ABSTRACT
Bursicon is the main regulator of post molting and post eclosion processes during arthropod development. The active Bursicon hormone is a heterodimer of Burs-α and Burs-β. However, adult midguts express Burs-α to regulate the intestinal stem cell niche. Here, we examined the potential expression and function of its heterodimeric partner, Burs-β in the adult midgut. Unexpectedly, our evidence suggests that Burs-β is not significantly expressed in the adult midgut. burs-β mutants displayed the characteristic developmental defects but showed wild type-like adult midguts, thus uncoupling the developmental and adult phenotypes seen in burs-α mutants. Gain of function data and ex vivo experiments using a cAMP biosensor, demonstrated that Burs-α is sufficient to drive stem cell quiescence and to activate dLGR2 in the adult midgut.
Our evidence suggests that the post developmental transactivation of dLGR2 in the adult midgut is mediated by Burs-α and that the β subunit of Bursicon is dispensable for these activities.
KEYWORDS:
Introduction
Adult insect ecdysis is a hormonal-mediated process that coordinates somatic changes culminating with the hardening and sclerotization of the cuticle and expansion of the wings.Citation18 The neurohormone Bursicon is the crucial hormone responsible for the coordination of those final steps of adult ecdysis.
The molecular identity of Bursicon remained unknown for decades until the CG13419 locus (called bursicon or burs, hereafter called burs-α) was identified to be responsible for wing inflation.Citation6
Bursicon is a cysteine knot protein, with close homology to the vertebrate Bone Morphogenetic Protein antagonist family members. This class of proteins share the ability to form dimers of an α-subunit and a β-subunit or in some cases they can also form homodimers.Citation8,13
Despite the initial hypothesis that the Bursicon hormone could consist of homodimers, the bioactive Bursicon hormone is a heterodimer of Burs-α and pBurs (Partner of Bursicon, encoded by the CG15284 locus, also known as and hereafter called burs-β).Citation13,14
The Bursicon heterodimer ligates and activates the dLGR2 receptor, encoded by the rickets (rk) locus (CG8930),Citation13 leading to cAMP production. rk mutants phenocopy burs mutants showing similar cuticle tanning and hardening and wing inflation defects. Moreover, the wing inflation defects of rk are refractory to be rescued via Bursicon injection.Citation13
Notably, the binding of Bursicon to dLGR2 was not competed by Burs-α or Burs-β homodimers in cell culture assays, supporting the current notion that only the α/β heterodimer is bioactive in tanning assays and it binds to dLGR2 with higher affinity.Citation13
Paradoxically, CNS immunolabelling studies in Manduca sextaCitation13 indicate that while most Bursicon expressing neuroendocrine cells co-express both subunits, few others unambiguously express only one of the 2 subunits. Therefore, these cells accumulate and likely secrete Burs-α or Burs-β homodimers.Citation15 Nevertheless, the biological role of these Bursicon homodimers, if any, is not well understood. Recently An et al. provided evidence suggesting that homodimers participate in the insect immune response.Citation1
We have recently characterized a previously unknown post-developmental role of Bursicon/dLGR2 signaling. Burs-α is expressed in a subset of enteroendocrine (ee) cells in the mature adult midgut to control the activity of its receptor dLGR2 in the visceral muscle (VM). The VM is part of the intestinal stem cell (ISC) niche, and Bursicon/dLGR2/cAMP signaling suppresses ISC proliferation by modulating the production of the niche derived EGF-like growth factor Vein.Citation17
In this study, we examined the potential expression and function of the heterodimerisation partner of Burs-α, Burs-β, in the adult Drosophila midgut. Our results show that Burs-α is expressed in the adult gut in the absence of its dimerisation partner Burs-β. Indeed, our loss and gain of function data indicate that burs-β mutations, contrary to burs-α, do not affect adult midgut homeostasis, supporting the notion of a Burs-β independent biological activity of Burs-α. We also show that recombinant Burs-α alone is a biologically active peptide, sufficient to activate dLGR2 in the VM leading to increased production of cAMP. Therefore, our evidence suggests that the post developmental Bursicon/dLGR2/cAMP axis in the adult midgut is mediated by the production of the Burs-α subunit alone.
