ABSTRACT
Fetal growth restriction (FGR) affects up to 5% of pregnancies and is associated with significant perinatal complications. Maternal deficiency of vitamin D, a secosteroid hormone, is common in FGR-affected pregnancies. We recently demonstrated that decreased expression of the vitamin D receptor (VDR) in idiopathic FGR placentae could impair trophoblast growth. As strict regulation of cell-cycle genes in trophoblast cells is critical for optimal feto-placental growth, we hypothesised that pathologically decreased placental VDR contributes to aberrant regulation of cell-cycle genes. The study aims were to (i) identify the downstream cell-cycle regulatory genes of VDR in trophoblast cells, and (ii) determine if expression was changed in cases of FGR. Targeted cell-cycle gene cDNA arrays were used to screen for downstream targets of VDR in VDR siRNA-transfected BeWo and HTR-8/SVneo trophoblast-derived cell lines, and in third trimester placentae from FGR and gestation-matched control pregnancies (n = 25 each). The six candidate genes identified were CDKN2A, CDKN2D, HDAC4, HDAC6, TGFB2 and TGFB3. TGFB3 was prioritised for further validation, as its expression is largely unknown in FGR. Significantly reduced mRNA and protein expression of TGFB3 was verified in FGR placentae and the BeWo and HTR-8/SVneo trophoblast cell lines, using real-time PCR and immunoblotting respectively. In summary, decreased placental VDR expression alters the expression of regulatory cell-cycle genes in FGR placentae. Aberrant regulation of cell-cycle genes in the placental trophoblast cells may constitute a mechanistic pathway by which decreased placental VDR reduces feto-placental growth.
Introduction
Vitamin D is a pleiotropic secosteroid (i.e., ‘open ring’) hormone with effects in many tissues throughout the body. Vitamin D exerts its physiological effects principally through binding with its receptor VDR and regulating the expression of genes responsible for cellular proliferation, differentiation, apoptosis and angiogenesis in local tissues [Citation1,Citation2]. Not surprisingly, vitamin D has been shown to exhibit anti-proliferative as well as pro-differentiation activity in a variety of cell types including keratinocytes, osteoblasts, mesenchymal, neural, vascular endothelial, chondrocytes and immune cells [Citation3].
In pregnancy, vitamin D has important roles in maintaining the health of both mother and child. Deficiency of vitamin D has been linked with increased risks of multiple adverse pregnancy outcomes including pre-eclampsia, gestational diabetes, preterm birth and small for gestational age babies [Citation4]. Furthermore, vitamin D deficient women are at risk of not only osteopenia, osteoporosis, and increased risk of infertility, but are also more likely to carry a growth restricted infant [Citation5]. Interestingly, the incidences of low birth weight offspring is decreased in women who receive the recommended doses of calcium and vitamin D during pregnancy [Citation6,Citation7]. Given the importance of the intrauterine environment mediating both immediate and long-term effects on the health of the offspring, which may not be fully reversible [Citation8], understanding how vitamin D deficiency may cause FGR needs to be established.
At the maternal-fetal interface, vitamin D aids pregnancy implantation and maintenance by increasing immune tolerance [Citation9]. Vitamin D diffusion from the mother to the fetus is thought to be facilitated by serum concentrations of 1,25(OH)2D, the active form of vitamin D, being higher in the maternal circulation compared to the fetal circulations [Citation10], but it is now clear that the decidua and placental trophoblast cells generate large amounts of 1,25(OH)2D [Citation11–Citation13]. In early pregnancy, 1,25(OH)2D induces decidualisation, a critical process for successful implantation [Citation14,Citation15]. During pregnancy, the conversion of 25(OH)D to 1,25(OH)2D is increased in the placenta as well as the maternal kidney and decidua [Citation13]. Placental trophoblast cells respond to 1,25(OH)2D, and influences the anti-inflammatory and anti-microbial responses [Citation15].
