ABSTRACT
Integuments are the first line to protect insects from physical damage and pathogenic infection. In lepidopteran insects, they undergo distinct morphology changes such as scale formation during metamorphosis. However, we know little about integument development and scale formation during this stage. Here, we use the silkworm, Bombyx mori, as a model and show that stem cells in the integument of each segment, but not intersegmental membrane, divide into two scale precursor cells during the spinning stage. In young pupae, the scale precursor cell divides again. One of the daughter cells becomes a mature scale-secreting cell that undergoes several rounds of DNA duplication and the other daughter cell undergoes apoptosis later on. This scale precursor cell division is crucial to the development and differentiation of scale-secreting cells because scale production can be blocked after treatment with the cell division inhibitor paclitaxel. Subsequently, the growth of scale-secreting cells is under the control of 20-hydroxyecdysone but not juvenile hormone since injection of 20-hydroxyecdysone inhibited scale formation. Further work demonstrated that 20-hydroxyecdysone injection inhibits DNA duplication in scale-secreting cells while the expression of scale-forming gene ASH1 was down-regulated by BR-C Z2. Therefore, this research demonstrates that the scale cells of the silkworm develops through stem cell division prior to pupation and then another wave of cell division differentiates these cells into scale secreting cells soon after entrance into the pupal stage. Additionally, DNA duplication and scale production in the scale-secreting cells were found to be under the regulation of 20-hydroxyecdysone.
Introduction
In the life cycle of insects undergoing complete metamorphosis, they experience several rounds of ecdysis.Citation1,Citation2 During each ecdysis, there are many changes in morphology, along with the remodeling of some internal organs.Citation3-5 The insect integument, including the outside solid cuticle, is very crucial to control water evaporation, to protect insects from physical abrasion and pathogen invasion, and to sense the external environment.Citation1,Citation2,Citation6,Citation7 After eclosion, lepidopteran adults are covered by scales, and butterflies, one of the most conspicuous insects, have diverse colored scales on their integument. However, we know very little about the development of the insect integument, particularly regarding scale production at the time of metamorphosis in lepidoptera insects.
Insect ecdysis and metamorphosis are under the coordinated control of two important hormones, which are 20-hydroxyecdysone (20E) and juvenile hormone (JH).Citation8,Citation9 Responding to the changing titers of these two hormones, many development related genes are expressed or inhibited in sequence at different times to regulate insect growth, development and metamorphosis.Citation3,Citation8,Citation10,Citation11 In insects, 20E can bind to the receptor complex, EcR/USP, to regulate the expression of primary response genes like BR-C, E74, E75 and E93.Citation12-15 Subsequently, these primary response genes continue to induce the expression of downstream genes for different physiological functions.Citation3,Citation16,Citation17 Broad-Complex (BR-C), one of the primary genes responding to the increase of 20E, is a family of transcription factors and contains a zinc finger domain that can bind to DNA to regulate metamorphosis.Citation18 During the silkworm metamorphosis, BR-C expression synchronizes with pupal commitmentCitation19,Citation20 and in Manduca sexta and Drosophila melanogaster, the synchronization of BR-C expression and pupal commitment is also observed.Citation21,Citation22 Contrarily, the JH-inducible gene Krüppel homolog 1 (Kr-h1) repress the precocious larval–adult metamorphosis by inhibiting the transcription of BR-C and Ecdysone induced protein 93F (E93).Citation23
During metamorphosis, the changes in insect integument are especially significant.Citation1,Citation24 In lepidopteran insects, the adults are covered by scales that are produced during the pupal stage. Cuticles are also changed during metamorphosisCitation12 and cuticle proteins, like BmorCPG11 and BMWCP5 in the silkworm, are regulated by BR-C via 20E.Citation25-28 In Drosophila, the achaete–scute complex (AS-C) regulates bristle formation.Citation29 In studies where there was loss of complex function, Drosophila adults lost most of their bristles. On the contrary, studies involving over-expression of the complex resulted in adults with many more bristles produced.Citation30-32 In another lepidopteran insect Pseudodeltote coenia, ASH1, the homolog of the Drosophila AS-C gene, was found to be expressed in scale precursors of pupal wing discs.Citation33 Bm-ASH1 also plays a key role during the development of wing scales in B. mori.Citation34,Citation35 Insect hormones can regulate the expression of different genes in the integument. However, very limited information on the cell apoptosis, cell division and DNA duplication in the integuments. An epidermal cell line from the 5th instar larval integument of Helicoverpa armigera (named HaEpi) was established, and the HaEpi cell line responded to 20E application to induce the expression of different genes.Citation36 Another cell line established from imaginal discs of the Indian meal moth (IAL-PID2) caused an accumulation in the G2 phase of cycle and induced the formation of epithelial-like aggregates upon 20E addition.Citation37 When the larval integument of Schistocerca was cultured with alpha-ecdysone, cellular divisions happened, which is a preliminary and indispensable condition for ulterior differentiation. And the application of beta-ecdysone, new ducts were formed in the theorical imaginal exuvia.Citation38 Therefore, we know very little on the morphological changes of integuments upon different hormone releasing in insects.
In this work, we studied the insect integument development from the last feeding stage to the adult stage using the silkworm B. mori as a model. We demonstrate that the integument stem cell in each segment divides into scale precursor cells during the wandering stage, which is crucial for integument development. We also found that cell division and DNA duplication of scale precursor cells soon after pupation are essential for scale development. During this process, a sudden increase in 20E titer after its injection inhibited scale formation by preventing DNA duplication. At the same time, the scale-formation related gene, AS-C1, was down-regulated upon the increase of 20E, and the production of BR-C Z2 was found to indirectly inhibit AS-C1 expression. This work, therefore, describes a picture of lepidopteran insect integument and scale cell development during metamorphosis.
Results
Morphological changes of lepidopteran insect integument
In the silkworm, during the body size changes, the morphology of the integument also transforms from larvae to adults (). The Nistari silkworm larval surface is very smooth with some patterns on the integument (). After the feeding stage, the silkworm larvae begin to wander and spin, during which period the body length shortens ( and ). However, the pupal integument is very different from the larval one (). Pupae are covered by a layer of yellow tanned cuticle, and the larval patterns are lost. After eclosion, the adults are covered by scales on their bodies (). Clearly, beside the body size changes, there are significant changes in the integument morphology during each life cycle in the silkworm.
Figure 1. Morphological changes in the silkworm during metamorphosis. (A) Morphologies of the silkworm at different developmental stages. (a) Silkworm larvae on day 5 of the 5th larval stage (V-5). After that, the larvae enter the wandering (W) stage (b) and begin to spin. At the end of spinning (W-33 h), the larvae become short and enter the pre-pupa stage (c). Morphologies of pupae (day 2 of pupae; P-2) and adults (day 1 of adults; A-1) are also shown. (B) Apoptosis detection from the beginning of wandering (W-0 h) to the P-4 stages. The 7th and 8th segments were sampled for the assay. (a, b) W-33 h; (c-f) P-2; (g-h) P-4. In (a, c, e, g), pictures were merged from those using the blue filter (DAPI) and the red filter (TUNEL). In (b, d, f, h), pictures were merged from those using the DIC filter and the filters described previously. In (a-d, g, h), the 7th segments (SE) and in (e, f) the intersegmental membrane (IM) were assayed. (C) A Summary of apoptosis in the integument from larvae to adults. Apoptosis signals were detected from P-1 to P-4 and the bar thickness indicates the relative amount of apoptotic cells detected. No apoptotic cells were detected in other stages. Bar: (A) 5 mm; (B) 35 μm.