Results
Bursicon-β (Burs-β) is not expressed in the Drosophila adult midgut
Our previous study expanded the biological activity of Bursicon beyond development, showing a role for it in the regulation of adult intestinal stem cells quiescence.Citation17
We demonstrated that the mature (spliced) burs-α transcript is detected in the midgut via quantitative RT-PCR (qPCR) and that Burs-α immunolabelling is restricted to a subset of posterior enteroendocrine cells.Citation17
Consistently, both whole midgut expression databasesCitation3,4 and a cell-specific transcriptomic databaseCitation7 confirmed burs-α expression in the posterior adult midgut and posterior enteroendocrine cells, respectively.
Unlike burs-α, however, these transcriptomic databases indicate that burs-β is not significantly expressed in the adult midgut.
Considering that the ecdysal “tanning hormone” is an obligate heterodimer of the α and the β subunits, we sought to examine the expression of the binding partner of Burs-α -Burs-β- in the adult midgut.
Consistent with Burs-β's known role as a subunit of the heterodimeric tanning hormone during metamorphosis, burs-β transcripts were readily detected in extracts from heads of late/dark pupae, while very low to undetectable expression was found in adult heads extracts (). This result is in agreement with the reported apoptosis of the Burs-β+ve neurons soon after eclosion.Citation9,15 Previously, we reported an age dependent burs-α expression in adult midguts,Citation17 however burs-β transcripts were low to undetectable in adult guts at all ages tested ().
Figure 1. burs-β is not expressed in the adult gut and is not involved in gut homeostasis. (A) RT-qPCR for burs-β mRNA levels relative to rp49 from dark pupae (DP) heads as control, and from heads and midguts at 3 and 14 d of adult life from w1118 flies. While burs-β is highly expressed in head extracts during metamorphosis, levels are low to undetectable in adult tissues. (B) Confocal transversal sections from posterior midguts from flies of the indicated genotypes. Note that flies mutant for the burs-α subunit show epithelial gut hyperplasia (bursz1091), while loss of the burs-β subunit (Dfexc110/pupal6) shows no detectable effects on gut homeostasis. DAPI (blue) and Phalloidin (Green) label nuclei and actin filaments, respectively. (C) Quantification of ISC proliferation, assessed by pH3 counting in posterior midguts from animals of the indicated genotypes. Data are presented as average (n > 10) values ± SEM p values to the control are indicated above each bar. While adult control flies (w1118) have a modest mitotic index, mutant guts for the dLGR2/rk receptor (rk1) or the burs-α subunit (bursz5569 and bursz1091) are hyperproliferative. No difference in the proliferative status is observed in guts from burs-β subunit (pburspupal6/Df(2)110 and Df(2)110/Df(2)Exel6035) mutants. (D) Representative confocal projections of adult posterior midguts quantified in (C), stained for pH3 (Red), and DAPI (blue).
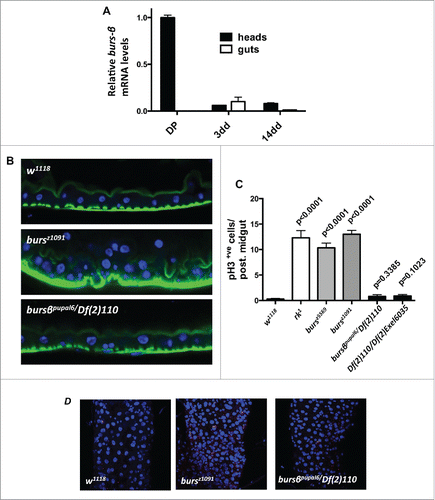
Together with the published transcriptomic databases from the adult Drosophila midgut, our results indicate that burs-β is not significantly expressed in this tissue, supporting the notion of a Burs-β independent activity of Burs-α in adult midgut.