Vitamin D signalling pathways are proposed to be important in placental development. The presence of vitamin D receptor VDR, and vitamin D hydroxylases CYP27B1 and CYP24A1 in trophoblast cells, in addition to their ability to synthesise vitamin D, suggests that vitamin D/VDR pathway might have an important role in placental development and function. Our recent work demonstrated reduced VDR expression in placentae from idiopathic fetal growth restricted pregnancies, which was associated with abnormal trophoblast cell differentiation and apoptosis in vitro [Citation16]. Trophoblast cell proliferation is mediated by the induction of cell cycle inhibitors that prevent the transition from DNA synthesis to the differentiation phase by changes in the expression of growth factors and cytokines [Citation3]. Like malignant cells, trophoblast cells differentiate into migratory extravillous trophoblast cells [Citation17], a well-controlled process during normal placentation [Citation18,Citation19], which is fundamental for subsequent invasion of the endometrium and development of the placenta. Cell proliferation is largely regulated by the interaction of cyclins, cyclin-dependent kinases (CDKs), and tumour suppressor gene products namely TP53 [Citation20,Citation21]. As such, the interaction of vitamin D through VDR with these genes in trophoblast cells may affect placental development in early pregnancy, and therefore influence the trajectory of subsequent fetal growth.
Hence, in this study, we hypothesised that regulatory cell-cycle genes are downstream target genes of VDR and that their placental expression will be altered in idiopathic FGR-affected pregnancies compared with gestation-matched uncomplicated pregnancies. We used two siRNA-transfected placental trophoblast cell lines to identify downstream cell-cycle gene targets of VDR using a cell-cycle gene PCR array, and then verified the findings in a study of placentae obtained from normal and FGR-affected pregnancies. We also determined the functional effect of silencing VDR expression on cell cycle progression by examining trophoblast proliferation in vitro.
Results
VDR siRNA inactivation in BeWo and HTR-8/SVneo trophoblast cell lines
VDR was previously determined to be significantly reduced by 75% and 70% at the mRNA and protein levels respectively in BeWo cells after 72 hours of siRNA transfection using si2, while no effect was observed for si1 [Citation16]. For the HTR-8/SVneo cells, there were significant decreases of VDR at both the mRNA and protein levels 48 hours after transfection with si1 and si2 (). VDR mRNA was significantly reduced by 87% and 93% by si1 and si2 respectively (). The decreases in VDR mRNA were then further confirmed at the protein level using immunoblotting (). Significant decreases of 36% and 31% of VDR protein were achieved (). As si2 showed the most consistent knockdown at both the mRNA and protein levels for both cell lines, all subsequent analyses were performed with si2.
Figure 1. VDR siRNA knockdown in HTR-8/SVneo trophoblast cell line. A: Reduction of VDR mRNA expression after siRNA transfection at 48 hours. B: Representative immunoblot of VDR protein and GAPDH as loading control at 47kDa and 37kDa respectively. C: Protein quantitation of VDR expression at 48 hours. The graphs depict results from n≥3 independent experiments. Data presented as mean ± SEM. **p<0.01, ***p<0.001, One way ANOVA with Bonferroni's post-test.
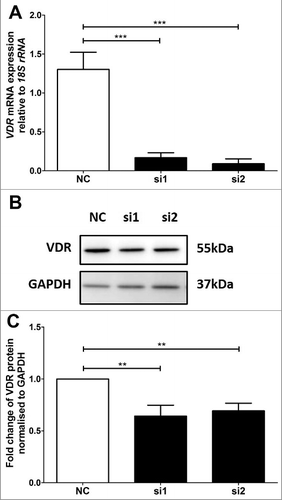
Following VDR si2 treatment, functional analysis in HTR-8/SVneo demonstrated a time-dependent increase in proliferation at 2–8h, thereafter a significant decrease in proliferation was observed at 24h and 48h (). However, in BeWo cells, a significant in proliferation was observed only at 24h () and no significant change was observed at all other time points tested (data not shown).
Figure 2. Functional analysis following VDR siRNA transfection. A time-dependent significant alteration in proliferation of HTR-8/SVneo (A) and in BeWo cells (B) as determined by absorbance from CyQuant assay. Significant increase of HTR-8/SVneo cell proliferation was observed at 4 and 8h time points and thereafter from 24h time-point a significant decrease in proliferation was observed. In BeWo cells a significant increase in proliferation was observed at 24h. Data of n = 3 independent experiments performed at least in triplicate or quadruplicate are presented as mean ± SEM. ***p ≤ 0.001, ****p ≤ 0.0001. one-way ANOVA.
