ABSTRACT
DNA replication checkpoint (Mec1-Mrc1-Rad53 in budding yeast) is an evolutionarily conserved surveillance system to ensure proper DNA replication and genome stability in all eukaryotes. Compared to its well-known function as a mediator of replication checkpoint, the exact role of Mrc1 as a component of normal replication forks remains relatively unclear. In this study, we provide in vitro biochemical evidence to support that yeast Mrc1 is able to enhance the activity of DNA polymerase ϵ (Pol ϵ), the major leading strand replicase. Mrc1 can selectively bind avidly to primer/template DNA bearing a single-stranded region, but not to double-stranded DNA (dsDNA). Mutations of the lysine residues within basic patch 1 (BP1) compromise both DNA binding and polymerase stimulatory activities. Interestingly, Mrc1-3D, a mutant mimicking phosphorylation by the Hog1/MAPK kinase during the osmotic stress response, retains DNA binding but not polymerase stimulation. The stimulatory effect is also abrogated in Mrc1 purified from cells treated with hydroxyurea (HU), which elicits replication checkpoint activation. Taken together with previous findings, these results imply that under unperturbed condition, Mrc1 has a DNA synthesis stimulatory activity, which can be eliminated via Mrc1 phosphorylation in response to replication and/or osmotic stresses.
Introduction
It is one of the fundamental tasks for all living beings to maintain their genomic integrity. During the course of genome replication in each cycle, cells are inevitably challenged by either intrinsic or extrinsic replication stress including unusual DNA structures, DNA lesions, protein barriers, oncogene-induced aberrant replication, insufficient dNTP or histone supplies, replication-transcription collisions and so on [Citation1–4]. Therefore, besides precisely spatio-temporally controlled DNA replication per se, eukaryotic cells have developed a complicated safeguard system called the replication (or the S phase) checkpoint to deal with these challenges and thus ensure proper fork progression until the entire genome is replicated [Citation5–11].
Replication stress may cause the functional uncoupling of helicase and polymerase activities Citation[12,13]. This eventually leads to the accumulation of excess RPA-coated single-stranded DNA (ssDNA), the major signal for activation of a conserved Mec1/ATR-Mrc1/Claspin-Rad53/Chk1 pathway [Citation14,15]. The main downstream effects of the replication checkpoint include: 1, stabilization of stalled forks to prevent fork collapse; 2, cell cycle arrest to allow for removal of stress and/or DNA repair; 3, inhibition of late origin firing; 4, stress-induced transcription; 5, resolving replication-transcription conflicts; 6, fork repair/restart once obstacles are removed Citation[16,17].
Mrc1 (yeast ortholog of vertebrate Claspin) was originally discovered as a mediator of replication checkpoint signaling between the sensor protein kinase Mec1 and the effector kinase Rad53 [Citation18–20]. However, further studies demonstrated that it has additional functions. Mrc1/Claspin may participate in the replication initiation stage through facilitating recruitment of the Dbf4-dependent kinase (DDK) [Citation21,22]. Mrc1 is also required for maximal rates of DNA synthesis in a reconstituted yeast DNA replication system in vitro and also in vivo [Citation23–27]. These results imply an active role for Mrc1 in promoting synthesis activity or replication fork pausing/stabilization [Citation28]. Mrc1 is a central component of normal replisome, associating with the holo-helicase CMG (Cdc45-MCM-GINS) and the major leading strand replicase Pol ϵ (Pol2-Dpb2-Dpb3-Dpb4) [Citation29]. Both its N- and C-termini interact independently with Pol2, the catalytic subunit of Pol ϵ. Although the Mrc1N-mediated interaction is abolished when Mrc1 is phosphorylated by Mec1 when cells are treated with ribonucleotide reductase inhibitor HU, it still serves as an integrator of replisome through the Mrc1C interaction. In the absence of Mrc1, the chromatin association of Pol2 is partially compromised as evidence by ChIP assays. Intriguingly, when yeast cells suffer osmotic stress, Mrc1 is phosphorylated by Hog1/MAPK, which results in decreased Mrc1-Pol2 interaction and Cdc45 loading [Citation30]. These in turn delays both origin firing and fork progression. This mechanism is believed to be critical for cells to avoid collisions between replication and bursting transcription. These findings implicate that the physical interactions between Mrc1 and Pol ϵ function not only as an integral scaffold of replisome but also as a regulator of catalysis.
In this study, we report a DNA polymerase regulatory role of yeast Mrc1, which is further modulated through its phosphorylation. Purified Mrc1 is able to enhance the synthesis activity of Pol ϵ in vitro. This stimulation activity requires both N- and C-termini of Mrc1. Mrc1 can selectively bind single-stranded DNA (ssDNA) but not dsDNA substrates. Mutation of three lysine residues within basic patch 1 (BP1) markedly reduces both ssDNA binding and DNA polymerase stimulatory efficacy. Intriguingly, an Mrc1-3D mutant mimicking phosphorylation by Hog1/MAPK kinase under osmotic stress retains DNA binding but not polymerase stimulation activity. Similarly, HU-triggered Mrc1 phosphorylation by Mec1 kinase abrogates the polymerase stimulation as well. These in vitro biochemical results may help us to understand the multiple physiological functions of Mrc1 in regulation of replication fork progression under both normal and challenged conditions.