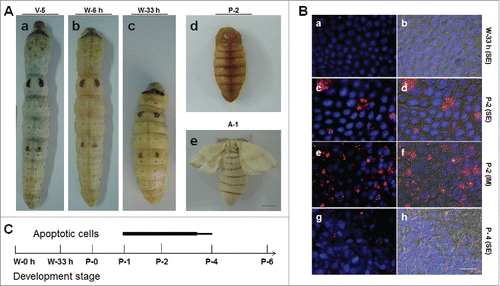
By performing a detailed survey of the integument structure in larvae, pupae and adults using tissue sections and hematoxylin-eosin staining, we found that the integument structure is different at each developmental stage (Figure S1). Before pupation, the larvae are covered by a layer of cuticle that decreases in thickness from the feeding stage (Figure S1A) and wandering stage (Figure S1B) to the pre-pupation stage (Figure S1C). The yellow and tanned cuticle of the pupae is hard and easily lost during tissue sectioning (Figure S1D). At approximately P-7 (day 7 of pupae), scales are clear under the pupal cuticle (Figure S1E) and adults are covered by scales (Figure S1F). Because integument morphological transformation may also accompany changes in cell structures, tubulin distribution was assayed over different developmental stages. The results show that tubulin is uniformly distributed in larval epidermal cells (Figure S2A and S2A`). After the insect enters the pre-pupation stage, tubulin begins to move toward one side of the cell (Figure S2B-S2C`). On P-7, tubulin clearly concentrates on one side of each epidermal cell (Figure S2D-S2D`), suggesting that this arrangement may be related to flight function. These results demonstrated that the insect integument changes significantly at each development stage and led us to investigate the mechanism that regulates integument development from the larval thru the adult stages.
Cell apoptosis, division and DNA duplication in the integument during metamorphosis
From the feeding stage (V-5, day 5 of 5th larval stage) to adults (A-1), B. mori body size decreases considerably () and adult scales are also produced (). Because integument development indicates that there might be changes in epidermis cell number, cell apoptosis was assayed from the feeding stage (V-5) to the adult stage (A-1). Even though insect size became noticeably shorter (), cell apoptosis was not detected before pupation ( and ). Approximately one day after pupation, a few apoptotic cells were detected in the intersegmental membrane ( and ), but many more apoptotic cells were observed in the neighboring segment ( and ). Just before P-4, very few apoptotic cells were detected ( and ). Therefore, apoptosis occurs in cells of the intersegmental membrane and segment from approximately P-1 to P-4 (). The above results are unusual because it would be anticipated that apoptotic cells would appear during the spinning stage since that is when the insect size has markedly decreased ().
Beginning with the feeding stage, DNA duplication and cell division in the epidermal cells were continuously assayed with BrdU injected into the insects to label DNA duplication in cells.Citation39 Two waves of DNA duplication were detected. One was from W-0 h (0 h after the wandering stage) to W-33 h (33 h after the wandering stage, which is also the end of the spinning stage) (Figure S3A-S3C), and another one from P-0:12 h to P-4 (Figure S3F-S3G). No DNA duplication was detected from the end of the spinning stage to 12 h after pupation (P-0: 12h). Pictures at two typical time points are shown here: W-51 h (Figure S3D) and P-0: 12 h (Figure S3E). After P-4, no DNA duplication was detected in the pupal epidermis cells (e.g. P-9:8 h; Figure S3H). The above two waves of DNA duplication are summarized in Figure S3I.
In the silkworm larvae at the 5th feeding stage, DNA duplication and cell division were not observed if wounds were made and sampled for assay 12 h later (Figure S4A-S4A`). However, DNA duplication was observed around the wounds between 24 and 48 h later (Figure S4B-S4C`), but very few cells were detected to incorporate BrdU at 72 h after the wound was initiated (Figure S4D-S4D`). A few epidermis cells were observed to divide around the wounds between 24–48 h after the wounds were made (inset in Figure S4C`), indicating that there are epidermal stem cells that can divide in the silkworm larvae. In later pupae (e.g. 24 h after a wound being made on P-7: P-7+24 h; Figure S4E and 4E`) and adults (e.g. 24 h after a wound made on A-2: A-2+24 h; Figure S4F and S4F'), no DNA duplication and cell division were observed around the wounds, indicating that there are no epidermal stem cells in the later pupal and adult stages. The above data demonstrate that cell apoptosis, cell division and DNA duplication accompany the integument development process during metamorphosis. According to the wound repair assay, there are stem cells in the larval epidermis (inset in Figure S4C) but not later pupal and adult epidermis, which subsequently raises the question of whether the scale cells develop from epidermal stem cells.
Scale formation depends on epidermal cell division
When entering the wandering stage, cell division and DNA duplication were assayed in the 7th segment (labeled as 1) and intersegmental membranes (labeled as 2) as indicated in . At W-6 h, cell division was detected in the segment but not in the intersegmental membrane according to PH3 staining () and DNA duplication was detected in both regions according to BrdU incorporation and staining (-). In the segment, BrdU-positive cells appear in pairs and in similar sizes to each other (), indicating symmetric cell division in this region. In the intersegmental membrane, there is also DNA duplication and in this region the nuclei of DNA-positive cells are not paired and are larger than those of negative cells at the assayed time (). During the wandering stage, when BrdU was injected into the larvae every 6 h, almost all cells in the segment and intersegmental membrane were labeled by BrdU (data not shown), indicating that all epidermal cells were growing in size through DNA duplication. These data demonstrate that the epidermal stem cells divide into two similar daughter cells through symmetric cell division during the wandering stage.
Figure 2. Cell division in the silkworm integument. (A) The morphology of the 7th segment (labeled as (1)) and the intersegmental membrane (labeled as (2)) between the 7th and 8th segments. (B) One wave of cell division was detected in the 7th segment after W-0 h. The arrows point to PH3-positive cells that were undergoing cell division in the 7th segment (1) but not in the intersegmental membrane (2). (C, D) Detection of DNA duplication after a pulse of BrdU with BrdU incorporation stained as green. In (C), the arrow points to BrdU-positive cells appearing in pairs. In (D), the arrow points to larger nuclei that incorporated BrdU, and the arrowheads point to small nuclei without BrdU incorporated. (E) Another wave of cell division was detected at P-1:18 h (after P-0:12 h) as indicated by PH3 staining. (F) Injection of BrdU every 6 h after P-0:0 h until the sampling time as indicated. At P-1:18 h, BrdU-positive cells appear in pairs, and some BrdU-positive cells in pairs became larger than others. (G) After a pulse of BrdU injection at P-2, BrdU was incorporated by cells with small scales produced. The BrdU-positive cells were observed as singles and not in pairs. (H) After labeling by BrdU as shown in (F), at P-3:5 h, the larger BrdU-positive cells were observed to have small scales produced. (I) A summary of the two waves of cell division in the integument. (J) The integument stem cells divide into precursor scale cells after symmetrical cell division during the wandering stage. Upon entering the pupal stage, the precursor scale cells differentiate into scale secreting cells after an asymmetrical cell division. The red bar indicates a scale. The nucleus is drawn in blue. Bar: (B-D) 35 μm; (E-H) 15 μm.