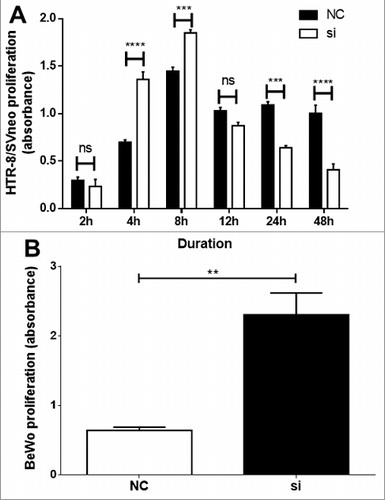
Cell-cycle gene screening PCR array
Consistent changes were observed in 6 cell-cycle genes across pooled samples from VDR siRNA-transfected trophoblast cell lines and human idiopathic FGR-affected placentae, and their respective controls (). The six candidate genes were CDKN2A, CDKN2D, HDAC4, HDAC6, TGFB2 and TGFB3 (). Of these downstream target genes identified, TGFB3 was then selected for further validation, as its expression is largely unknown in FGR-affected placentae.
Table 1. Panel of cell-cycle genes examined in cDNA array and their associated fold-changes in expression. Genes in bold were those that showed consistent changes in pooled samples from human FGR placentae and the VDR siRNA transfected BeWo and HTR-8/SVneo trophoblast cell lines relative to their respective controls. 18S rRNA served as the housekeeping gene for all samples.
Figure 3. Relative expression of candidate downstream target cell-cycle genes of VDR. Identified cell-cycle genes demonstrated consistent gene expression changes in pooled samples of VDR siRNA transfected BeWo and HTR-8/SVneo trophoblast cells (n = 3 independent experiments performed at least in triplicate wells for treatment groups) and FGR placentae (n = 25) compared with gestation-matched control placentae (n = 25). CDKN2A: cyclin-dependent kinase inhibitor 2A; CDKN2D: cyclin-dependent kinase inhibitor 2D; HDAC4: histone deacetylase 4; HDAC6: histone deacetylase 6; TGFB2: transforming growth factor beta 2; TGFB3: transforming growth factor beta 3. Data presented from pooled samples, therefore no error bars provided.
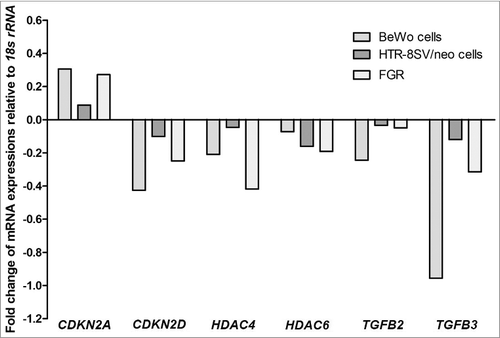
TGFB3 validation in trophoblast cell lines
Altered expression of TGFB3 mRNA and TGFβ3 protein was verified using real-time PCR and immunoblotting in VDR siRNA transfected BeWo () and HTR-8/SVneo () cells. In the VDR siRNA transfected BeWo cells, TGFB3 mRNA and TGFβ3 protein were reduced by approximately 92% and 66% respectively (), as compared with BeWo cells transfected with the non-targeted siRNA control (NC). Similarly, HTR-8/SVneo cells transfected with VDR siRNA had significant reductions of approximately 95% and 30% for TGFB3 mRNA and TGFβ3 protein respectively relative to NC ().
Figure 4. Validation of reduced TGFB3 mRNA and TGFβ3 protein expression in BeWo trophoblast cells. A: Reduction of TGFB3 mRNA expression following 48h VDR siRNA transfection, where ‘NC’ refers to non-targeted siRNA control and ‘si’ refers to VDR siRNA treated cells. B: Representative immunoblot of TGFβ3 protein and GAPDH as loading control at 47kDa and 37kDa respectively. C: Protein quantitation of TGFβ3 expression. The graphs depict results from n≥3 independent experiments. Data presented as mean ± SEM. *p < 0.05, ***p<0.001, Student's t test.
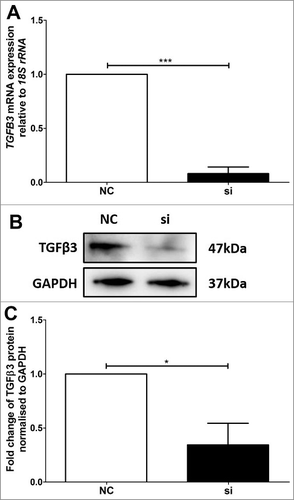
Figure 5. Validation of reduced TGFB3 mRNA and TGFβ3 protein expression in HTR-8/SVneo trophoblast cells. A: Reduction of TGFB3 mRNA expression after siRNA transfection, where ‘NC’ refers to non-targeted siRNA control and ‘si’ refers to VDR siRNA2 treated cells. B: Representative immunoblot of TGFβ3 protein and GAPDH as loading control at 47kDa and 37kDa respectively. C: Protein quantitation of TGFβ3 expression. The graphs depict results from n≥3 independent experiments. Data presented as mean ± SEM. *p < 0.05, ***p<0.001, Student's t test.
