ bone marrow population, bone marrow cells were stained with an anti-Ter119 FITC as indicated above, followed by annexin V phycoerythrin (or annexin V FITC) / DAPI staining. Samples were analyzed using a Gallios cytometer.
Sorting
Bone marrow cells were stained with anti-Ter119 FITC antibody, positive cells were sorted using a FACSAria (BD Biosciences), and purities of up to 95% were obtained.
Thymidine uptake and TK assays
Uptake assays were performed as described inCitation53 using [3H]-thymidine. A TK assay was performed as indicated in.Citation54
dNTP analysis
Cells were counted, resuspended in 0.5 mL ice-cold 70% methanol and stored at -20°C. Subsequent dNTP extraction and analysis were performed as described in.Citation19
Exon sequencing
Exome sequences were mapped to the UCSC mouse reference genome, mm10, with Burrows-Wheeler Aligner software.Citation55 Data were processed using the Picard program for marking duplicates and indexing the resulting BAM files (http://picard.sourceforge.net), followed by indel realignment and base recalibration using GATK.Citation56 Variants were called by VarScan2Citation57 under the conditions of minimum coverage of 10, minimum variant frequency of 0.2, and minimum base quality of Phred score 30. Variants were also called with GATK HaplotypeCaller using the default parameters, and the genotyped variants were processed with GATK VariantRecalibrator for variant recalibration. The variants called by both programs were used for variant analysis. Annotations were performed using ANNOVAR with the software-provided mm10 databases.Citation58 Variant Effect Predictor was also used for annotating the variants using Ensembl databases.Citation59 Annotations by both methods were combined.
Statistics
Data are represented by the mean of biological replicates and standard deviation (s.d.). Statistical analysis was performed in GraphPad Prism (La Jolla, CA, USA), as detailed in each figure legend. Differences were considered significant when *p<0.05, **p<0.01, ***p<0.001.
Disclosure of Potential Conflicts of Interest
No potential conflicts of interest were disclosed.
Supplemental Material
Supplemental data for this article can be accessed on the publisher's website.
Author Contributions
M.A-I. and E.E. conceived, designed and performed most of the experiments. X.R-C. and A.G. performed experiments. B.D., Y.C.K and S.M.W. performed and analyzed exome sequencing. M.A-I., X.R-C., E.E. analyzed the data. M.A-I., X.R-C., M.R. and E.E. wrote the manuscript.
1120914_Supplemental_Material.zip
Download Zip (470.9 KB)Acknowledgments
We thank Cathy Tournier (University of Manchester, Manchester, UK) for providing Erk5fl/fl mice. We wish to thank the technical staff of the FACS Facility and the staff of the Animal Facility (Facultat de Psicologia) for maintaining the mice.
Funding
This work was supported by grant FBG300412 from the Fundació Bosch i Gimpera, University of Barcelona (to M.R.). X.R-C. and M.A-I. were supported by a predoctoral fellowship from the Fundació Bosch i Gimpera (FBG300412).
References
- Kasler HG, Victoria J, Duramad O, Winoto A. ERK5 is a novel type of mitogen-activated protein kinase containing a transcriptional activation domain. Mol Cell Biol 2000; 20:8382-9; PMID:11046135; http://dx.doi.org/10.1128/MCB.20.22.8382-8389.2000