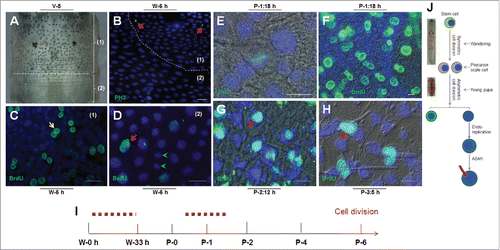
In the pupal stage, PH3 was detected in some epidermal cells at P-1:18 h, indicating the occurrence of cell division (). After careful examination, we found that cell division occurs in the young pupal integument after P-0:12 h (data not shown). At P-0:12 h, no BrdU-positive cells were detected (Figure S3E), which indicates that there is no cell division in the epidermis during the first 12 h after pupation. BrdU was then injected into young pupae every 6 h after pupation and at 12 h following the first injection (P-1:18 h), BrdU was detected in pairs (). In contrast to what was observed during the wandering stage described above (), one daughter cell was clearly larger than the other one (), which indicates an asymmetric cell division. One pulse of BrdU injection (at P-2:9 h) labeled a cell containing a small scale (), indicating that the scale-secreting cells continuously incorporate BrdU following cell division. As shown in , when BrdU was injected every 6 h, at P-3:5 h, the larger BrdU-positive cells were shown to have scales (). Thus, after the asymmetrical division, one daughter cell becomes the scale secreting cell in which DNA is duplicated ().
At P-0:20 h, paclitaxel, which is a cell division inhibitor,Citation37 was injected into each pupa. After eclosion, almost no scales were detected on the dorsal side (). When the solvent DMSO (control) was injected, cell division was still detected in the epidermis (), but if paclitaxel was injected, no dividing cells were detected according to PH3 staining (). On P-4, BrdU was detected in scale secreting cells when DMSO was injected (), while the injection of paclitaxel decreased the size and number of BrdU-positive cells (). No scales were detected in paclitaxel treated adults. Thus, the second wave of cell division during the early pupal stage is very important for the differentiation and development of scale secreting cells ().
Figure 3. Integument cell division during early pupal stage is important to scale development. (A) Injection of paclitaxel, a cell division inhibitor, affects the formation of scales. Paclitaxel (2 μg) was injected at P-0:20 h. No scale was observed on the dorsal side. (B, C) Detection of cell division after paclitaxel injection in pupae on P-2. DMSO did not inhibit cell division as indicated by PH3 staining (B). No cell division was detected if paclitaxel was injected (C). (D, E) 72 h after paclitaxel treatment, BrdU was injected. (D) DMSO did not affect DNA duplication and scale formation. (E) Paclitaxel injection did not inhibit DNA duplication, but few scales were detected. (F) A summary of the importance of the second wave of cell division to scale formation. The red bar indicates a scale. The nucleus is drawn in blue. Bar: (A) 5 mm; (B-E) 20 μm.
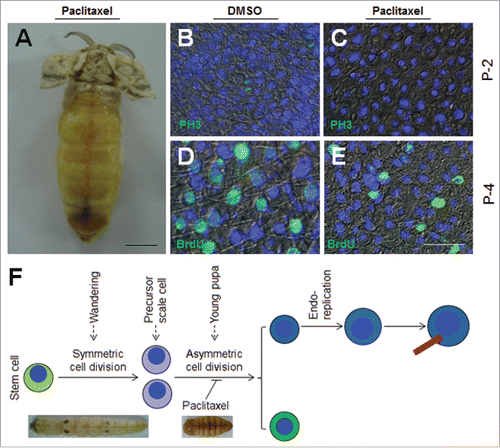
In the case of BrdU continuous injection, some smaller earlier-labeled BrdU-positive cells probably disappeared because only the larger ones were detected (). After BrdU was continuously injected (i.e., once every 6 h), the integument (P-2) was sampled by TUNEL and BrdU detection at the same time (-). Many apoptotic bodies were shown to have incorporated BrdU (). To sample the integument (without tissue section) on P-2 for direct staining of BrdU and TUNEL, we found that some smaller BrdU-positive cells were apoptotic (-). The results indicate that, after asymmetric cell division in young pupae, some non-scale secreting cells became apoptotic later (). These data demonstrate that scale cells are differentiated from scale precursor cells that are produced from epidermal stem cells during the wandering stage ().
Figure 4. Apoptosis of one daughter cell after the second wave of asymmetrical cell division. BrdU was injected at P-3:0 h. BrdU and TUNEL staining indicate DNA duplication and apoptosis respectively either by tissue section (A-D) or directly (E-H). DAPI was used to counter-stain nuclei (A, E). BrdU is labeled in green (B, F) and TUNEL is labeled in red (C, C`). These images were merged to show the co-localization of green and red fluorescence (D, H). The arrows point to the granules exhibiting the co-localization of BrdU and TUNEL signals. (I) A summary of the fate of daughter cells derived from the second wave of asymmetrical cell division. The red bar indicates a scale. The nucleus is drawn in blue. Bar: 20 μm.
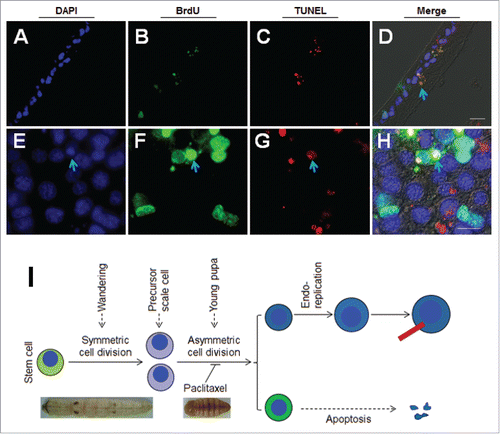
Influence of 20-hydroxyecdysone (20E) on the scale development in a time- and dose-dependent manner
During pupation, the titer of plasma 20E changes considerably and reaches a peak between P-2 and P-3.Citation40,Citation41 To determine if increasing 20E can affect integument development, different doses of 20E were injected into pupae at P-0:20 h. Compared with DMSO injection (), the injection of 5 μg of 20E had very limited influence on scale production (), but when approximately 10–15 μg of 20E was injected, scale formation was significantly deterred ( and ). In another experiment, approximately 15 μg of 20E was injected into each pupa at different times after pupation. When 20E was injected at P-0:20 h, almost no scales were detected on the dorsal side as shown (). However, scales were shorter if the same amount of 20E was injected at P-1:6 h and P-1:16 h, respectively ( and ). Almost no influence on scale formation was detected when 20E was injected either on P-3 () or the time after P-3 (data not shown). On adult wings, there are also many scales (). When 20E was injected, the change of scales observed for the wings were very similar to those observed on the integuments, suggesting that the mechanism that regulates scale production on the integuments and wings is similar. It is apparent that 20E can affect scale cell development in a time- and dose-dependent manner.