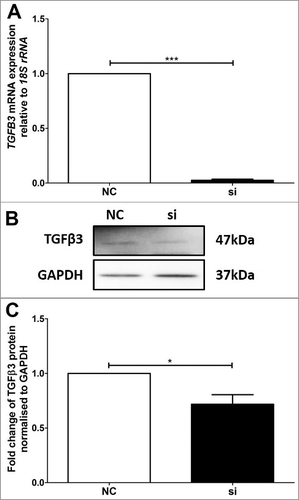
TGFB3 validation in human placentae
TGFB3 mRNA expression was significantly decreased by 46% in placental tissue from third-trimester idiopathic FGR-affected pregnancies compared with that from control pregnancies (). Representative immunoreactive TGFβ3 (47kDa, top panel) and protein loading control, GAPDH (37kDa, bottom panel), are shown in . Semi-quantitation of TGFβ3 immunoreactive protein relative to GAPDH showed a significant reduction of 64% in FGR placentas compared with placentas from the gestational age-matched control placentas ().
Figure 6. Validation of reduced TGFB3 mRNA and TGFβ3 protein expression in third trimester control and FGR placentae. A: TGFB3 mRNA expression was reduced in FGR placentae (n = 25) compared with control placentae (n = 25). B: Representative immunoblot of TGFβ3 protein and GAPDH as loading control at 47kDa and 37kDa respectively. C: Protein quantitation of TGFβ3 expression was performed in a subset of n = 8 control and n = 7 FGR placentae. Data presented as mean ± SEM.*p < 0.05, ***p<0.001, Student's t test.
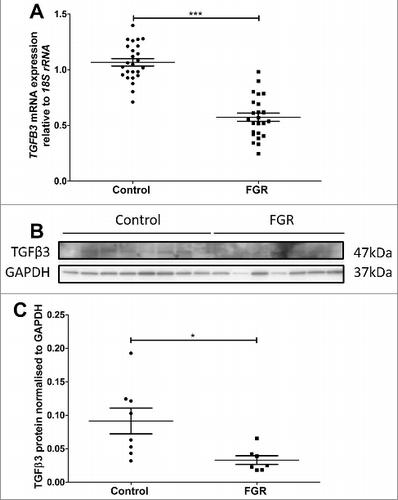
Discussion
Feto-placental growth is a complex process requiring tight regulation of cell cycle gene expressions at the transcriptional level. Proliferation, continuous syncytial fusion and cascade of apoptosis are necessary for integrity maintenance of the syncytium layer [Citation22], and remodelling of spiral arterioles by migratory and invasive extravillous trophoblasts [Citation23]. Thus, placental growth is heavily dependent on villous cytotrophoblast proliferation as they give rise to two major trophoblast subpopulations – syncytiotrophoblast, of a fusion phenotype and extravillous trophoblasts, of an invasive phenotype. Inhibition of the cell cycle is almost always a requisite for differentiation. Forced inhibition of the cell cycle very often induces terminal differentiation and vice versa [Citation24,Citation25]. The molecular pathways that couple the cell cycle to differentiation involve molecules of the G1/S transition including growth factors, downstream signalling pathways, Myc, the Rb/E2F pathway, and the CDK inhibitors (e.g., p21). Inhibition of cell proliferation in the G1 phase is often accompanied by cell differentiation. Therefore, in this study we utilised a cell-cycle specific array, which included the key genes that are critical for cell-cycle regulation.
By using the siRNA-mediated transfection VDR approach, we investigated the mechanisms by which VDR mediates the genes that are crucial for the functional regulation of both syncytiotrophoblast and extravillous trophoblast cells in normal and FGR-affected placentae. We used the BeWo and HTR-8/SVneo cell lines as in vitro models of syncytiotrophoblast and extravillous trophoblast cells, and identified downstream target genes of VDR, which included 20 up-regulated genes and 6 down-regulated genes. These findings were further verified in human idiopathic FGR-affected placentae. This study showed for the first time that decreased VDR expression results in significant changes in downstream cell cycle regulatory genes including up-regulated CDKN2A and down regulated CDKN2D, HDAC4, HDAC6, TGFB2 and TGFB3. Expression of cell cycle regulators change as a function of differentiation and gestational age [Citation26]. However, it is not well understood how trophoblast proliferation is coordinated with differentiation. Therefore, we also demonstrated significant alterations in the proliferation of BeWo and HTR-8/SVneo cells following VDR knockdown, highlighting the importance of VDR in regulating cell cycle progression in trophoblast cells.