Results and discussion
Mrc1 enhances DNA polymerase activity of Pol ϵ in vitro
Based on our previous findings that Mrc1 connects Pol ϵ with the helicase core complex Mcm2-7 at the leading strand, we hypothesized that Mrc1 may modulate the activity of Pol ϵ. To test this possibility, we first overexpressed and purified 4-subunit Pol ϵ from S. cerevisiae () and recombinant Mrc1 proteins from E. coli (), respectively. DNA polymerase activity of Pol ϵ or Pol2 was measured as 3H-dTMP incorporation into a poly(dA)-oligo(dT) substrate (). Surprisingly, even after two successive affinity purification, we noticed that Mrc1 protein was still contaminated with moderate DNA polymerase activity (data not shown). However, if we removed nucleic acids with DNase I prior to affinity chromatography, DNA polymerase contaminants in Mrc1 could be successfully eliminated (). With this, we set out to test the effect of Mrc1 on Pol ϵ. As shown in , the polymerase activity of Pol ϵ on a poly(dA)-oligo(dT) primer template increased more than 100% in the presence of an equimolar concentration of Mrc1 in vitro.
Figure 1. Mrc1 stimulates the DNA polymerase activity of Pol ϵ in vitro. (A) Purification of the Pol ϵ complex containing the catalytic Pol2, and accessory Dpb2, Dpb3 and Dpb4 subunits from yeast. See Experimental Procedures for details. (B) Purification of recombinant GST-Mrc1-His6 through two sequential affinity chromatography as described in Experimental Procedures. (C) The 3H-dTMP incorporation by Pol ϵ is significantly increased in the presence of full-length Mrc1 protein. DNA Pol ϵ (1 nM) was incubated with poly(dA)-oligo(dT) in a total volume of 60 μL at 37°C for 30min. Mrc1 or its fragment Mrc1N (1-567)/Mrc1C(568-1096) (1 nM) was added to the reactions as indicated. The 3H-dTMP incorporation was measured by scintillation counting. Averages from at least three independent experiments ± SD were shown. Statistical significance was measured using Student's t-test. **P < 0.01; n.s., not significant. (D) The DNA polymerase activity of Pol2 can be stimulated by Mrc1 protein as well. The experiments were performed as above except that Pol2 was used instead of Pol ϵ. Mean ± SD are shown from three independent experiments. Statistical significance was measured using Student's t-test. **P < 0.01; n.s., not significant. (E) The stimulation effect of Mrc1 was validated by primer extension assays. The increasing amount of Mrc1 protein (1-100 nM) was added into the primer extension reactions containing 1 nM Pol ϵ at 37°C. The reactions were quenched and subjected to 8% PAGE containing 7M urea followed by autoradiography. (F) Quantification of two biological repeats of (E) using Bio-Rad Quantity One.
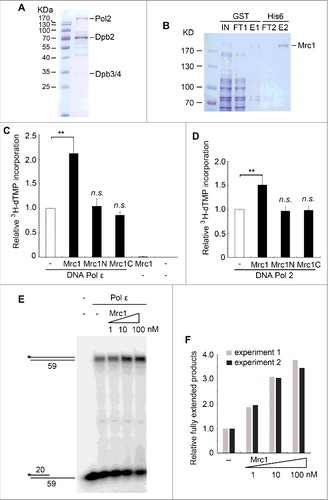
Given that both the N- and C- termini of Mrc1 interact with Pol2 [Citation29], we next examined which domain(s) are responsible for Pol ϵ stimulation. Neither Mrc1N(1-566) nor Mrc1C(567-1096) was able to increase the polymerase activity (), suggesting that both interaction domains are important. Moreover, DNA synthesis activity of the catalytic subunit Pol2 could be elevated by Mrc1 although to a lower extent than that of Pol ϵ (). These data suggest that Mrc1 has a DNA Pol ϵ-stimulating activity in vitro.
In order to validate the effect of Mrc1 on Pol ϵ, we then performed a primer extension assay using 32P-labelled 20-mer/59-mer as primer/template. Indeed, the fully extended products increased markedly in an Mrc1 dose-dependent manner (, ), verifying a DNA synthesis stimulatory role of Mrc1. These results are in agreement with the in vivo observations that replication forks move more slowly in mrc1Δ than in wild-type (WT) cells [Citation23–26].