- Regan CP, Li W, Boucher DM, Spatz S, Su MS, Kuida K. Erk5 null mice display multiple extraembryonic vascular and embryonic cardiovascular defects. Proc Natl Acad Sci USA 2002; 99:9248-53; PMID:12093914; http://dx.doi.org/10.1073/pnas.142293999
- Sohn SJ, Sarvis BK, Cado D, Winoto A. ERK5 MAPK regulates embryonic angiogenesis and acts as a hypoxia-sensitive repressor of vascular endothelial growth factor expression. J Biol Chem 2002; 277:43344-51; PMID:12221099; http://dx.doi.org/10.1074/jbc.M207573200
- Yan L, Carr J, Ashby PR, Murry-Tait V, Thompson C, Arthur JSC. Knockout of ERK5 causes multiple defects in placental and embryonic development. BMC Dev Biol 2003; 3:11; PMID:14675480; http://dx.doi.org/10.1186/1471-213X-3-11
- Sohn SJ, Li D, Lee LK, Winoto A. Transcriptional regulation of tissue-specific genes by the ERK5 mitogen-activated protein kinase. Mol Cell Biol 2005; 25:8553-66; PMID:16166637; http://dx.doi.org/10.1128/MCB.25.19.8553-8566.2005
- Kato Y, Tapping RI, Huang S, Watson MH, Ulevitch RJ, Lee JD. Bmk1/Erk5 is required for cell proliferation induced by epidermal growth factor. Nature 1998; 395:713-6; PMID:9790194; http://dx.doi.org/10.1038/27234
- Ananieva O, Macdonald A, Wang X, Mccoy CE, Mcilrath J, Tournier C, Arthur JSC. ERK5 regulation in naïve T-cell activation and survival. Eur J Immunol 2008; 38:2534-47; PMID:18792406; http://dx.doi.org/10.1002/eji.200737867
- Díaz-Rodríguez E, Pandiella A. Multisite phosphorylation of Erk5 in mitosis. J Cell Sci 2010; 123:3146-56; http://dx.doi.org/10.1242/jcs.070516
- Iñesta-Vaquera FA, Campbell DG, Tournier C, Gómez N, Lizcano JM, Cuenda A. Alternative ERK5 regulation by phosphorylation during the cell cycle. Cell Signal 2010; 22:1829-37; http://dx.doi.org/10.1016/j.cellsig.2010.07.010
- Mehta PB, Jenkins BL, McCarthy L, Thilak L, Robson CN, Neal DE, Leung HY. MEK5 overexpression is associated with metastatic prostate cancer, and stimulates proliferation, MMP-9 expression and invasion. Oncogene 2003; 22:1381-9; PMID:12618764; http://dx.doi.org/10.1038/sj.onc.1206154
- Arias-González L, Moreno-Gimeno I, Del Campo AR, Serrano-Oviedo L, Valero ML, Esparís-Ogando A, la Cruz-Morcillo de MÁ, Melgar-Rojas P, García-Cano J, Cimas FJ, et al. ERK5/BMK1 is a novel target of the tumor suppressor VHL: implication in clear cell renal carcinoma. Neoplasia 2013; 15:649-59; http://dx.doi.org/10.1593/neo.121896
- Esparís-Ogando A, Díaz-Rodríguez E, Montero JC, Yuste L, Crespo P, Pandiella A. Erk5 participates in neuregulin signal transduction and is constitutively active in breast cancer cells overexpressing ErbB2. Mol Cell Biol 2002; 22:270-85; http://dx.doi.org/10.1128/MCB.22.1.270-285.2002
- Madak-Erdogan Z, Ventrella R, Petry L, Katzenellenbogen BS. Novel Roles for ERK5 and Cofilin as Critical Mediators Linking ERalpha-Driven Transcription, Actin Reorganization and Invasiveness in Breast Cancer. Mol Cancer Res 2014; 12:714-27; PMID:24505128; http://dx.doi.org/10.1158/1541-7786.MCR-13-0588
- Perez-Madrigal D, Finegan KG, Paramo B, Tournier C. The extracellular-regulated protein kinase 5 (ERK5) promotes cell proliferation through the down-regulation of inhibitors of cyclin dependent protein kinases (CDKs). Cell Signal 2012; 24:2360-8; PMID:22917534; http://dx.doi.org/10.1016/j.cellsig.2012.08.001