Burs-β is not required for adult midgut homeostasis
In addition to the well-studied developmental phenotypes, our recent workCitation17 indicates that burs-α loss of function (using mutant alleles and adult ee cell-specific knockdown) results in an adult midgut phenotype, characterized by excessive intestinal stem cell proliferation and epithelial multilayering ().
To rigorously test the effects of burs-β loss of function in the adult midgut, we used transheterozygous allelic combinations of 2 different excision lines for the burs-β locus and a heterozygous loss of function mutant of burs-β over a deficiency covering the entire burs-β locus (See Materials and Methods). Adult midguts from these flies were compared to those from mutant animals for either burs-α or its receptor rk, which displayed the characteristic developmental defects, including wing inflation, adult midgut ISC hyperproliferation and epithelial multilayering ().Citation17
Remarkably, while burs-β mutants showed the characteristic developmental defects observed also in burs-α or rk mutant animals (not shown), they displayed quiescence of the intestinal stem cells () resembling wild type tissue, as assessed by phosphorylated Histone3 (pH3) counts. Consistently, burs-β mutants showed a normal monolayered intestinal epithelium ( and Fig. S1A, B), which was morphologically indistinguishable from wild type midguts (Fig. S1A, B). This wild type-like gut phenotype is in sharp contrast with the levels of ISC proliferation and epithelial multilayering observed in adult midguts from burs-α and rk mutants ( and Fig. S1A, B).
Altogether, these results reveal a different requirement of Burs-α and Burs-β production in the adult midgut to sustain its homeostasis. This further supports our previous conclusions that the adult midgut phenotypes from burs-α and rickets mutants are uncoupled from the developmental phenotypes.Citation17
Ectopic overexpression of Burs-α in the midgut suppresses ISC proliferation
Burs-α overexpression in enteroendocrine cells induces suppression of intestinal stem cell proliferation in regenerating or old midguts.Citation17 However, these gain of function experiments on enteroendocrine cells, which express the endogenous Burs-α protein, do not entirely rule out the possibility that minimal levels of Burs-β in these cells could mediate the gain of function Burs-β phenotype in the midgut. To address such possibilities we misexpressed Burs-α in 2 additional gut cytotypes, where we don't normally detect Burs-α immunoreactivity:Citation17 the enterocyte (using the MyoIA-gal4ts driver) and the visceral muscle (using the how-gal4ts driver). The rates of proliferation were quantified in DSS-damaged regenerating midguts, which present relatively high levels of ISC proliferation compared to sucrose only controls. Upon DSS treatment, the mis-expression of Burs-α in the visceral muscle or in enterocytes resulted in a significant suppression of ISC proliferation (). In another paradigm of high ISC proliferation, the aging midgut, we observed a strong suppression of ISC proliferation via misexpression of Burs-α in the VM (). Together with our loss of function analysis these results further confirm that Burs-α does not require Burs-β to direct ISC quiescence.
Figure 2. Burs-α miss-expression drives ISC quiescence. (A and B) Quantification of ISC proliferation, assessed by pH3 counting, in posterior midguts from animals of the indicated genotypes, either treated with sucrose only (control) or damaged by DSS treatment. Data is presented as average values (n > 10) ± SEM p values are indicated in each figure panel. (C) Quantifications of ISC proliferation in aging posterior midguts (30 d at 29°C) from the indicated genotypes. Data are presented as average values (n > 10) ± SEM p values are indicated in figure panel.