Trophoblast proliferation is regulated spatially and temporally throughout pregnancy [Citation27]. Proliferation or cell division goes through cell growth and DNA synthesis phases, which are mediated by checkpoints to ensure fidelity of the replication. Previous studies of targeted disruption of specific transcription factors in transgenic animals have shown that characteristics of FGR, including compromised trophoblast function and placental insufficiency, can arise when trophoblast proliferation and cell growth are affected [Citation28,Citation29]. A commercially available human cell cycle PCR array was preferred over the whole genome microarray to identify the downstream target genes of VDR involved in cell cycle progression, as this was more specific and directly important to the pathways regulating trophoblast development. We previously used this method and successfully identified novel downstream targets of placental transcription factors [Citation30]. The downstream genes of VDR identified were differentially expressed, suggesting their involvement in trophoblast proliferation and differentiation, and potential importance in establishing normal placental trophoblast function (). The possibility that altered expressions of these downstream targets are implicated in placental pathologies of idiopathic FGR-affected pregnancies was confirmed by the finding of similar gene expression patterns in the FGR and GMC placental samples. Clinically, several of these cell cycle regulators have the potential to be developed as markers of trophoblast dysfunction.
Figure 7. The functions and the mechanism of vitamin D receptor. 25-hydroxyvitamin D [25(OH)D] is the major circulating form of vitamin D that is used by clinicians to determine vitamin D status. This form of vitamin D is biologically inactive and is bound to the vitamin D–binding protein (VDBP), which transports it in the circulation to target organs, tissues and cells. 25(OH)D is converted by CYP27B1 to the biologically active form, 1,25-dihydroxyvitamin D [1,25(OH)2D] in targeted cells. Through intrinsic synthesis of 1,25(OH)2D3 and uptake of 1,25(OH)2D3 from the extracellular environment, vitamin D receptor (VDR) is activated and heterodimerizes with retinoid X receptor (RXR). This VDR/RXR complex is translocated into the nucleus and binds to the vitamin D response element (VDRE) in the promoter region to either induce or suppress transcription of a variety of genes. To control VDR activity a series of negative feedback loops exists; activated VDR both induce expression of the 1,25(OH)2D degrading enzyme CYP24A1 and down-regulates expression of the 1,25(OH)2D synthesizing enzyme CYP27B1. Decreased VDR expression results in significant changes in downstream cell cycle regulatory genes including up-regulated CDKN2A and down regulated CDKN2D, HDAC4, HDAC6, TGFB2 and TGFB3. The downstream genes of VDR were differentially expressed, suggesting their involvement in trophoblast function (proliferation, differentiation, migration and invasion), and contributing to placental insufficiency in fetal growth restriction (FGR).
![Figure 7. The functions and the mechanism of vitamin D receptor. 25-hydroxyvitamin D [25(OH)D] is the major circulating form of vitamin D that is used by clinicians to determine vitamin D status. This form of vitamin D is biologically inactive and is bound to the vitamin D–binding protein (VDBP), which transports it in the circulation to target organs, tissues and cells. 25(OH)D is converted by CYP27B1 to the biologically active form, 1,25-dihydroxyvitamin D [1,25(OH)2D] in targeted cells. Through intrinsic synthesis of 1,25(OH)2D3 and uptake of 1,25(OH)2D3 from the extracellular environment, vitamin D receptor (VDR) is activated and heterodimerizes with retinoid X receptor (RXR). This VDR/RXR complex is translocated into the nucleus and binds to the vitamin D response element (VDRE) in the promoter region to either induce or suppress transcription of a variety of genes. To control VDR activity a series of negative feedback loops exists; activated VDR both induce expression of the 1,25(OH)2D degrading enzyme CYP24A1 and down-regulates expression of the 1,25(OH)2D synthesizing enzyme CYP27B1. Decreased VDR expression results in significant changes in downstream cell cycle regulatory genes including up-regulated CDKN2A and down regulated CDKN2D, HDAC4, HDAC6, TGFB2 and TGFB3. The downstream genes of VDR were differentially expressed, suggesting their involvement in trophoblast function (proliferation, differentiation, migration and invasion), and contributing to placental insufficiency in fetal growth restriction (FGR).](/cms/asset/2ffb9402-c215-43c6-8ba4-56b00e55cdc0/kccy_a_1405193_f0007_oc.jpg)