Figure 5. Influence of 20E on scale formation is stage- and dose-dependent. (A) Influence of 20E on scale formation is dose-dependent. Different amounts of 20E were injected into each pupa at P-0:20 h. And at A-1, the adults were compared. When the amount of 20E injected was increased, the scales became short. (B) Influence of 20E on scale formation is stage-dependent. Approximately 15 μg of 20E was injected into each pupa at the indicated time points. Injection of 20E at P-0:20 h (a) significantly inhibited the formation of scales. When the injection time was postponed, scale lengths were longer (b, c, d). Scale formation was dose-dependently influenced. (C) Comparison of BrdU-incorporation at P-2 (a-d) and nuclei sizes at P-4 (e-h) after different amounts of 20E were injected at P-0:20 h. BrdU was injected 3 h ahead of sampling time. With 20E increased, the number of BrdU-positive cells was decreased at P-2 (a-d). At P-4, large nuclei were observed with DMSO injection (e). When different amounts of 20E were injected, there were fewer large nuclei (f-h). (D) Detection of DNA duplication at different time points after injection of DMSO (a-d) or 15 μg of 20E (e-h) at P-0:20 h. At P-1:12 h, the number of BrdU-positive cells in pairs were almost the same between the DMSO (a) and 20E (e) injection. At P-2, more BrdU-positive cells were observed in the DMSO-injected (b) than in 20E-injected larvae (f). Bar: (A-B) 5 mm; (C-D) 70 μm.
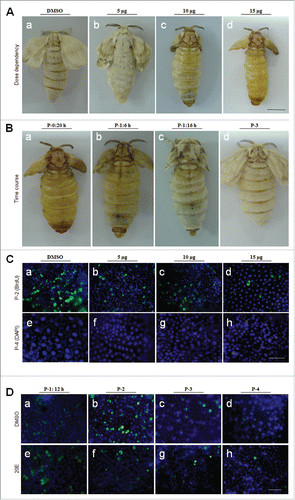
20E injection inhibits DNA duplication but not cell division in the integuments of young pupae
Because we wanted to know if DNA duplication is influenced by 20E injection, different doses of 20E were injected as shown in , followed by one pulse of BrdU labeling at 3 h later. On P-2, DNA duplication was inhibited if the injected 20E was increased ( to ). On P-4, the scale cells have larger nuclei due to normal DNA duplication when the control DMSO was injected (). However, when 20E was injected, larger nuclei were not very obvious ( to ). At P-0:20 h, 15 μg of 20E was injected into each pupa, and the integuments were dissected for BrdU (one pulse, 3 h before sampling) detection on P-1:12 h, P-2, P-3 and P-4, respectively (). When DMSO was injected, BrdU-positive cells appeared in pairs at P-1:12 h (). On P-2, one BrdU-positive cell that appeared in pairs became larger after DMSO injection (). On P-3 and P-4, BrdU-positive cells were decreased ( and ). When 20E was injected beforehand, at P-1:12 h, BrdU-positive cells appeared in pairs as was observed when DMSO was injected (compare and ). However, on P-2, very few BrdU-positive cells were detected (), which indicates that one time of 20E injection (at P-0: 20 h) can influence DNA duplication for a long time. On P-3 and P-4, BrdU-positive cells were not significantly different in number in the 20E injected as compared with the DMSO injection ( and ). These results show that a sudden increase in 20E titer in early pupae inhibits actual scale cell size.
20E (15 μg/pupa) then was injected into each pupa at P-0:20 h as shown in . At P-1:12 h, the integuments were sampled for detecting cell division. The results show that stem cells in the integuments were still labeled with PH3 (). At P-2:12 h, the integuments from pupae that had received a DMSO or 20E injection were then compared. At this stage, the sizes of epidermal cells from pupae receiving a 20E injection were smaller and the scales were also very short when compared with those from pupae with a DMSO injection (). After 20E was injected, the transcription of several genes related to the cell cycle and DNA duplication were down-regulated at 15 h (). These data demonstrate that 20E injection in early pupae can affect DNA duplication by inhibiting it but will not impact cell division ().
Figure 6. Injection of 20E did not inhibit integument cell division but did inhibit DNA duplication. Approximately 15 μg of 20E was injected into each pupa at P-0: 20 h. (A-C) Detection of integument cell division after PH3 staining. Integument cell division was not influenced by 20E injection as assayed at P-1:12 h. (D-I) 20E inhibition of DNA duplication and scale formation. At P-2:12 h, new scales were compared between DMSO (D-F) and 20E (G-I) injection. The arrows point to scales that appear in different sizes. (J) Different amounts of 20E were injected into pupae at P-0:20 h. Approximately 18 h later, integuments were sampled for qRT-PCR. Injection of 20E affected the transcription of cell cycle related genes. Each column represents the mean ± S.E.M. of at least three independent experiments (K) A summary of the development of scale-secreting cells. A sudden increase of 20E in young pupae deters scale production. Bar: 35 μm.
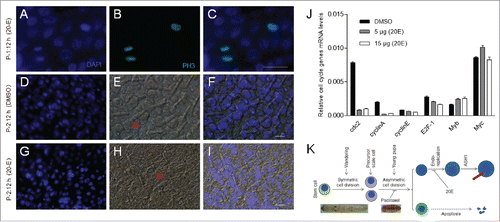
Increasing 20E deters the scale production
The above data demonstrate that the scale-secreting cells can still be differentiated and develop when 20E was injected into young pupae. However, scale growth seems to be deterred. When approximately 15 μg of 20E was injected into each pupa, the wings and bodies were covered by very small scales ( and ), which indicates that 20E affects scale production in integuments and wings by a similar mechanism. Because A achaete–scute (AS-C) in Drosophila and the AS-C homolog ASH1 in lepidopteran insects are expressed in scale precursor cells in the wings,Citation33 We assessed the influence of 20E on ASH1 production in the silkworm. ASH1 transcription was initially assayed at different developmental stages. The results indicate that ASH1 transcription increases within 24 h after pupation, and then decreases (). Approximately 15 μg of 20E was injected at P-0:20 h as shown in . At P-1:0 h, the transcription of ASH1 was significantly decreased compared with DMSO injection (). This decrease was also observed at other time points (), indicating again that the influence of 20E injection is long lasting.
Figure 7. 20E inhibits scale formation via BR-C Z2 which down-regulates the expression of scale protein ASH1. (A) ASH1 transcription during different developmental stages. Silkworm larvae at IV-M (4th molting stage), V-1 and different pupal stages were assayed. After pupation, the transcription of ASH1 is up-regulated within the first 24 h, with the level of ASH1 decreasing after P-1:0 h. (B) Injection of 20E significantly inhibited ASH1 transcription. 20E was injected at P-0:20 h and integuments were sampled at the indicated stages for comparison. 20E significantly decreased ASH1 transcription at each stage. (C) Addition of 20E significantly inhibited ASH1 expression in vitro. The ASH1 promoter was combined with the luciferase gene in the pGL3 plasmid for over-expression in S2 cells. With 20E added, luciferase activity was significantly decreased when compared with DMSO treatment. (D) BR-C Z2, BR-CZ2-GFP and GFP (control) were co-expressed with the above pGL3 plasmid, respectively. Over-expression of BR-C Z2 or BR-C Z2-GFP significantly inhibited ASH1 expression. Each column represents the mean ± S.E.M. of at least three independent experiments. (E) A model of scale formation. An integument stem cell divides into two similar daughter cells after symmetric cell division during the wandering stage. In young pupae, the precursor scale cells then experience asymmetric cell division. One cell undergoes apoptosis and the other one experiences several rounds of endoreplication to become the scale secreting cell. When the titer of 20E is increased ahead of the appropriate stage, DNA duplication and scale production in scale secreting cells are inhibited. The red bar indicates a scale. The nucleus is drawn in blue.