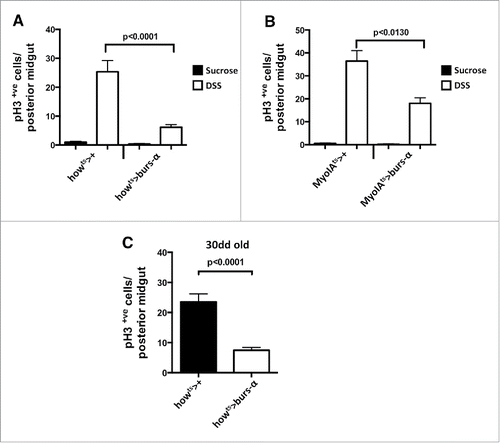
Burs-α is sufficient to activate cAMP production in the adult midgut through dLGR2
We have previously shown that hemolymph extracted from just eclosed animals—known to have high titres of circulating Burs-α/Burs-β heterodimer—resulted in sustained cAMP production in the adult midgut visceral muscle in a dLGR2-dependent manner.Citation17
However, our burs-β transcript expression assays, as well as the mutant phenotype analyses shown above, suggest that Burs-α is sufficient to regulate adult midgut homeostasis in absence of Burs-β. Therefore, we challenged the paradigm that the obligate functional activator of dLGR2 is exclusively the heterodimeric form of Bursicon by testing the ability of the single Burs-α subunit to induce cAMP production in the context of the midgut visceral muscle. To achieve this, we utilized recombinant His-tag Burs-α, which was produced in Yeast and purified using a poly-histidine tag. Its identity was confirmed by western-blotting assays using anti-Burs-α antibodies (). Under reducing conditions, the recombinant protein was detected exclusively with a gel mobility expected for the monomer (approximately 25 kDa). In contrast, when the recombinant protein was allowed to sustain cysteine bridges in non-reducing conditions, a fraction of the recombinant protein was detected as homodimer (approximately 50 kDa, ) as expected for a cysteine knot protein and in agreement with previous reports.Citation8
Figure 3. Recombinant Burs-α can activate dLGR2 in the adult midgut. (A) Time lapse FLIM-FRET of Epac1-camps biosensor activation in the VM upon recombinant Burs-α administration. An increase in cAMP concentration is reported as a shift from blue to red. The frames 0 and 30 min are shown. (B) Quantification of the FLIM/FRET assays as in A. Each time point represents the mean from 3 biological repeats. Note that the effect of Burs-α administration is attenuated by dlgr2/rk knockdown in the VM. p values from 2-way ANOVA with Bonferroni correction are shown. (C) Western blot analysis of recombinant His-Burs-α under reducing (left lane) and non-reducing (right lane) conditions, identified with an anti-Burs-α antibody. Under non-reducing conditions, the formation of a dimeric form is detected.
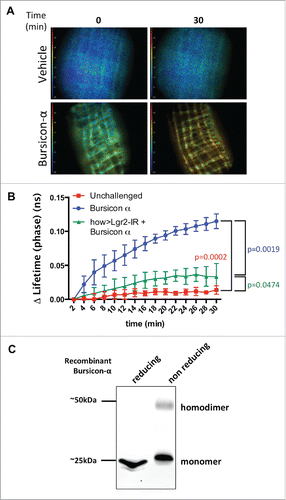
This recombinant Burs-α homodimer-containing solution was then employed in an ex-vivo time course FLIM-FRET assay in midguts expressing the YFP/CFP FRET biosensor UAS-Epac1-camps.Citation16 The reporter was driven specifically within the VM, where the rk/dLGR2 receptor is expressed, to monitor changes in cAMP concentration in the VM.
Contrary to current knowledge in heterologous systems, treatment with the recombinant Burs-α resulted in significant cAMP production, while the buffer-only treatment did not alter cAMP levels (). The cAMP production triggered by Burs-α homodimers was significantly suppressed after VM knockdown of the dLGR2 receptor (). Together, these results suggest that Burs-α, possibly in its homodimeric conformation, can ligate to and activate dLGR2 in the adult midgut visceral muscle leading to increased cAMP levels.