This study showed that a novel candidate VDR downstream target gene, TGFB3, an inhibitor of cell cycle progression through G1 phase, and its protein TGFβ3 are differentially expressed in human idiopathic FGR-affected placentas. Transcriptional regulation of feto-placental development is well-documented in mouse knockout studies where targeted disruption of specific transcription factors has demonstrated FGR-like effects including impaired vascular and trophoblast development and placental insufficiency [Citation31]. In vivo, transforming growth factor betas (TGFβs), members of a large super family of cytokines [Citation32], are primarily produced by the decidua, and proposed to regulate trophoblast invasion [Citation33]. TGFβs induce morphological changes characteristic of epithelial-to-mesenchymal transition including reorganization of the cytoskeleton, down-regulation of E-cadherin, ZO-1, vinculin and keratin, and the over-expression of vimentin [Citation34]. Normal human pregnancy depends on adequate spiral arteriole remodelling induced by invasive trophoblast. Inhibition of placental TGFβ3, which is over-expressed in pre-eclampsia that is associated with impaired trophoblast invasion, restores the invasive capacity of these extravillous trophoblast cells [Citation35]. Nevertheless, another study of showed no changes in placental TGFβ3 expression in preeclampsia and FGR compared with normal pregnancies [Citation36]. Thus, although TGFβ3 can regulate trophoblast invasion in vitro, in vivo data are inconsistent between groups and may be related to sample size and different underlying causes of pathology.
In summary, our study demonstrates that decreased placental VDR expression contributes to altered expression of TGFβ3 in FGR, suggesting a mechanistic pathway by which decreased placental VDR expression may contribute to abberant cell-cycle regulation thereby reducing the feto-placental growth in human idiopathic FGR. Decreased TGFβ3 expression may contribute to uncontrolled cell proliferation and premature differentiation, leading to inadequate formation and regeneration of the syncytium, which may impair exchange of nutrients and synthesis of hormones required for feto-placental growth and development, thus causing placental insufficiency, the common underlying characteristic of human idiopathic FGR.
Materials and methods
Patient samples
Third trimester placental tissue samples were collected with written informed consent from 25 idiopathic FGR-affected and 25 gestation-matched control (GMC) pregnancies at The Royal Women's Hospital (Melbourne, Victoria, Australia). Ethics approval was obtained from the hospital's Human Research Ethics Committee. Using these placental samples, previous studies in our laboratory have shown consistent gene expression changes in FGR compared with gestation-matched control pregnancies [Citation16,Citation37–Citation41]. FGR was defined as birthweight less than the 10th centile for gestational age according to Australian growth charts [Citation42], accompanied by two or more of the following features: abnormal umbilical artery Doppler flow velocimetry; oligohydramnios as determined by amniotic fluid index (AFI) of <7; asymmetric growth of the fetus as defined by a head circumference (HC) to abdominal circumference (AC) ratio >1.2. The exclusion criteria for both FGR and GMC pregnancies were multiple pregnancies, illicit drug dependency, maternal smoking, pre-eclampsia, prolonged rupture of the membranes, placental abruption, intrauterine viral infection, and fetal congenital anomalies. Briefly, placentae were collected and processed within 20 minutes of birth. Placental tissues were excised from randomly selected areas of central placental cotyledons, avoiding areas with infarcts and decidua. Samples of fresh placental tissues were snap frozen in liquid nitrogen, then stored at -80°C for subsequent RNA and protein analyses.
Trophoblast cell lines
The choriocarcinoma-derived BeWo trophoblast cell line can be induced to fuse and form a multi-nucleated monolayer in vitro, and is an established model for the syncytium [Citation43]. The BeWo cell line was a kind gift from Prof. Stephen Rogerson (Department of Medicine, The Royal Melbourne Hospital, Australia). The HTR-8/SVneo cell line is a well-characterised transformed cell line used to model extravillous trophoblast cells [Citation44]. The cell line was kindly provided by Prof. Charles Graham (Queen's University, Kingston, ON, Canada). Both cells lines were maintained in RPMI-1640 tissue culture medium (Thermo Fisher Scientific), supplemented with 2 mM L-glutamine, 100 U/mL penicillin, 100 μg/mL streptomycin (Thermo Fisher Scientific) and 10%(v/v) fetal bovine serum (Thermo Fisher Scientific) in a humidified chamber at 37°C (5% CO2/95% air).