Mrc1's DNA binding activity is indispensable for DNA Pol ϵ stimulation
Although Mrc1 is overall an acidic protein with theoretical pI around 5.1, it contains an evolutionary conserved helix-loop-helix motif within BP1 (a.a. 288–332), which has been proposed to bind DNA () [Citation31]. To examine whether DNA binding capability is involved in polymerase stimulation, we mutated three lysine residues (K323, K324, K328) to either alanine (Mrc1-3KA) or glutamic acid (Mrc1-3KE). Mutant proteins were expressed and recovered at similar levels to WT (). As evidenced in gel shift assays, Mrc1 WT was capable of binding ssDNA prominently in vitro (). It is noteworthy that there were two shifted bands caused by Mrc1, indicating that two or more Mrc1 molecules can bind to a DNA substrate. Nevertheless, protein-DNA complexes were barely detectable in Mrc1-3KA, suggesting that these lysine residues in BP1 are indispensable for DNA binding activity. Interestingly, Mrc1 were also able to bind either primer-template or Y-shaped DNA containing single-stranded regions ( and ), but not dsDNA (). These results indicate that like Schizosaccharomyces pombe Mrc1 and human Claspin [Citation31–34], S. cerevisiae Mrc1 is also able to preferentially bind DNA containing a single-stranded region, a typical structure found at replication forks.
Figure 2. Mrc1 has DNA binding activity. (A) A schematic view of Mrc1 and its mutant proteins. BP1 represents the basic patch 1 in the N-terminus of Mrc1 containing multiple basic residues, probably involved in DNA binding. Alanine and glutamine acid substitutions of three lysine residues (K323, K324, and K328) were designated as Mrc1-3KA and Mrc1–3KE, respectively. Mrc1-3D bears the phospho-mimetic mutations of three residues (T169, S215, and S229), which have been demonstrated to be phosphorylated by Hog1 in response to osmotic stress. (B) Silver staining of purified recombinant Mrc1 mutant proteins (3KA, 3KE, 3D) as well as WT. (C) Purified Mrc1 WT or 3KA mutant protein was incubated with 32P-labelled ssDNA at the 5′-end. After incubation at 4°C for 30min, samples were analyzed via 5% native PAGE gels followed by autoradiography. E. coli SSB (single-stranded DNA binding protein) was used as a control.
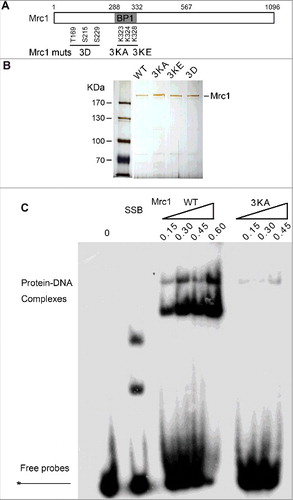
Figure 3. Mrc1 is able to bind DNA containing single-stranded regions but not dsDNA substrates. (A, B) Purified Mrc1 WT or mutant proteins (3KA, 3KE, 3D) were incubated with 32P-labelled primed ssDNA (A), dsDNA or Y-shaped duplex DNA (B) to assess their DNA binding specificity as described in .
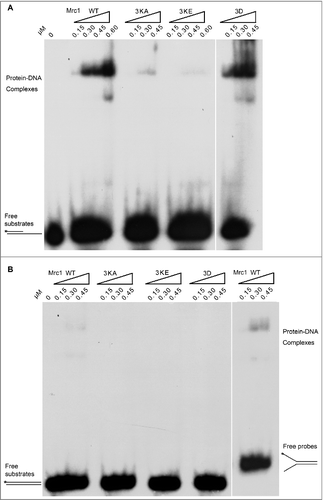
Next, we compared the polymerase stimulating activity of Mrc1 mutants with that of WT. As shown by primer extension assays, both DNA-binding defective mutants Mrc1-3KA and 3KE exhibited significantly compromised stimulation on Pol2 ( and ). These results argue for a contribution of primer-template DNA binding for Mrc1's polymerase stimulatory function.
Figure 4. The polymerase stimulation activity of Mrc1 is abrogated in the DNA-binding defective or phospho-mimetic mutants. (A) Primer extension assays were conducted as described in in the presence of increasing amount of Mrc1 WT or mutant proteins (0–100 nM). 100 nM Mrc1 proteins alone (WT, mrc1-3KA, mrc1-3KE or mrc1-3D) were examined in parallel to confirm that they do not contain any contaminating DNA polymerase activity. (B) Quantification of (A) from three independent repeats. The fully extended primers were quantified by Typhoon ImageQuant. The activity of DNA polymerase alone was normalized to 100%. Mean ± SD are shown from three independent experiments. Statistical significance was measured using Student's t-test. **P < 0.01; *P < 0.05; n.s., not significant.