Discussion
The neurohormone Bursicon is released at the end of the molting cycle to mediate the post eclosion behavior, including sclerotization of the cuticle and wing expansion, through the activation of its receptor dLGR2.Citation18
We recently extended the understanding of Bursicon signaling outside the developmental processes, describing a local signaling circuit in the adult Drosophila posterior midgut, which restrains ISC proliferation.Citation17
The current understanding of Bursicon/dLGR2/cAMP signaling predicts that only the heterodimeric complex, made of the 2 cysteine-knot subunits Burs-α and Burs-β, is the functional agonist of the G-protein-coupled receptor dLGR2.
However, some lines of evidence suggest that alternative homodimeric conformations of Burs-α and Burs-β exist both in vivo and in vitro,Citation10 but so far their biological functions remain largely elusive.
In this study, we provide evidence that in the adult Drosophila midgut, Bursicon signaling is mediated by the Burs-α subunit in absence of the heterodimeric partner Burs-β. We have also shown that Burs-β is dispensable for the maintenance of the gut integrity and while mutations in burs-α strongly affect gut morphology and function, the phenotype of mutant burs-β midguts is indistinguishable from wild type ones.
Our ex-vivo live cAMP assay indicates that, at least in the midgut context, Burs-α can activate cAMP production in the VM through its natural receptor dLGR2, making the involvement of an alternative receptor unlikely.
Ectopic overexpression of a UAS-burs-α construct in different midgut cell populations leads to a similar enhanced anti–proliferative effect to the one observed when the construct was expressed in ee cells, making it unlikely that Burs-α can form heterodimeric complexes with other cysteine-knot proteins, and suggesting that a homodimeric complex is responsible for the observed gain of function phenotype.
Elegant studies have previously excluded any biological activity of Burs-α or Burs-β homodimers using in vitro competitive binding experiments.Citation13 However, several lines of evidence support a role for the homodimers. During molting, neurons positive for either one of the 2 Bursicon subunits are detected in Manduca sextaCitation5 and Teleogryllus commodus,Citation5 suggesting that the homodimers could have a role during ecdysis. In Tribolium, injection of double stranded RNA for burs-α, but not burs-β, affected anterior–posterior contractions during ecdysis.Citation2
A report also connects Burs-β homodimers to a relish-dependent activation of immune response genes,Citation1 although through a dLGR2 independent mechanism.
Our study describes the first in vivo evidence for Burs-α activity independent of Burs-β.
While the developmentally active hormone is undoubtedly a heterodimer, our ex vivo live cAMP recording and in vivo functional data argues that the post developmental role of Bursicon/dLGR2 signaling in midgut homeostasis is solely mediated by Burs-α. Although this is in apparent discrepancy with the current knowledge, it is possible that dLGR2 activation by Burs-α requires gut-specific cofactors necessary for homodimer binding. The absence of those putative co-receptor proteins could account for the lack of homodimer activity observed in the heterologous expression system used for competitive binding experiments.Citation13
Our evidence strengthened the previous findings suggesting that Burs-α, probably in its homodimeric form, is the functional peptide responsible for dLGR2 receptor activation in the adult Drosophila midgut visceral muscle acting to restrict intestinal stem cell proliferation and to control midgut homeostasis.
Overall, this study provides key insights to the growing body of evidence supporting a physiological role for Burs-α homodimers and to the general understanding of Bursicon signaling.
Materials and methods
Fly husbandry
Flies were kept on molasses medium in a constant 12 h light-dark cycle. Unless otherwise indicated, adults of the desired experimental genotypes were aged for 10–14 d at 25°C. Food was changed every 2 d.
Fly stocks
The stock w1118 (Bloomington #6326) was used as control strain.
w; cn, bw, rk1 (Bl #3589)
UAS-Epac1-camps (Bl#25407)
UAS-dlgr2-IR (VDRC #105360/kk)
Df(2) Exel6035 (Bl #7518)
Pupal6 (DGRC #101309) is a burs-β loss of function allele
Df(2) 110Citation12
bursz5569 (Citation6)
bursz1091 (Citation6)
how-gal4Citation11 for visceral muscle expression
MyoIA-gal4Citation11 for enterocyte expression
tub-gal80ts (Bl #7108)
UAS-bursCitation17
Further information on Drosophila genes and stocks are available from FlyBase (http://flybase.org/).