VDR inactivation using short interference RNA
To silence VDR expression, cultured trophoblast cells were transfected with two independent Ambion® VDR siRNAs – si1 and si2 (Thermo Fisher Scientific), using RNAiFect transfection reagent (Qiagen), as described previously [Citation16]. BeWo cells were transfected with 15nM of siRNA for 72 hours, while HTR-8/SVneo cells were transfected with 40nM of siRNA for 48 hours. Non-targeting siRNA (Santa Cruz Biotechnology Inc.) served as a negative control (NC) for transfection experiments.
RNA extraction and cDNA synthesis
Total placental RNA was extracted using the RNeasy Midi-kits (Qiagen). Total RNA from cultured cells was extracted using the Purelink™ RNA Mini Kit (Life Technologies Corp.), both according to the manufacturers’ protocols. The Nanodrop 2000 Spectrophotometer (Thermo Fisher Scientific) was used to determine the yield and purity of the RNA. Total placental and cellular RNA were reverse-transcribed and cDNA was prepared in a two-step reaction using 2 μg of RNA as described previously [Citation16].
Quantitative real-time reverse transcription polymerase chain reaction
Quantification of mRNA expression relative to the house-keeping gene, 18S rRNA, was determined by quantitative real-time reverse transcription polymerase chain reaction (qRT-PCR) on the 7500 Real-time PCR System (Applied Biosystems) as described previously [Citation16]. The probes used were FAM (VDR Hs00172113_m1; TGFB3 Hs01086000_m1) and VIC (18S rRNA, 4319413E-1112055). PCR data were analysed according to the 2−ΔΔCT method [Citation45].
Human regulatory cell cycle gene cDNA array
The TaqMan® Array Human Cyclins & Cell Cycle Regulation, Fast 96-Well Plate (Cat#: 4418768; Applied Biosystems) was used to screen and identify the downstream target genes of VDR from pooled human placentae and trophoblast cell cDNA samples according to the manufacturer's instructions. This array was chosen as it included 44 key genes that are critical for the regulation of proliferation of cells in the G1 phase. The PCR array reaction was performed on the ABI Prism 7500 Sequence Detector (Applied Biosystems) under these cycling parameters: 95°C for 10 minutes, followed by 40 cycles of denaturation at 95°C for 15 minutes and primer extension at 60°C for 1 minute. Data were analysed using the DataAssist™ Software v3.01 (Life Technologies) and presented as relative quantification (RQ; log fold changes). Relative expression levels of genes were normalised against that of the endogenous reference gene 18S rRNA to compensate for possible differences in the loading amount of cDNA, threshold was set at <0.1, and tested groups (targeted vs non-targeted siRNA cells; FGR vs control placentae) were compared.
Protein extraction and western immunoblotting
Protein was extracted from placentae of FGR-affected and GMC pregnancies and cultured trophoblast cells as described previously [Citation16]. Briefly, for western immunoblotting, total protein (25 μg) from each sample was electrophoresed on a precast gradient gel (4-12% Tris-HCl; Bio-Rad Laboratories) under reducing conditions with XT sample buffer (Bio-Rad Laboratories) and transferred onto a polyvinylidene fluoride (PVDF) membrane (Merck Millipore). An affinity purified mouse VDR monoclonal antibody (0.2 μg/mL; R&D Systems), rabbit VDR polyclonal antibody (0.4 μg/mL; Santa Cruz Biotechnology Inc.), and mouse monoclonal TGFβ3 antibody (0.4 μg/ml, Santa Cruz Biotechnology Inc.) were used to probe the membranes; rabbit monoclonal glyceraldehyde 3-phosphate dehydrogenase (GAPDH; Imgenex) or tubulin monoclonal antibody (Imgenex) were used for normalising the total protein load. Then, secondary antibody was applied and incubated at RT for 1 hour. Secondary antibodies used include rabbit anti-mouse IgG (H+L) horse-radish peroxidise (HRP) conjugate (750 ng/ml; Invitrogen) and goat anti-rabbit IgG (H+L) HRP conjugate (500 ng/ml; Life Technologies). A tyramide signal amplification kit (Perkin-Elmer) was used to amplify the immunoreactivity for VDR protein following the manufacturer's instructions where necessary. Enhanced chemiluminescence (ECL) prime Western blotting detection reagent (GE Healthcare) or Clarity™ Western ECL substrate (Bio-Rad Laboratories) was then applied to visualise the protein of interest by exposing the membrane using ImageQuant LAS-4000 (GE Healthcare). The level of immunoreactive protein of interest relative to the total protein loading control antibodies (GAPDH or tubulin) was determined semi-quantitatively using NIH ImageJ 1.44p scanning densitometry software.