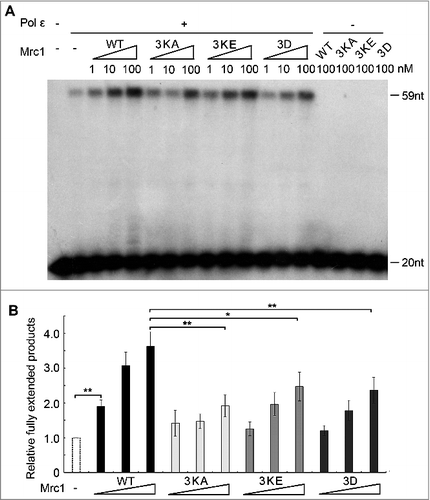
Both Hog1- and Mec1- dependent phosphorylations of Mrc1 abolish its stimulatory activity
Mrc1 can be phosphorylated by Hog1/MAPK and Mec1/ATR kinases in response to osmotic and replication stresses, respectively [Citation19,30]. This urged us to ask whether phosphorylation of Mrc1 may modulate its DNA binding or its role in stimulating DNA synthesis. First, we tested the DNA binding of a Mrc1-3D mutant, which mimics the phosphorylation status by Hog1 as reported by the Posas group [Citation30]. As shown in , the Mrc1-3D mutant retained close to WT levels of ssDNA-binding. However, like DNA-binding defective mutants, Mrc1-3D largely lost Pol2-stimulatory activity ( and ). Thus, the Mrc1-3D mutant is a separation of function mutant. Since Mrc1-3D has been demonstrated to be defective in associating with Pol2 [Citation30], our results imply that the DNA synthesis stimulation activity of Mrc1 requires both its ssDNA-binding and Pol2-associating activities.
To further study the regulation of Mrc1 by phosphorylation, we next purified endogenously phosphorylated Mrc1 from yeast cells. As the mediator of replication checkpoint, Mrc1 is phosphorylated by another kinase, Mec1, when cells encounter replication stresses such as dNTP depletion by HU [Citation19]. We immunoprecipitated myc-tagged Mrc1 from yeast cells grown in the presence or absence of HU treatment (). Mrc1 was phosphorylated after HU treatment as observed previously () [Citation29]. The immuno-purified Mrc1 had barely detectable DNA polymerase activity (). Without HU treatment, the native Mrc1 enhanced DNA polymerase activity of Pol2 to a similar extent to recombinant Mrc1. (Compare to ). However, after 200 mM HU treatment for 1 h, nearly all Mrc1 molecules migrated slowly (i.e. phosphorylated, ) and lost the stimulatory effect (). Moreover, the stimulation could be restored after λ-phosphatase treatment of Mrc1 prior to being added into the DNA polymerase reactions, verifying that the loss of stimulation is due to phosphorylation. Consistently, the endogenously purified Mrc1 displayed a potent stimulation on DNA Pol ϵ as recombinant Mrc1, which was modulated by phosphorylation as well (Compare to ). Our previous study shows that Mrc1 phosphorylation affects the interaction of Mrc1 with Pol2 N-terminus, the catalytic domain of Pol2 [Citation29]. Moreover, like the recombinant version, mrc1-3D, mimicking Hog1 phosphorylation, purified from yeast cells largely lost its stimulatory activity (). These results suggest that phosphorylation of Mrc1 by different kinases may abolish its DNA synthesis stimulatory activity under various stress conditions.
Figure 5. Mrc1 phosphorylation abolishes its stimulation effect on Pol2. (A) Endogenously myc13-tagged Mrc1 protein was precipitated by anti-myc from cells with or without HU treatment for 1 h. Mrc1 protein migrated slowly when it was phosphorylated after HU treatment. (B) Phosphorylated Mrc1 loses the stimulation effect on DNA Pol2. The DNA polymerase activity was measured as described in 1C. Precipitates from an untagged MRC1 strain were used as controls. Mean ± SD are shown from three independent experiments. Statistical significance was measured using Student's t-test. **P < 0.01; n.s., not significant. (C) A brief summary of the roles of Mrc1 under normal or stressed conditions based on previous findings and this study. Under normal conditions, Mrc1 connects Pol ϵ with CMG holo-helicase. These physical interactions can stimulate the DNA synthesis activity of Pol ϵ and thus ensure the coupling between duplex unwinding by CMG helicase and DNA synthesis by Pol ϵ. When cells experience replication or osmotic stresses, Mrc1 becomes phosphorylated by Mec1 or Hog1. This abrogates the DNA synthesis stimulation, which may contribute to fork pausing and stabilization.