RNA extraction and RT-qPCR
Total RNA was extracted as in,Citation17 using RNAeasy mini kit (Qiagen) followed by DNase-treatment (Qiagen). cDNA synthesis was performed using the High-Capacity cDNA reverse transcription kit (Applied Biosystems) and PerfeCTa SYBR Green FastMix (Quanta Biosciences) was used for qPCR following manufacturers instructions. Data were extracted and analyzed using Applied Biosystems 7500 software version 2.0 and GraphPad Prism 5 software. Expression of the target genes was measured relative to that of RpL32 (rp49). Results represent the average from 3 technical replicates for each of 3 independent biological replicates ± SEM
The following primers were used:
Rpl32 f AGGCCCAAGATCGTGAAGAA
Rpl32 r TGTTGCACCAGGAACTTCTTGAA
burs-β f AGGATTGTGCAACAGTCAGG
burs-β r AGCAATGGGTTAGAGTGATGAC
Regeneration assays
Experimental and control flies were collected within 48 hr of eclosion at 18°C and moved to 29°C for 10 d on standard diet. Flies were then transferred to 5% sucrose (vehicle) or 5% sucrose−3% DSS (Fisher Scientific) on paper discs (Whatman) for 4 d. Media were changed daily. Guts were then dissected and analyzed using immunofluorescence and confocal imaging.
Histology and tissue analysis
Immunofluorescence: Tissues were dissected in PBS and fixed for 30–40 min in 4% para-formaldehyde (Polysciences, Inc.). After fixation samples were washed 3 times in PBS + 0.1% Triton X-100 (PBST) and incubated in primary antibodies overnight at 4°C. Samples were then washed as described and subjected to secondary antibody staining for 2 hr at room temperature followed by washing and mounting on Vectashield containing DAPI (Vector Laboratories, Inc.). Primary and secondary antibodies were incubated in PBST+ 0.5% BSA. Rabbit anti-pH3 S10 and S28 1:100 (Cell Signaling); rabbit anti-Bursicon (α-subunit) 1:250 (Luan H, Lemon WC, Peabody NC, 2006). Anti-mouse or anti-rabbit secondary antibodies Alexa 488 or Alexa 594 (Invitrogen) were used at 1:200 or 1:100, respectively. F-Actin was visualized with Alexa 488-Phallodin (Invitrogen) 1:500. Nuclei were counterstained with DAPI. Confocal images were collected using a Zeiss 710 Confocal microscope and processed with Zeiss ZEN 2010, ImageJ or Adobe Photoshop CS.
Quantifications and statistics of histological and tissue analyses
ISC proliferation, scored by the number of pH3+ve cells, was restricted to the posterior midgut from female flies. pH3+ve nuclei in at least 10 midguts where counted manually under a confocal microscope.
The data were plotted using Graphpad Prism 5 software. Results represent average values ± SEM. We used Mann-Whitney test to calculate statistical significance.
Acquisition of fluorescent lifetimes of Epac1-camps FRET reporter
The method was used as previously described.Citation17 Briefly, midguts were exposed on a glass-bottom 35 mm tissue culture dish (MatTek Corporation MA, USA) and imaged on a Nikon Eclipse TE 2000-U microscope to measure CFP lifetime changes based on CFP/YFP FRET (436/20X, T455LP dichroic mirror, 480/40M) and a 445 nm intensity modulated LED for illumination. FLIM-FRET was measured by the frequency domain method using the Lambert Instruments fluorescence attachment (LIFA). 10 µM Fluorescein solution in 0.1 M Tris-Cl (pH > 10) was used as a reference standard with a known lifetime of 4.0 ns. Data were acquired every minute for 30 minutes following the administration of 5 μl of 900nM yeast-produced recombinant Bursicon-α (Cusabio) or S2 medium as control. Each treatment was performed on 3 biological replicates. Five regions of interest (ROI) consistent over the acquisition time for each gut were selected. Data from each ROI were expressed as difference to the basal lifetime and averaged over 2 minutes. A two-way ANOVA test with Bonferroni correction was applied to assess statistically significant differences. p values < 0.05 were considered statistically significant.