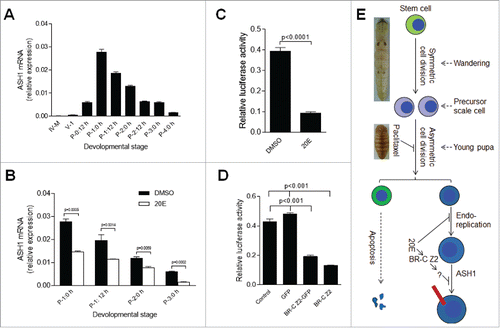
In the ASH1 promoter sequence, there are two BR-C Z2 binding domains (data not shown). In B. mori, and Drosophila, BR-CZ is an ecdysone response element.Citation18,Citation42,Citation43 The transcription of BR-C Z2 was enhanced when 20E was injected (Figure S5). In the silkworm wing discs, BR-C Z2 binds to BMWCP10 to synergistically regulate its expression upon increasing 20E titer.Citation27 Because we wanted to know whether BR-C Z2 regulates ASH1 production, we performed a luciferase activity assay. The promoter of ASH1 was cloned and placed in front of the luciferase reporter gene. The above ASH1 promoter sequence was inserted into pGL3 plasmid for expression in S2 cells to detect ASH1 transcription activity. Compared with DMSO, the addition of 20E decreased the luciferase activity significantly (), which is consistent with results from 20E injection in vivo (). Next, BR-C Z2, BR-CZ2-GFP and GFP genes were cloned separately into pAC5.1. These plasmids, including a blank pAC5.1, were co-expressed with the above pGL3 plasmid containing the ASH1 promoter and reporter gene, respectively. The expression of BR-CZ2 or BR-CZ2-GFP significantly decreased the luciferase activity (). In order to verify whether BR-C Z2 can bind to the ASH1 promoter, an electrophoretic mobility shift assay (EMSA) was performed. While we did not detect any direct binding (data not shown), the production of the BR-C Z2 transcription factor may induce the expression of some other protein(s) that can deter ASH1 expression. Consequently, these results suggest that when the 20E titer is gradually increased in haemolymph, enhanced BR-CZ2 expression results in the production of an unknown inhibitor that subsequently decreases ASH1 and scale protein production ().
Discussion
Many investigations have studied the development of tissues, like fat bodies and midguts, during ecdysis and metamorphosis in different insect species. While the integument, which has different morphology during the metamorphosis of each developmental stage ( and Figure S1), is also an important organ that protects insects from excessive water evaporation, physical abrasion and pathogen invasion.Citation1,Citation6,Citation7 There are few studies describing development of the insect integument and scale production during metamorphosis. Using the silkworm B. mori as a model, we demonstrated that adult scales are differentiated from larval integument stem cells after a symmetric cell division during the wandering stage. After that the precursor scale cells will experience asymmetric cell division to produce scale cells in young pupae. However, the DNA duplication and scale production in the scale secreting cells are under the control of 20E ().
During metamorphosis, insect size changes significantly. Before pupation, silkworm insects shorten to become almost half of the feeding stage size (). Prior to this study, we concluded that there should be large numbers of apoptotic cells occurring around metamorphosis due to the morphological changes of the whole silkworm (). However, apoptosis did not occur until pupal stage on the epidermis ( and Figure S7). Furthermore, one wave of cell division was detected between the beginning of the wandering stage (W-0 h) to the end of the spinning stage (W-33 h) ( and Figure S7). In addition, DNA duplication also occurred in epidermis cells (Figure S3 and Figure S7). These data indicate that the number and sizes of epidermis cells are still increased during the spinning period. The reasons that insect size decreases are probably due to non-feeding and evacuation of gut contents, as well as due to spinning that can empty the silk gland. During the spinning stage, some tissues like the midgut and silk gland undergo apoptosis.Citation44,Citation45 Therefore, insects become smaller before pupation even though the epidermis cells increase in size and number.
Integument structures change greatly during metamorphosis. Tissue sectioning followed by haematoxylin and eosin staining demonstrates that there is a large change in integuments after pupation (Figure S1). The white larval cuticle became thinner and was replaced by the yellow one ( and Figure S1A-S1D). At approximately P-2:12 h, small scales were formed (), which indicates that the integument may be remodeled around this time period. The repair of physical wounds shows that there are epidermal cells that can divide in the larval stage but not later on in the pupal and adult stages (Figure S4), indicating that there are stem cells in the larval integument. During metamorphosis, two waves of epidermal cell division were observed ( and Figure S7). The first one occurs between W-0 h to W-33 h ( and Figure S7). When cell division was blocked via the injection of an inhibitor like paclitaxel during this period, the larvae could not pupate (data not shown). Therefore, the first wave of cell division is important to integument development. We note that it is possible that paclitaxel may inhibit cell division in other important tissues that also affect insect development. The second wave of cell division occurs between P-0:12 h to P-2:0 h. Interruption of the cell division during this period affects scale formation (). However, different from the first wave of cell division, the adult can still lay eggs (data not shown), which indicates that the second wave of epidermal cell division has no influence on development but affects scale formation. Using BrdU to label DNA duplication, we found that the two daughter cells derived from stem cell division incorporated BrdU, which made them appear in pairs during the wandering stage (). Upon entering the pupal stage, if BrdU was injected for several times, one daughter cell became larger after several rounds of DNA duplication (). Prior to this study, we concluded that there should be large numbers of apoptotic cells occurring around metamorphosis due to the morphological changes of the whole silkworm (). However, apoptosis did not occur until pupal stage on the epidermis ( and Figure S7).
In the life cycle, 20E and JH coordinately cooperate to regulate insect growth and development.Citation3,Citation8,Citation9 However, after pupation, methoprene, a JH analog, does not influence integument development in pupae (Figure S6). Upon pupation, the titer of 20E gradually increases in haemolymph in vivo and may regulate DNA duplication in epidermal cells and scale formation. During metamorphosis, cell cycle related genes are activated, which are also dependent on ecdysone signaling.Citation46 In the wing discs of B. mori, cycB, cycD, cycE and myc were all up-regulated upon 20E addition in vitro.Citation41 Ecdysone signals regulate the cell cycle in insects in a complicated mechanism.Citation47-50 When 20E was increased through injection, we found that the expression of many cell cycle related genes was down-regulated (). This working mechanism of 20E is different from that of paclitaxel that deterred precursor scale cells from division and differentiation into scale cells. Because Drosophila ASH and lepidopteran ASH1 (homolog of ASH) can affect bristle and scale formation,Citation22,Citation30,Citation33-35 we assessed this gene in this study. When 20E was injected, the production of ASH1 in the silkworm was significantly down-regulated (). Additional studies demonstrated that the transcription factor BR-C Z2 acts as a negative feed-back to inhibit ASH1 expression through an unknown mechanism (), showing the need for further study in the future.