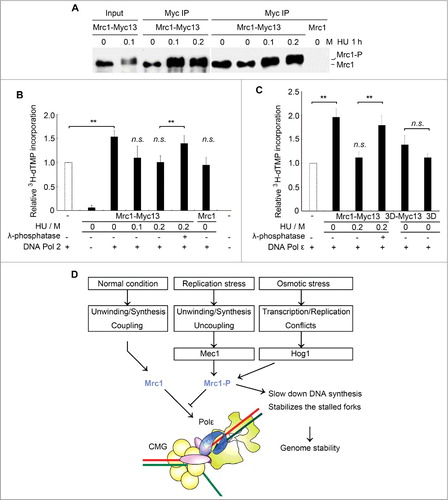
In addition to its well-established role as a mediator of the replication checkpoint, this study provides in vitro evidence to support a direct role for Mrc1 in stimulating DNA synthesis. This is in well agreement with the recent results of the Diffley group, which demonstrated that Mrc1 is the chief progression factor to boost basal in vitro DNA replication activity in a system reconstituted from purified proteins to levels consistent with in vivo rates. Two other factors, Tof1 and Csm3, can strengthen the effect, mainly through interacting with and stabilization of Mrc1 in the replisome [Citation27]. Two independent studies have shown that hTim-Tipin, which form a stable complex with Claspin, just like the Mrc1-Tof1-Csm3 complex in budding yeast, are able to facilitate the DNA polymerase activity of Pol ϵ and/or Pol δ in vitro, albeit at high molar ratios of Tim-Tipin/polymerase [Citation35,36]. It would be of interest to test the effect of Tof1 and Csm3 on Mrc1's Pol ϵ stimulation activity. More importantly, here we show that the stimulatory activity is further regulated by phosphorylation and can be eliminated by Mrc1 phosphorylation occurring in response to either replication or osmotic stresses.
The model in summarizes our work in the context of previous studies with respect to regulation of fork progression in unperturbed or perturbed conditions. Mrc1 enhances DNA synthesis through preferentially binding to primer-template DNA and the catalytic domain of Pol2 during normal replication fork progression. When cells encounter replication or osmotic stresses, Mrc1 is phosphorylated by Mec1 or Hog1 kinases [Citation19,30]. This may affect its associations with Pol2 and/or ssDNA, which in turn eliminates its DNA synthesis stimulatory activity. DNA synthesis is retarded while CMG keeps unwinding. This causes the functional uncoupling of DNA unwinding and synthesis [Citation12,13]. Eventually, ssDNA accumulates then activates the Mec1-Mrc1-Rad53 replication checkpoint cascade, which inhibits CMG unwinding and stabilizes the stalled fork [Citation14,15]. The DNA synthesis slow-down function of Mrc1 phosphorylation in response to stresses may contribute to minimizing the aberrant fork movements and/or conflicts with transcription induced to deal with stresses, which are important for maintaining genome stability.
Materials and methods
Yeast strains and basic methods
The yeast strains derived from W303-1a () were constructed basically as previously described [Citation37]. All plasmid constructs () were validated by sequencing.
Table 1. Strains used in this study.
Table 2. Plasmids used in this study.
Expression and purification of recombinant Mrc1 from E. coli
Mrc1 was cloned (pGEX4T-3-Mrc1-His6) and overexpressed in Escherichia coli BL21 (DE3) CodonPlus RIL (Stratagene). Cells were grown to an A600 of 0.8, 0.1 mM isopropyl 1-thio-β-d-galactopyranoside was added and growth continued an additional 4 h at 25°C. Cells were harvested and disrupted by Freezer/Mill 6870 (SPEX Sample Prep) in lysis buffer containing (20 mM Hepes-NaOH, pH 7.5, 300 mM NaCl, 5 mM β-mercaptoethanol, 800 nM aprotinin, 4 mM benzamidine, 2 mM phenylmethylsulfonyl fluoride (PMSF), 1% Triton X-100. Cell lysates were first precipitated with 175 mM ammonium sulfate and 0.4% polyethyleneimine. Nucleic acids were further removed by incubation with 10–20 U Dnase I at 4°C for 2 h. GST-Mrc1-6His was purified by glutathione and Ni2+ columns according to the manufacture's manuals using an ÄKTA apparatus (GE Healthcare). The eluate was concentrated to 3 mg/ml in storage buffer (15 mM Tris-HCl (pH 8.0), 150 mM NaCl, 1 mM dithiothreitol, and 15% glycerol) and stored in aliquots at -80°C.
Purification of phosphorylated Mrc1 from S. cerevisiae
Endogenous Mrc1 was labeled with a myc13 tag and partially purified via immunoprecipitation (IP) as described previously [Citation37]. Briefly, yeast cultures were treated with 0–200 mM HU for 1 h. Cells (4 × 108) were collected and lysed at 4ºC with glass beads (BeadBeater) in IP buffer [45 mM HEPES, pH 7.2, 150 mM NaCl, 1 mM EDTA, 10% glycerol, 0.2% NP-40, 2 mM dithiothreitol, 1 mM PMSF, 1 × Protease Inhibitor Cocktail tablet (Roche), 1 × PhosSTOP tablet (Roche)]. Whole cell extract was mixed with 4 μg indicated antibodies and rotated for 3 h at 4ºC, and then 20 μl of protein G beads were added and incubated for another hour. Beads were then washed three times with 1 ml IP buffer and boiled in 50 μl SDS-sample buffer. For DNA polymerase assays, after wash, beads were incubated with 1 nM DNA polymerase ϵ or 2 nM Pol2 described below. Western analysis was performed to detect specific proteins. Blots were probed with the indicated antibodies in phosphate-buffered saline containing 0.1% Tween and 2% dried milk. 9E10 (1:1000, Covance) was used to detect or precipitate Mrc1 carrying a myc13 tag.