- Yang Q, Deng X, Lu B, Cameron M, Fearns C, Patricelli MP, Yates JR, Gray NS, Lee J-D. Pharmacological inhibition of BMK1 suppresses tumor growth through promyelocytic leukemia protein. Cancer Cell 2010; 18:258-67; PMID:20832753; http://dx.doi.org/10.1016/j.ccr.2010.08.008
- Yang Q, Liao L, Deng X, Chen R, Gray NS, Yates JR, Lee JD. BMK1 is involved in the regulation of p53 through disrupting the PML-MDM2 interaction. Oncogene 2013; 32:3156-64; PMID:22869143; http://dx.doi.org/10.1038/onc.2012.332
- Reichard P. Interactions between deoxyribonucleotide and DNA synthesis. Annu Rev Biochem 1988; 57:349-74; PMID:3052277; http://dx.doi.org/10.1146/annurev.bi.57.070188.002025
- Toy G, Austin WR, Liao H-I, Cheng D, Singh A, Campbell DO, Ishikawa T-O, Lehmann LW, Satyamurthy N, Phelps ME, et al. Requirement for deoxycytidine kinase in T and B lymphocyte development. Proc Natl Acad Sci USA 2010; 107:5551-6; PMID:20080663; http://dx.doi.org/10.1073/pnas.0913900107
- Austin WR, Armijo AL, Campbell DO, Singh AS, Hsieh T, Nathanson D, Herschman HR, Phelps ME, Witte ON, Czernin J, et al. Nucleoside salvage pathway kinases regulate hematopoiesis by linking nucleotide metabolism with replication stress. J Exp Med 2012; 209:2215-28; PMID:23148236; http://dx.doi.org/10.1084/jem.20121061
- Voeller D, Rahman L, Zajac-Kaye M. Elevated levels of thymidylate synthase linked to neoplastic transformation of mammalian cells. Cell Cycle 2004; 3:1005-7; PMID:15280655; http://dx.doi.org/10.4161/cc.3.8.1064
- Rahman L, Voeller D, Rahman M, Lipkowitz S, Allegra C, Barrett JC, Kaye FJ, Zajac-Kaye M. Thymidylate synthase as an oncogene: a novel role for an essential DNA synthesis enzyme. Cancer Cell 2004; 5:341-51; PMID:15093541; http://dx.doi.org/10.1016/S1535-6108(04)00080-7
- Xu X, Page JL, Surtees JA, Liu H, Lagedrost S, Lu Y, Bronson R, Alani E, Nikitin AY, Weiss RS. Broad overexpression of ribonucleotide reductase genes in mice specifically induces lung neoplasms. Cancer Res 2008; 68:2652-60; PMID:18413732; http://dx.doi.org/10.1158/0008-5472.CAN-07-5873
- Dobrovolsky VN, McGarrity LJ, VonTungeln LS, Mittelstaedt RA, Morris SM, Beland FA, Heflich RH. Micronucleated erythrocyte frequency in control and azidothymidine-treated Tk+/+, Tk+/− and Tk−/− mice. Mutat Res 2005; 570:227-35; http://dx.doi.org/10.1016/j.mrfmmm.2004.11.006
- Mizunuma M, Hirata D, Miyahara K, Tsuchiya E, Miyakawa T. Role of calcineurin and Mpk1 in regulating the onset of mitosis in budding yeast. Nature 1998; 392:303-6; PMID:9521328; http://dx.doi.org/10.1038/32695
- Sørensen CS, Syljuåsen RG. Safeguarding genome integrity: the checkpoint kinases ATR, CHK1 and WEE1 restrain CDK activity during normal DNA replication. Nucleic Acids Res 2012; 40:477-86; PMID:21937510; http://dx.doi.org/10.1093/nar/gkr697
- Zhang Y, Hunter T. Roles of Chk1 in cell biology and cancer therapy. Int J Cancer 2014; 134:1013-23; PMID:23613359; http://dx.doi.org/10.1002/ijc.28226
- Rodriguez R, Meuth M. Chk1 and p21 cooperate to prevent apoptosis during DNA replication fork stress. Mol Biol Cell 2006; 17:402-12; PMID:16280359; http://dx.doi.org/10.1091/mbc.E05-07-0594
- Gartel AL, Tyner AL. Transcriptional regulation of the p21((WAF1/CIP1)) gene. Exp Cell Res 1999; 246:280-9; PMID:9925742; http://dx.doi.org/10.1006/excr.1998.4319
- Mathews CK. DNA precursor metabolism and genomic stability. FASEB J 2006; 20:1300-14; PMID:16816105; http://dx.doi.org/10.1096/fj.06-5730rev