Materials and methods
Insect feeding and dissection
B. mori larvae (Nistari) were reared on mulberry leaves at 25 °C under a 12-h photoperiod. In this species of silkworm, there are approximately 6 days during the 5th feeding stage noted as V-X (day X of 5th larval feeding stage), 50 h from the beginning of the wandering stage to the day before pupation noted as W-X h (X h after wandering stage), 10 days of the pupal stage noted as P-X (day X of pupal stage), and 3–4 days of the adult stage noted as A-X (day X of adult stage). In this text, P-X: Y h means at Y h of the P-X stage. To obtain samples for quantitative real-time PCR (qRT-PCR), and tissue section, silkworms were dissected in autoclaved 0.85% NaCl solution after bleeding using a needle to puncture one abdominal leg, then fat body and other tissues were carefully removed from the epidermis. In this work, unless otherwise mentioned, the integuments of the 7th and 8th segments were cut and washed three times in autoclaved 0.85% NaCl solution. For tissue sections, the integuments were fixed overnight in Carnoy's fluid at room temperature, transferred into 70% ethanol until use.Citation51 In some experiments, after tissue sectioning, the samples were stained using hematoxylin-eosin solution.
DNA duplication and cell division
Silkworms at different developmental stages were injected with 5-bromo-2'-deoxyuridine (BrdU, Invitrogen, San Diego, USA) at 10 μl/g body weight to label DNA duplication in cells. Pupae at P-0:0 h were injected with BrdU every 6 hours to label all cells undergoing DNA duplication. Three hours after BrdU injection or at the scheduled time point, the silkworm larvae and/or pupae were sampled as described above and fixed for tissue sectioning. To detect BrdU incorporated by cells, the samples were deparaffined and boiled in sodium citrate buffer (10 mM, pH 7.0) for 1 min in a microwave oven, followed by washing with PBS buffer (140 mM NaCl, 10 mM phosphate buffer, and 3 mM KCl, pH 7.4) three times. The samples were incubated with the primary antibody against BrdU (Invitrogen; 1:1000) in TBST buffer (137 mM NaCl, 2.7 mM KCl, 19 mM Tris containing 1 ml Tween 20 in each liter, pH 7.4) for three hours, washed in PBS three times, and then incubated in TBST buffer containing the secondary anti-mouse antibody conjugated with Alexa Fluor-488 or Alexa Fluor-594 for three hours. After washing, 4',6-diamidino-2-phenylindole (DAPI) was used to counterstain nuclei. Images were acquired using a fluorescence microscope with the corresponding filters (Olympus BX51, Japan). To detect cell division, the samples were permeated with 0.1% Triton for 10 min, and washed with PBS buffer three times. The primary antibody against phospho-Histone H3 (PH3, Merck; 1:1000) in TBST buffer was incubated with the samples. After that, the washing and incubation with the secondary antibody were similar to BrdU staining as described above.
Apoptosis detection
The silkworm integuments were permeated with 0.1% Triton for 10 min, and then the samples were stained using an In Situ Cell Death TMR kit (Roche) according to the manufacturer`s instructions. DAPI was used to counter-stain nuclei and all images were taken with the fluorescent microscope described above.
Quantitative real-time PCR
Total RNA was extracted from integuments at different development stages or from insects that had been injected with 20E or DMSO injection using Trizol reagent (Invitrogen) according to the manufacturer`s instructions. cDNA from total RNA (2 mg) was reverse-transcribed using a first strand cDNA synthesis kit (TOYOBO). Primers are listed in Table S1. qRT-PCR was conducted using SYBR® Green Realtime PCR Master Mix (TOYOBO) kit, initiated at 95 °C for 5 min, followed by 40 cycles of 95 °C for 30 s, 55 °C for 10 s, and 72 °C for 20 s using a Rotor-Gene 3000 (Bio-rad) system. Melting curve analysis was performed to confirm the specificity of amplification. Relative mRNA levels of different genes were normalized to Rsp7, and the △Ct method was used to analyze relative gene expression levels,Citation52 with the graphs made using GraphPad Prism 5.
Dual Luciferase Assay
A dual luciferase assay was performed to test ASH1 transcription activity and its regulation. An approximately 3 kb region of the ASH1 promoter (upstream of the transcription start site) was predicted using the promoter predictor feature of the NNPP version 2.2 (http://www.fruitfly.org/seq_tools/promoter.html), which is based on artificial neural networks.Citation53 The predicted promoter was cloned and placed in front of the Renilla luciferase reporter gene, which was inserted into the pGL3 basic luciferase reporter vector (Promega) (see Table S1 for primer information). Transient transfection in S2 cells with the reporter pGL3 vector and the reference pRL vector respectively then was performed according to the protocol of the Effectene Kit (QIAGEN).
The full length cDNA of silkworm BR-C Z2 (NCBI Reference Sequence: NM_001111333.1) then was amplified by PCR, and cloned into the pAc5.1 vector. In the same way, BR-C Z2-GFP and GFP alone were also cloned and placed into the pAc5.1 vector (see Table S1 for primer information). These plasmids were co-transfected with the pGL3 construct containing the ASH1 promoter and luciferase reporter gene into S2 cells according to the protocol of the Effectene Kit (QIAGEN).
At the scheduled time, S2 cells were harvested and washed twice in PBS. The cell pellets were suspended in the reporter Passive Lysis Buffer (Promega) and sonicated on ice. Samples were then centrifuged at 12,000 g for 2 min at 4 ℃, and the supernatants were used for the Luciferase activity assay. The enzyme activities were measured with a luminometer (Perkin Elmer) using a Dual Luciferase reporter assay system (E1910, Promega). The luciferase activity was normalized to the level of Renilla luciferase.
Disclosure of interest
The authors declare that there is no conflict of interest.
Funding
This work was supported by the National Natural Science Foundation of China under Grant 31672360 and 31472043.
References
- Reynolds SE, Samuels RI, Physiology and biochemistry of insect moulting fluid, In: Evans PD, Editor. Adv In Insect Phys. Academic Press; 1996. p. 157-232. Philadelphia, PA: Elsevier Ltd.