Purification of Pol ϵ and Pol2 from S. cerevisiae
S. cerevisiae strains PY116 harboring pJL1, pJL5 or pJL9 for overexpression of yeast DNA Pol ϵ complex are generous gifts from Erik Johansson (Umeå University, Umeå, Sweden). Overexpression and purification of either 4-subunit Pol ϵ or the catalytic Pol2 subunit were carried out as described [Citation38–40].
DNA polymerase assays
DNA polymerase activity was measured by the incorporation of [3H]dTTP precursor into a poly(dA)-oligo(dT) substrate basically as described previously [Citation41]. Typical reaction mixtures contained 1 nM DNA polymerase ϵ or 2 nM Pol2 purified as abovementioned. Mrc1 protein was titrated from 0 to 100 nM. Mean ± SD are shown from three independent repeats. Statistical significance was measured using Student's t-test.
DNA binding assays by gel shift
The gel shift assays were performed basically as described in with minor modifications [Citation42]. 20-mer (5′-CGACCGTGCCAGCCTAAATTTCAATA-3′) and 59-mer (5′-AGGTCTCGACTAACTCTAGTCGTTGTTCCACCCGTCCACCCGACGCCACCTCCTG-3′) oligonucleotides were 5′-end labelled by T4 polynucleotide kinase in the presence of γ32P-ATP for preparing primer/template and dsDNA substrates, respectively. Then, DNA substrates were prepared through annealing them to an unlabeled complementary 59-mer oligonucleotide as the template. The substrates with the indicated structures were purified from the unannealed oligonucleotides by a 10% native polyacrylamide gel. The increasing amount of Mrc1 WT or mutant protein (0.15 μM, 0.3 μM, 0.45 μM, 0.6 μM) was incubated with 0.1 μM indicated 5′-labeled DNA substrates for 30 min at 4°C in a 10-μl volume containing 50 mM Tris-HCl, 50 mM NaCl, 10% glycerol, 1 mM EDTA, 2 mM dithiothreitol, 0.25 mg/ml BSA. The samples were analyzed through a 5% native polyacrylamide gel in 0.5 × TBE buffer followed by autoradiography/phosphorimaging (Typhoon Imager, GE Healthcare).
Primer extension assays
Primer extension assays were carried out using a 20-/59-mer DNA as a primer/template, which was 32P-labelled and prepared as described above. The activity of DNA polymerase ϵ was titrated in mixtures (20 μl) containing 2 nM 20-/59-mer primer/template, 200 μM each dNTP, 50 mM Tris-Cl (pH 7.5), 1 mM DTT, 10% glycerol, 8 mM MgCl2, 0.1 mg/mL BSA, DNA Pol ϵ (1 nM) and Mrc1 (0–100/nM).
The mixtures were incubated at 37°C for 0.5–10 min before being quenched with 2 μl of stop solution (250 mM EDTA, 98% formamide, 0.1% bromphenol blue, and 0.1% xylene cyanol). The samples were boiled for 4 min, chilled on ice, and then separated on an 8% polyacrylamide/bisacrylamide (19:1) gel containing 7 M urea in 0.5 × TBE buffer. The gel was analyzed using a Typhoon Imager (GE Healthcare). The fully extended primers were quantified by ImageQuant. The activity of DNA polymerase alone was normalized to 100%. Mean ± SD are shown from three independent experiments. Statistical significance was measured using Student's t-test.
Disclosure of potential conflicts of interest
No potential conflicts of interest were disclosed.
Acknowledgments
We are grateful to Drs. Erik Johansson (Umeå University), Francesc Posas (Universitat Pompeu Fabra) and Thomas Petes (Duke University) for sharing key reagents. This work was supported by the National Natural Science Foundation of China (Key Program 31630005, 31770084, 31771382 and 31271331); Opening Project of the State Key Laboratory of Microbial Resources; Program for Extramural Scientists (2015SKLAB6-26) of the State Key Laboratory of Agrobiotechnology; and Chinese Universities Scientific Fund 2015TC039.
Additional information
Funding
References
- Lambert S, Carr AM. Impediments to replication fork movement: stabilisation, reactivation and genome instability. Chromosoma. 2013;122:33–45. doi:10.1007/s00412-013-0398-9. PMID:23446515
- Magdalou I, Lopez BS, Pasero P, et al. The causes of replication stress and their consequences on genome stability and cell fate. Semin Cell DevBiol. 2014;30:154–164. doi:10.1016/j.semcdb.2014.04.035.