- Bohman C, Eriksson S. Deoxycytidine kinase from human leukemic spleen: preparation and characteristics of homogeneous enzyme. Biochemistry 1988; 27:4258-65; PMID:2844225; http://dx.doi.org/10.1021/bi00412a009
- Sarup JC, Fridland A. Identification of purine deoxyribonucleoside kinases from human leukemia cells: substrate activation by purine and pyrimidine deoxyribonucleosides. Biochemistry 1987; 26:590-7; PMID:3030413; http://dx.doi.org/10.1021/bi00376a034
- Fujiwara T, Bandi M, Nitta M, Ivanova EV, Bronson RT, Pellman D. Cytokinesis failure generating tetraploids promotes tumorigenesis in p53-null cells. Nature 2005; 437:1043-7; PMID:16222300; http://dx.doi.org/10.1038/nature04217
- Shen WH, Balajee AS, Wang J, Wu H, Eng C, Pandolfi PP, Yin Y. Essential Role for Nuclear PTEN in Maintaining Chromosomal Integrity. Cell 2007; 128:157-70; PMID:17218262; http://dx.doi.org/10.1016/j.cell.2006.11.042
- Senovilla L, Vitale I, Galluzzi L, Vivet S, Joza N, Younes AB, Rello-Varona S, Castedo M, Kroemer G. p53 represses the polyploidization of primary mammary epithelial cells by activating apoptosis. Cell Cycle 2009; 8:1380-5; PMID:19342895; http://dx.doi.org/10.4161/cc.8.9.8305
- Shan X, Czar MJ, Bunnell SC, Liu P, Liu Y, Schwartzberg PL, Wange RL. Deficiency of PTEN in Jurkat T cells causes constitutive localization of Itk to the plasma membrane and hyperresponsiveness to CD3 stimulation. Mol Cell Biol 2000; 20:6945-57; PMID:10958690; http://dx.doi.org/10.1128/MCB.20.18.6945-6957.2000
- Kamihira S, Terada C, Sasaki D, Yanagihara K, Tsukasaki K, Hasegawa H, Yamada Y. Aberrant p53 protein expression and function in a panel of hematopoietic cell lines with different p53 mutations. Eur J Haematol 2009; 82:301-7; PMID:19220422; http://dx.doi.org/10.1111/j.1600-0609.2009.01211.x
- Wolf D, Rotter V. Major deletions in the gene encoding the p53 tumor antigen cause lack of p53 expression in HL-60 cells. Proc Natl Acad Sci USA 1985; 82:790-4; PMID:2858093; http://dx.doi.org/10.1073/pnas.82.3.790
- Cheng J, Haas M. Frequent mutations in the p53 tumor suppressor gene in human leukemia T-cell lines. Mol Cell Biol 1990; 10:5502-9; PMID:2144611; http://dx.doi.org/10.1128/MCB.10.10.5502
- Aggerholm A, Grønbaek K, Guldberg P, Hokland P. Mutational analysis of the tumour suppressor gene MMAC1/PTEN in malignant myeloid disorders. Eur J Haematol 2000; 65:109-13; PMID:10966170; http://dx.doi.org/10.1034/j.1600-0609.2000.90181.x
- Xu Z, Stokoe D, Kane LP, Weiss A. The inducible expression of the tumor suppressor gene PTEN promotes apoptosis and decreases cell size by inhibiting the PI3K/Akt pathway in Jurkat T cells. Cell Growth Differ 2002; 13:285-96; PMID:12133897
- Stadtfeld M, Graf T. Assessing the role of hematopoietic plasticity for endothelial and hepatocyte development by non-invasive lineage tracing. Development 2005; 132:203-13; PMID:15576407; http://dx.doi.org/10.1242/dev.01558
- Liu Y. Suppression of Fas-FasL coexpression by erythropoietin mediates erythroblast expansion during the erythropoietic stress response in vivo. Blood 2006; 108:123-33; PMID:16527892; http://dx.doi.org/10.1182/blood-2005-11-4458
- Rovira-Clavé X, Angulo-Ibáñez M, Tournier C, Reina M, Espel E. Dual role of ERK5 in the regulation of T cell receptor expression at the T cell surface. J Leukoc Biol 2015; (in press) :jlb.2A0115–034R.