- Payre F, Genetic control of epidermis differentiation in Drosophila. Int J Dev Biol. 2004;48(2-3):207-15. doi:10.1387/ijdb.15272387. PMID:15272387
- Riddiford L, Hiruma K, Zhou X, Nelson CA. Insights into the molecular basis of the hormonal control of molting and metamorphosis from Manduca sexta and Drosophila melanogaster. Insect Biochem Mol Biol. 2003;33:1327-38. doi:10.1016/j.ibmb.2003.06.001. PMID:14599504
- Truman JW, Riddiford LM, The origins of insect metamorphosis. Nature 1999. 401(6752):447-52. doi:10.1038/46737. PMID:10519548
- Nijhout HF, Williams CM, Control of moulting and metamorphosis in the tobacco hornworm, Manduca sexta (L.): growth of the last-instar larva and the decision to pupate. J Exp Biol. 1974;61(2):481-91. PMID:4443740
- Han F, Lu A, Yuan Y, Huang W, Beerntsen BT, Huang J, Ling E. Characterization of an entomopathogenic fungi target integument protein, Bombyx mori single domain von Willebrand factor type C, in the silkworm, Bombyx mori. Insect Mol Biol. 2017;26(3):308-316. doi:10.1111/imb.12293. PMID:28168773
- Brey PT, Lee WJ, Yamakawa M, Koizumi Y, Perrot S, François M, Ashida M. Role of the integument in insect immunity: epicuticular abrasion and induction of cecropin synthesis in cuticular epithelial cells. Proc Natl Acad Sci U S A. 1993;90(13):6275-9. doi:10.1073/pnas.90.13.6275. PMID:8327509
- Truman JW, Riddiford LM, The morphostatic actions of juvenile hormone. Insect Biochem Mol Biol. 2007;37(8):761-70. doi:10.1016/j.ibmb.2007.05.011. PMID:17628276
- Riddiford LM, Cherbas P, Truman JW, Ecdysone receptors and their biological actions. Vitam Horm. 2000;60:1-73. doi:10.1016/S0083-6729(00)60016-X. PMID:11037621
- Gruntenko NE, Rauschenbach IY, Interplay of JH, 20E and biogenic amines under normal and stress conditions and its effect on reproduction. J Insect Physiol. 2008. 54(6):902-8;doi:10.1016/j.jinsphys.2008.04.004. PMID:18511066
- Dubrovsky EB, Hormonal cross talk in insect development. Trends Endocrinol Metab. 2005;16(1):6-11. doi:10.1016/j.tem.2004.11.003. PMID:15620543
- Chen L, Zhu J, Sun G, Raikhel AS. The early gene Broad is involved in the ecdysteroid hierarchy governing vitellogenesis of the mosquito Aedes aegypti. J Mol Endocrinol. 2004;33(3):743-61. doi:10.1677/jme.1.01531. PMID:15591032
- Pierceall WE, Li C, Biran A, Miura K, Raikhel AS, Segraves WA. E75 expression in mosquito ovary and fat body suggests reiterative use of ecdysone-regulated hierarchies in development and reproduction. Mol Cell Endocrinol. 1999;150(1-2):73-89. doi:10.1016/S0303-7207(99)00022-2. PMID:10411302
- Sun G, Zhu J, Li C, Tu Z, Raikhel AS. Two isoforms of the early E74 gene, an Ets transcription factor homologue, are implicated in the ecdysteroid hierarchy governing vitellogenesis of the mosquito, Aedes aegypti. Mol Cell Endocrinol. 2002;190(1-2):147-57. doi:10.1016/S0303-7207(01)00726-2. PMID:11997188
- Gonzy G, Pokholkova GV, Peronnet F, Mugat B, Demakova OV, Kotlikova IV, Lepesant JA, Zhimulev IF. Isolation and characterization of novel mutations of the Broad-Complex, a key regulatory gene of ecdysone induction in Drosophila melanogaster. Insect Biochem Mol Biol. 2002;32(2):121-32. doi:10.1016/S0965-1748(01)00097-2. PMID:11755053
- Liu X, Dai F, Guo E, Li K, Ma L, Tian L, Cao Y, Zhang G, Palli SR, Li S. 20-Hydroxyecdysone (20E) primary response gene E93 Modulates 20E signaling to promote Bombyx larval-pupal metamorphosis. J Biol Chem. 2015;290(45):27370-83. doi:10.1074/jbc.M115.687293. PMID:26378227
- de Rosny E, de Groot A, Jullian-Binard C, Gaillard J, Borel F, Pebay-Peyroula E, Fontecilla-Camps JC, Jouve HM. Drosophila nuclear receptor E75 is a thiolate hemoprotein. Biochemistry. 2006;45(32):9727-34. doi:10.1021/bi060537a. PMID:16893174
- Karim FD, Guild GM, Thummel CS, The Drosophila Broad-Complex plays a key role in controlling ecdysone-regulated gene expression at the onset of metamorphosis. Development. 1993;118(3):977-988. PMID:8076529
- Muramatsu D, Kinjoh T, Shinoda T, Hiruma K The role of 20-hydroxyecdysone and juvenile hormone in pupal commitment of the epidermis of the silkworm, Bombyx mori. Mech Dev. 2008;125(5-6):411-20. doi:10.1016/j.mod.2008.02.001. PMID:18331786
- Uhlirova M, Foy BD, Beaty BJ, Olson KE, Riddiford LM, Jindra M. Use of Sindbis virus-mediated RNA interference to demonstrate a conserved role of Broad-Complex in insect metamorphosis. Proc Natl Acad Sci U S A. 2003;100(26):15607-15612. doi:10.1073/pnas.2136837100. PMID:14668449
- Zhou B, Riddiford LM, Hormonal regulation and patterning of the broad-complex in the epidermis and wing discs of the tobacco hornworm, Manduca sexta. Dev Biol. 2001;231(1):125-37. doi:10.1006/dbio.2000.0143. PMID:11180957
- Kiss I, Beaton AH, Tardiff J, Fristrom D, Fristrom JW. Interactions and developmental effects of mutations in the Broad-Complex of Drosophila melanogaster. Genetics 1988;118(2):247-259. PMID:3129334
- Kayukawa T, Jouraku A, Ito Y, Shinoda T. Molecular mechanism underlying juvenile hormone-mediated repression of precocious larval-adult metamorphosis. Proc Natl Acad Sci U S A. 2017;114(5):1057-1062. doi:10.1073/pnas.1615423114. PMID:28096379
- Truman JW, Riddiford LM, Endocrine insights into the evolution of metamorphosis in insects. Annu Rev Entomol. 2002;47(1):467-500. doi:10.1146/annurev.ento.47.091201.145230. PMID:11729082
- Ali MS, Iwanaga M, Kawasaki H, Ecdysone-responsive transcription factors determine the expression region of target cuticular protein genes in the epidermis of Bombyx mori. Dev Genes Evol. 2012;222(2):89-97. doi:10.1007/s00427-012-0392-x. PMID:22460818
- Ali MS, Wang HB, Iwanaga M, Kawasaki H. Expression of cuticular protein genes, BmorCPG11 and BMWCP5 is differently regulated at the pre-pupal stage in wing discs of Bombyx mori. Comp Biochem Physiol B Biochem Mol Biol. 2012;162(1-3):44-50. doi:10.1016/j.cbpb.2012.02.001. PMID:22366249
- Wang HB, Nita M, Iwanaga M, Kawasaki H. betaFTZ-F1 and Broad-Complex positively regulate the transcription of the wing cuticle protein gene, BMWCP5, in wing discs of Bombyx mori. Insect Biochem Mol Biol. 2009;39(9):624-33. doi:10.1016/j.ibmb.2009.06.007. PMID:19580866