- Zeman MK, Cimprich KA. Causes and consequences of replication stress. Nat Cell Biol. 2014;16:2–9. doi:10.1038/ncb2897. PMID:24366029
- Muñoz S, Méndez J. DNA replication stress: from molecular mechanisms to human disease. Chromosoma. 2017;126:1–15. doi:10.1007/s00412-016-0573-x. PMID:26797216
- Friedel AM, Pike BL, Gasser SM. ATR/Mec1: coordinating fork stability and repair. Curr Opin Cell Biol. 2009;21:237–244. doi:10.1016/j.ceb.2009.01.017. PMID:19230642
- Branzei D, Foiani M. Maintaining genome stability at the replication fork. Nat Rev Mol Cell Biol. 2010;11:208–219. doi:10.1038/nrm2852. PMID:20177396
- Ciccia A, Elledge SJ. The DNA damage response: making it safe to play with knives. Mol Cell. 2010;40:179–204. doi:10.1016/j.molcel.2010.09.019. PMID:20965415
- Errico A, Costanzo V. Mechanisms of replication fork protection: a safeguard for genome stability. Crit Rev Biochem Mol Biol. 2012;47:222–235. doi:10.3109/10409238.2012.655374. PMID:22324461
- Cortez D. Preventing replication fork collapse to maintain genome integrity. DNA Repair. 2015;32:149–157. doi:10.1016/j.dnarep.2015.04.026. PMID:25957489
- Yazinski SA, Zou L. Functions, Regulation, and Therapeutic Implications of the ATR Checkpoint Pathway. Ann Rev Genet. 2016;50:155–173. doi:10.1146/annurev-genet-121415-121658. PMID:27617969
- Feng W. Mec1/ATR, the program manager of nucleic acids Inc. Genes. 2017;8:10.
- Byun TS, Pacek M, Yee MC, et al. Functional uncoupling of MCM helicase and DNA polymerase activities activates the ATR-dependent checkpoint. Genes Dev. 2005;19:1040–1052. doi:10.1101/gad.1301205. PMID:15833913
- Nedelcheva MN, Roguev A, Dolapchiev LB, et al. Uncoupling of unwinding from DNA synthesis implies regulation of MCM helicase by Tof1/Mrc1/Csm3 checkpoint complex. J Mol Biol. 2005;347:509–521. doi:10.1016/j.jmb.2005.01.041. PMID:15755447
- Zou L, Elledge SJ. Sensing DNA damage through ATRIP recognition of RPA-ssDNA complexes. Science. 2003;300:1542–1548. doi:10.1126/science.1083430. PMID:12791985
- Navadgi-Patil VM, Burgers PM. Cell-cycle-specific activators of the Mec1/ATR checkpoint kinase. Biochem Soc Trans. 2011;39:600–605. doi:10.1042/BST0390600. PMID:21428947
- Bermejo R, Lai Mong S, Foiani M. Preventing replication stress to maintain genome stability: resolving conflicts between replication and transcription. Molecular Cell. 2012;45:710–718. doi:10.1016/j.molcel.2012.03.001. PMID:22464441
- Iyer D, Rhind N. The Intra-S checkpoint responses to DNA damage. Genes. 2017;8:74. doi:10.3390/genes8020074.
- Tanaka K, Russell P. Mrc1 channels the DNA replication arrest signal to checkpoint kinase Cds1. Nat Cell Biol. 2001;3:966–972. doi:10.1038/ncb1101-966. PMID:11715017
- Alcasabas AA, Osborn AJ, Bachant J, et al. Mrc1 transduces signals of DNA replication stress to activate Rad53. Nat Cell Biol. 2001;3:958–965. doi:10.1038/ncb1101-958. PMID:11715016
- Kumagai A, Dunphy WG. Claspin, a novel protein required for the activation of Chk1 during a DNA replication checkpoint response in Xenopus egg extracts. Mol Cell. 2000;6:839–849. doi:10.1016/S1097-2765(05)00092-4. PMID:11090622
- Yang C-C, Suzuki M, Yamakawa S, et al. Claspin recruits Cdc7 kinase for initiation of DNA replication in human cells. Nat Commun. 2016;7:12135. doi:10.1038/ncomms12135. PMID:27401717
- Matsumoto S, Kanoh Y, Shimmoto M, et al. Checkpoint-independent regulation of origin firing by Mrc1 through interaction with Hsk1 kinase. Mol Cell Biol. 2017;37:e00355–e00316. doi:10.1128/MCB.00355-16.
- Szyjka SJ, Viggiani CJ, Aparicio OM. Mrc1 is required for normal progression of replication forks throughout Chromatin in S. cerevisiae. Mol Cell. 2005;19:691–697. doi:10.1016/j.molcel.2005.06.037.