Western blotting
50 ng recombinant His-tagged Bursicon-α (Cusabio) was dissolved in loading-buffer containing DTT, β-mercaptoethanol and SDS, for reducing and denaturing conditions, or SDS only for non-reducing, denaturing conditions. Samples were heated for 5 min at 70° C and separated in a 4–12% Tris-HCl PA gel (Bio-Rad), then blotted onto nitrocellulose membranes and immunoblotted with anti-Bursicon antibody, followed by anti-mouse IRDye 800CW secondary antibody (1:1.000) (Licor). Detection was performed with Odyssey CLx imager (Licor).
Disclosure of Potential Conflicts of Interest
No potential conflicts of interest were disclosed.
1121334_Supplemental_Material.zip
Download Zip (145.9 KB)Acknowledgments
We thank B. White, E. M. Dewey, H. W. Honegger, A. J. Hsueh, J. Ewer, the Bloomington stock center and the Developmental Studies Hybridoma Bank for providing advice, fly lines, and reagents.
Funding
The NC3Rs and Cancer Research UK (Grant number A11594) supported this work. Dr. Cordero is supported by a Sir Henry Dale Fellowship jointly funded by the Wellcome Trust and the Royal Society (Grant Number 104103/Z/14/Z).
References
- An S, Dong S, Wang Q, Li S, Gilbert LI, Stanley D, Song Q. Insect neuropeptide bursicon homodimers induce innate immune and stress genes during molting by activating the NF-kappaB transcription factor Relish. PLoS One 2012; 7(3):e34510; PMID:22470576; http://dx.doi.org/10.1371/journal.pone.0034510
- Arakane Y, Li B, Muthukrishnan S, Beeman RW, Kramer KJ, Park Y. Functional analysis of four neuropeptides, EH, ETH, CCAP and bursicon, and their receptors in adult ecdysis behavior of the red flour beetle, Tribolium castaneum. Mech Dev 2008; 125(11–12): 984-95; PMID:18835439; http://dx.doi.org/10.1016/j.mod.2008.09.002
- Buchon N, Osman D, David FP, Fang HY, Boquete JP, Deplancke B, Lemaitre B. Morphological and molecular characterization of adult midgut compartmentalization in Drosophila. Cell Rep 2013; 3(5):1725-38; PMID:23643535; http://dx.doi.org/10.1016/j.celrep.2013.04.001
- Chintapalli VR, Wang J, Dow JA. Using FlyAtlas to identify better Drosophila melanogaster models of human disease. Nat Genet 2007; 39(6):715-20; PMID:17534367; http://dx.doi.org/10.1038/ng2049
- Dai L, Dewey EM, Zitnan D, Luo CW, Honegger HW, Adams ME. Identification, developmental expression, and functions of bursicon in the tobacco hawkmoth, Manduca sexta. J Comp Neurol 2008; 506(5):759-74;PMID:18076057; http://dx.doi.org/10.1002/cne.21575
- Dewey EM, McNabb SL, Ewer J, Kuo GR, Takanishi CL, Truman JW, Honegger HW. Identification of the gene encoding bursicon, an insect neuropeptide responsible for cuticle sclerotization and wing spreading. Curr Biol 2004; 14(13):1208-13; PMID:15242619; http://dx.doi.org/10.1016/j.cub.2004.06.051
- Dutta D, Dobson AJ, Houtz PL, Glasser C, Revah J, Korzelius J, Patel PH, Edgar BA, Buchon N. Regional Cell-Specific Transcriptome Mapping Reveals Regulatory Complexity in the Adult Drosophila Midgut. Cell Rep 2015; 12(2):346-58; PMID:26146076; http://dx.doi.org/10.1016/j.celrep.2015.06.009