- Shahin R, Iwanaga M, and Kawasaki H, Cuticular protein and transcription factor genes expressed during prepupal-pupal transition and by ecdysone pulse treatment in wing discs of Bombyx mori. Insect Mol Biol. 2016;25(2):138-52. doi:10.1111/imb.12207. PMID:26748620
- Garcia-Bellido A, de Celis JF, The complex tale of the achaete-scute complex: a paradigmatic case in the analysis of gene organization and function during development. Genetics. 2009;182(3):631-9. doi:10.1534/genetics.109.104083. PMID:19622761
- Garcia-Bellido A, Genetic analysis of the Achaete-Scute System of Drosophila melanogaster Genetics. 1979;91(3):491-520. PMID:17248895
- Campuzano S, Balcells L, Villares R, Carramolino L, García-Alonso L, Modolell J, Excess function hairy-wing mutations caused by gypsy and copia insertions within structural genes of the achaete-scute locus of Drosophila. Cell. 1986;44(2):303-12. doi:10.1016/0092-8674(86)90764-6. PMID:3002632
- Balcells L, Modolell J, and Ruiz-Gomez M, A unitary basis for different Hairy-wing mutations of Drosophila melanogaster. EMBO J. 1988;7(12):3899-906. PMID:3145198
- Galant R, Skeath JB, Paddock S, Lewis DL, Carroll SB. Expression pattern of a butterfly achaete-scute homolog reveals the homology of butterfly wing scales and insect sensory bristles. Curr Biol. 1998;8(14):807-13. doi:10.1016/S0960-9822(98)70322-7. PMID:9663389
- Zhou Q, Tang S, Chen Y, Yi Y, Zhang Z, Shen G. A scaleless wings mutant associated with tracheal system developmental deficiency in wing discs in the silkworm, Bombyx mori. Int J Dev Biol. 2004;48(10):1113-7. doi:10.1387/ijdb.041845qz. PMID:15602697
- Zhou QX, Li YN, Shen XJ, Yi YZ, Zhang YZ, Zhang ZF. The scaleless wings mutant in Bombyx mori is associated with a lack of scale precursor cell differentiation followed by excessive apoptosis. Dev Genes Evol. 2006;216(11):721-6. doi:10.1007/s00427-006-0091-6. PMID:16773336
- Shao HL, Zheng WW, Liu PC, Wang Q, Wang JX, Zhao XF, Establishment of a new cell line from lepidopteran epidermis and hormonal regulation on the genes. PLoS One 2008;3(9):e3127. doi:10.1371/journal.pone.0003127. PMID:18769621
- Hatt PJ, Moriniere M, Oberlander H, Porcheron P. Roles for insulin and ecdysteroids in differentiation of an insect cell line of epidermal origin. In Vitro Cell Dev Biol Anim. 1994;30a(10):717-20. doi:10.1007/BF02631276. PMID:7842173
- Caruelle JP, Cassier P, Hormonal stimulation and in vitro cellular differentiation in the integument of the desert locust Schistocerca gregaria (author's transl). Arch Anat Microsc Morphol Exp. 1979;68(2):127-38. PMID:533279
- Ling E, Shirai K, Kanekatsu R, Kobayashi Y, Tu Z, Funayama T, Watanabe H, Kiguchi K. Why does hemocyte density rise at the wandering stage in the silkworm, Bombyx mori? J Insect Biotechnol Sericol. 2003;72:101-9.
- Satake S, Kaya M, Sakurai S, Hemolymph ecdysteroid titer and ecdysteroid-dependent developmental events in the last-larval stadium of the silkworm, Bombyx mori: role of low ecdysteroid titer in larval-pupal metamorphosis and a reappraisal of the head critical period. J Insect Physiol. 1998;44(10):867-81. doi:10.1016/S0022-1910(98)00075-4. PMID:12770423
- Moriyama M, Osanai K, Ohyoshi T, Wang HB, Iwanaga M, Kawasaki H. Ecdysteroid promotes cell cycle progression in the Bombyx wing disc through activation of c-Myc. Insect Biochem Mol Biol. 2016;70:1-9. doi:10.1016/j.ibmb.2015.11.008. PMID:26696544
- Nishita Y and Takiya S, Structure and expression of the gene encoding a Broad-Complex homolog in the silkworm, Bombyx mori. Gene. 2004;339:161-72. doi:10.1016/j.gene.2004.06.039. PMID:15363856
- Reza AM, Kanamori Y, Shinoda T, Shimura S, Mita K, Nakahara Y, Kiuchi M, Kamimura M, Hormonal control of a metamorphosis-specific transcriptional factor Broad-Complex in silkworm. Comp Biochem Physiol B Biochem Mol Biol. 2004;139(4):753-61. doi:10.1016/j.cbpc.2004.09.009. PMID:15581808
- Xu Q, Lu A, Xiao G, Yang B, Zhang J, Li X, Guan J, Shao Q, Beerntsen BT, Zhang P, et al., Transcriptional profiling of midgut immunity response and degeneration in the wandering silkworm, Bombyx mori. PLoS ONE. 2012;7(8):e43769. doi:10.1371/journal.pone.0043769. PMID:22937093
- Tsuzuki S, Iwami M, Sakurai S, Ecdysteroid-inducible genes in the programmed cell death during insect metamorphosis. Insect Biochem Mol Biol. 2001;31(4-5):321-31. doi:10.1016/S0965-1748(00)00124-7. PMID:11222941
- Li TR, White KP, Tissue-specific gene expression and ecdysone-regulated genomic networks in Drosophila. Dev Cell. 2003;5(1):59-72. doi:10.1016/S1534-5807(03)00192-8. PMID:12852852
- Edgar BA, Lehner CF, Developmental control of cell cycle regulators: a fly's perspective. Science. 1996;274(5293):1646-52. doi:10.1126/science.274.5293.1646. PMID:8939845
- Ninov N, Manjon C, Martin-Blanco E, Dynamic control of cell cycle and growth coupling by ecdysone, EGFR, and PI3K signaling in Drosophila histoblasts. PLoS Biol. 2009;7(4):e1000079. doi:10.1371/journal.pbio.1000079. PMID:19355788
- Stevens B, Alvarez CM, Bohman R, O'Connor JD, An ecdysteroid-induced alteration in the cell cycle of cultured Drosophila cells. Cell 1980;22(3):675-82. doi:10.1016/0092-8674(80)90543-7. PMID:6780197
- Cranna N, Quinn L, Impact of steroid hormone signals on Drosophila cell cycle during development. Cell Div. 2009;4:3. doi:10.1186/1747-1028-4-3. PMID:19154610
- Shao Q, Yang B, Xu Q, Li X, Lu Z, Wang C, Huang Y, Söderhäll K, Ling E. Hindgut innate immunity and regulation of fecal microbiota through melanization in insects. J Biol Chem. 2012;287(17):14270-9. doi:10.1074/jbc.M112.354548. PMID:22375003
- Yang B, Huang W, Zhang J, Xu Q, Zhu S, Zhang Q, Beerntsen BT, Song H, Ling E, Analysis of gene expression in the midgut of Bombyx mori during the larval molting stage. BMC Genomics 2016;17(1):866. doi:10.1186/s12864-016-3162-8. PMID:27809786
- Reese MG, Application of a time-delay neural network to promoter annotation in the Drosophila melanogaster genome. Comput Chem. 2001;26(1):51-6. doi:10.1016/S0097-8485(01)00099-7. PMID:11765852