- Tourriere H, Versini G, Cordon-Preciado V, Alabert C, Pasero P. Mrc1 and Tof1 promote replication fork progression and recovery independently of Rad53. Mol Cell 2005; 19. doi:10.1016/j.molcel.2005.07.028. PMID:16137625
- Hodgson B, Calzada A, Labib K. Mrc1 and Tof1 regulate DNA replication Forks in different ways during Normal S phase. Mol Biol Cell. 2007;18:3894–3902. doi:10.1091/mbc.E07-05-0500. PMID:17652453
- Petermann E, Helleday T, Caldecott KW. Claspin promotes normal replication fork rates in human cells. Mol Biol Cell. 2008;19:2373–2378. doi:10.1091/mbc.E07-10-1035. PMID:18353973
- Yeeles JTP, Janska A, Early A, et al. How the Eukaryotic replisome achieves rapid and efficient DNA replication. Mol Cell. 2017;65:105–116. doi:10.1016/j.molcel.2016.11.017. PMID:27989442
- Katou Y, Kanoh Y, Bando M, et al. S-phase checkpoint proteins Tof1 and Mrc1 form a stable replication-pausing complex. Nature 2003;424:1078–1083. doi:10.1038/nature01900. PMID:12944972
- Lou H, Komata M, Katou Y, et al. Mrc1 and DNA polymerase epsilon function together in linking DNA replication and the S phase checkpoint. Mol Cell. 2008;32:106–117. doi:10.1016/j.molcel.2008.08.020. PMID:18851837
- Duch A, Felipe-Abrio I, Barroso S, et al. Coordinated control of replication and transcription by a SAPK protects genomic integrity. Nature. 2013;493:116–119. doi:10.1038/nature11675. PMID:23178807
- Zhao H, Russell P. DNA binding domain in the replication checkpoint protein Mrc1 of Schizosaccharomyces pombe. J Biol Chem. 2004;279:53023–53027. doi:10.1074/jbc.M410449200. PMID:15471884
- Sar F, Lindsey-Boltz LA, Subramanian D, et al. Human Claspin is a ring-shaped DNA-binding protein with high affinity to branched DNA structures. J Biol Chem. 2004;279:39289–39295. doi:10.1074/jbc.M405793200. PMID:15226314
- Tanaka T, Yokoyama M, Matsumoto S, et al. Fission yeast Swi1-Swi3 complex facilitates DNA binding of Mrc1. J Biol Chem. 2010;285:39609–39622. doi:10.1074/jbc.M110.173344. PMID:20924116
- Uno S, Masai H. Efficient expression and purification of human replication fork-stabilizing factor, Claspin, from mammalian cells: DNA-binding activity and novel protein interactions. Genes Cells. 2011;16:842–856. doi:10.1111/j.1365-2443.2011.01535.x. PMID:21790909
- Cho W-H, Kang Y-H, An Y-Y, et al. Human Tim-Tipin complex affects the biochemical properties of the replicative DNA helicase and DNA polymerases. Proc Natl Acad Sci. 2013;110:2523–2527. doi:10.1073/pnas.1222494110.
- Aria V, De Felice M, Di Perna R, et al. The human Tim-Tipin complex interacts directly with DNA polymerase ε and stimulates its synthetic activity. J Biol Chem. 2013;288:12742–12752. doi:10.1074/jbc.M112.398073. PMID:23511638
- Quan Y, Xia Y, Liu L, et al. Cell-cycle-regulated interaction between Mcm10 and double Hexameric Mcm2-7 is required for helicase splitting and activation during S phase. Cell Rep. 2015;13:2576–2586. doi:10.1016/j.celrep.2015.11.018. PMID:26686640
- Hogg M, Osterman P, Bylund GO, et al. Structural basis for processive DNA synthesis by yeast DNA polymerase ϵ. Nat Struct Mol Biol. 2014;21:49–55. doi:10.1038/nsmb.2712. PMID:24292646
- Ganai RA, Osterman P, Johansson E. Yeast DNA polymerase ε catalytic core and holoenzyme have comparable catalytic rates. J Biol Chem. 2015;290:3825–3835. doi:10.1074/jbc.M114.615278. PMID:25538242
- Ganai RA, Zhang X-P, Heyer W-D, et al. Strand displacement synthesis by yeast DNA polymerase ϵ. Nucleic Acids Res. 2016;44:8229–8240. doi:10.1093/nar/gkw556. PMID:27325747
- Dua R, Edwards S, Levy DL, et al. Subunit interactions within the saccharomyces cerevisiae DNA Polymerase ϵ (pol ϵ) complex: DEMONSTRATION OF A DIMERIC pol ϵ. J Biol Chem. 2000;275:28816–28825. doi:10.1074/jbc.M002376200. PMID:10878005
- Niu Y, Xia Y, Wang S, et al. A PRototypic lysine methyltransferase 4 from archaea with degenerate sequence specificity methylates chromatin proteins Sul7d and Cren7 in different patterns. J Biol Chem. 2013;288:13728–13740. doi:10.1074/jbc.M113.452979. PMID:23530048