- Kumar D, Abdulovic AL, Viberg J, Nilsson AK, Kunkel TA, Chabes A. Mechanisms of mutagenesis in vivo due to imbalanced dNTP pools. Nucleic Acids Res 2011; 39:1360-71; PMID:20961955; http://dx.doi.org/10.1093/nar/gkq829
- Jacque E, Schweighoffer E, Tybulewicz VLJ, Ley SC. BAFF activation of the ERK5 MAP kinase pathway regulates B cell survival. J Exp Med 2015; 212:883-92; PMID:25987726; http://dx.doi.org/10.1084/jem.20142127
- Puc J, Keniry M, Li HS, Pandita TK, Choudhury AD, Memeo L, Mansukhani M, Murty VVVS, Gaciong Z, Meek SEM, et al. Lack of PTEN sequesters CHK1 and initiates genetic instability. Cancer Cell 2005; 7:193-204; PMID:15710331; http://dx.doi.org/10.1016/j.ccr.2005.01.009
- Shi Q, King RW. Chromosome nondisjunction yields tetraploid rather than aneuploid cells in human cell lines. Nature 2005; 437:1038-42; PMID:16222248; http://dx.doi.org/10.1038/nature03958
- Bandyopadhyay S, Mehta M, Kuo D, Sung M-K, Chuang R, Jaehnig EJ, Bodenmiller B, Licon K, Copeland W, Shales M, et al. Rewiring of genetic networks in response to DNA damage. Science 2010; 330:1385-9; PMID:21127252; http://dx.doi.org/10.1126/science.1195618
- Queralt E, Igual JC. Functional connection between the Clb5 cyclin, the protein kinase C pathway and the Swi4 transcription factor in Saccharomyces cerevisiae. Genetics 2005; 171:1485-98; PMID:16118191; http://dx.doi.org/10.1534/genetics.105.045005
- Soriano-Carot M, Bañó MC, Igual JC. The yeast mitogen-activated protein kinase Slt2 is involved in the cellular response to genotoxic stress. Cell Div 2012; 7:1; PMID:22296825; http://dx.doi.org/10.1186/1747-1028-7-1
- Rovira-Clavé X, Angulo-Ibáñez M, Reina M, Espel E. The PDZ-binding domain of syndecan-2 inhibits LFA-1 high-affinity conformation. Cell Signal 2014; 26:1489-99; http://dx.doi.org/10.1016/j.cellsig.2014.03.012
- Hammers HJ, Kirchner H, Schlenke P. Ultraviolet-induced detection of halogenated pyrimidines: simultaneous analysis of DNA replication and cellular markers. Cytometry 2000; 40:327-35; PMID:10918283; http://dx.doi.org/10.1002/1097-0320(20000801)40:4%3c327::AID-CYTO8%3e3.0.CO;2-5
- Shu CJ, Campbell DO, Lee JT, Tran AQ, Wengrod JC, Witte ON, Phelps ME, Satyamurthy N, Czernin J, Radu CG. Novel PET probes specific for deoxycytidine kinase. J Nucl Med 2010; 51:1092-8; PMID:20554721; http://dx.doi.org/10.2967/jnumed.109.073361
- Taylor AT, Stafford MA, Jones OW. Properties of thymidine kinase partially purified from human fetal and adult tissue. J Biol Chem 1972; 247:1930-5; PMID:5012768
- Li H, Durbin R. Fast and accurate long-read alignment with Burrows-Wheeler transform. Bioinformatics 2010; 26:589-95; PMID:20080505; http://dx.doi.org/10.1093/bioinformatics/btp698
- DePristo MA, Banks E, Poplin R, Garimella KV, Maguire JR, Hartl C, Philippakis AA, del Angel G, Rivas MA, Hanna M, et al. A framework for variation discovery and genotyping using next-generation DNA sequencing data. Nat Genet 2011; 43:491-8; PMID:21478889; http://dx.doi.org/10.1038/ng.806
- Koboldt DC, Zhang Q, Larson DE, Shen D, McLellan MD, Lin L, Miller CA, Mardis ER, Ding L, Wilson RK. VarScan 2: somatic mutation and copy number alteration discovery in cancer by exome sequencing. Genome Res 2012; 22:568-76; PMID:22300766; http://dx.doi.org/10.1101/gr.129684.111
- Wang K, Li M, Hakonarson H. ANNOVAR: functional annotation of genetic variants from high-throughput sequencing data. Nucleic Acids Res 2010; 38:e164-4; PMID:20601685; http://dx.doi.org/10.1093/nar/gkq603
- McLaren W, Pritchard B, Rios D, Chen Y, Flicek P, Cunningham F. Deriving the consequences of genomic variants with the Ensembl API and SNP Effect Predictor. Bioinformatics 2010; 26:2069-70; PMID:20562413; http://dx.doi.org/10.1093/bioinformatics/btq330