- Honegger HW, Dewey EM, Ewer J. Bursicon, the tanning hormone of insects: recent advances following the discovery of its molecular identity. J Comp Physiol A Neuroethol Sens Neural Behav Physiol 2008; 194(12): 989-1005; PMID:19005656; http://dx.doi.org/10.1007/s00359-008-0386-3
- Honegger HW, Estevez-Lao TY, Hillyer JF. Bursicon-expressing neurons undergo apoptosis after adult ecdysis in the mosquito Anopheles gambiae. J Insect Physiol 2011; 57(7):1017-22; PMID:21554887; http://dx.doi.org/10.1016/j.jinsphys.2011.04.019
- Honegger HW, Market D, Pierce LA, Dewey EM, Kostron B, Wilson M, Choi D, Klukas KA, Mesce KA. Cellular localization of bursicon using antisera against partial peptide sequences of this insect cuticle-sclerotizing neurohormone. J Comp Neurol 2002; 452(2):163-77; PMID:12271490; http://dx.doi.org/10.1002/cne.10357
- Jiang H, Patel PH, Kohlmaier A, Grenley MO, McEwen DG, Edgar, BA. Cytokine/Jak/Stat signaling mediates regeneration and homeostasis in the Drosophila midgut. Cell 2009; 137(7):1343-55; PMID:19563763; http://dx.doi.org/10.1016/j.cell.2009.05.014
- Lahr EC, Dean D, Ewer J. Genetic analysis of ecdysis behavior in Drosophila reveals partially overlapping functions of two unrelated neuropeptides. J Neurosci 2012; 32(20):6819-29; PMID:22593051; http://dx.doi.org/10.1523/JNEUROSCI.5301-11.2012
- Luo CW, Dewey EM, Sudo S, Ewer J, Hsu SY, Honegger HW, Hsueh AJ. Bursicon, the insect cuticle-hardening hormone, is a heterodimeric cystine knot protein that activates G protein-coupled receptor LGR2. Proc Natl Acad Sci USA 2005; 102(8):2820-5; PMID:15703293; http://dx.doi.org/10.1073/pnas.0409916102
- Mendive FM, Van Loy T, Claeysen S, Poels J, Williamson M, Hauser F, Grimmelikhuijzen CJ, Vassart G, Vanden Broeck J. Drosophila molting neurohormone bursicon is a heterodimer and the natural agonist of the orphan receptor DLGR2. FEBS Lett 2005; 579(10):2171-6; PMID:15811337; http://dx.doi.org/10.1016/j.febslet.2005.03.006
- Peabody NC, Diao F, Luan H, Wang H, Dewey EM, Honegger HW, White BH. Bursicon functions within the Drosophila CNS to modulate wing expansion behavior, hormone secretion, and cell death. J Neurosci 2008; 28(53):14379-91; PMID:19118171; http://dx.doi.org/10.1523/JNEUROSCI.2842-08.2008
- Ponsioen B, Zhao J, Riedl J, Zwartkruis F, van der Krogt G, Zaccolo M, Moolenaar WH, Bos JL, Jalink K. Detecting cAMP-induced Epac activation by fluorescence resonance energy transfer: Epac as a novel cAMP indicator. EMBO Rep 2004; 5(12):1176-80; PMID:15550931.
- Scopelliti A, Cordero JB, Diao F, Strathdee K, White BH, Sansom OJ, Vidal M. Local control of intestinal stem cell homeostasis by enteroendocrine cells in the adult Drosophila midgut. Curr Biol 2014; 24(11):1199-211; PMID:24814146; http://dx.doi.org/10.1016/j.cub.2014.04.007
- Truman JW. Hormonal control of insect ecdysis: endocrine cascades for coordinating behavior with physiology. Vitam Horm 2005; 73:1-30; PMID:16399406; http://dx.doi.org/10.1016/S0083-6729(05)73001-6