Proliferation assay
Cell proliferation was measured by using CyQuant NF proliferation assay. HTR-8/SVneo and BeWo cells were seeded at a density of 10,000 cells per well and treated with VDR si2 for 2, 4, 8, 12, 24 and 48h in culture. Dye solution was prepared following manufacturer's instructions, and then 50 µl of dye solution was dispensed into each well and incubated for at least 1 hour. Then, the plate was read and measured for fluorescence absorbance, densitometry was analysed by ImageQuantile TL software.
Statistical analyses
Statistical analyses were performed using GraphPad Prism Software Version 5.0. Student's t tests and One-Way Analysis of Variance (ANOVA) were used as appropriate. Categorical data were analysed using the 2 × 2 contingency table with Fisher's exact test. A value of p< 0.05 was considered as significant.
Disclosure of potential conflicts of interest
The authors declare no conflict of interest.
Acknowledgements
This work was supported by an award to PM and PRE from the Australian Institute of Musculoskeletal Science, Western Health, Victoria, Australia.
Additional information
Funding
References
- Banerjee P, Chatterjee M. Antiproliferative role of vitamin D and its analogs–a brief overview. Mol Cell Biochem. 2003;253:247–254. doi:10.1023/A:1026072118217. PMID:14619976
- Verstuyf A, Carmeliet G, Bouillon R, et al. Vitamin D: a pleiotropic hormone. Kidney Int. 2010;78:140–145. doi:10.1038/ki.2010.17. PMID:20182414
- Samuel S, Sitrin MD. Vitamin D's role in cell proliferation and differentiation. Nutr Rev. 2008;66:S116–S124. doi:10.1111/j.1753-4887.2008.00094.x. PMID:18844838
- Wei SQ, Qi HP, Luo ZC, et al. Maternal vitamin D status and adverse pregnancy outcomes: a systematic review and meta-analysis. J Matern Fetal Neonatal Med. 2013;26:889–899. doi:10.3109/14767058.2013.765849. PMID:23311886
- Russell JG, Hill LF. True fetal rickets. Brit J Radiol. 1974;47:732–734. doi:10.1259/0007-1285-47-562-732. PMID:4433986
- Sabour H, Hossein-Nezhad A, Maghbooli Z, et al. Relationship between pregnancy outcomes and maternal vitamin D and calcium intake: a cross-sectional study. Gynecological Endocrinology: The Official Journal of the International Society of Gynecological Endocrinology. 2006;22:585–589. doi:10.1080/09513590601005409. PMID:17135038
- Scholl TO, Chen X. Vitamin D intake during pregnancy: association with maternal characteristics and infant birth weight. Early Hum Dev. 2009;85:231–234. doi:10.1016/j.earlhumdev.2008.10.006. PMID:19008055
- Barker DJ. The fetal and infant origins of adult disease. BMJ. 1990;301:1111. doi:10.1136/bmj.301.6761.1111. PMID:2252919
- Evans KN, Bulmer JN, Kilby MD, et al. Vitamin D and placental-decidual function. J Soc Gynecol Invest. 2004;11:263–271. doi:10.1016/j.jsgi.2004.02.002. PMID:15219879
- Kovacs CS, Kronenberg HM. Maternal-fetal calcium and bone metabolism during pregnancy, puerperium, and lactation. Endocr Rev. 1997;18:832–872. PMID:9408745
- Diaz L, Sanchez I, Avila E, et al. Identification of a 25-hydroxyvitamin D3 1alpha-hydroxylase gene transcription product in cultures of human syncytiotrophoblast cells. J Clin Endocrinol Metab. 2000;85:2543–2549. PMID:10902806
- Evans KN, Nguyen L, Chan J, et al. Effects of 25-hydroxyvitamin D3 and 1,25-dihydroxyvitamin D3 on cytokine production by human decidual cells. Biol Reprod. 2006;75:816–822. doi:10.1095/biolreprod.106.054056. PMID:16957024
- Weisman Y, Harell A, Edelstein S, et al. 1 alpha, 25-Dihydroxyvitamin D3 and 24,25-dihydroxyvitamin D3 in vitro synthesis by human decidua and placenta. Nature. 1979;281:317–319. doi:10.1038/281317a0. PMID:551281
- Du H, Daftary GS, Lalwani SI, et al. Direct regulation of HOXA10 by 1,25-(OH)2D3 in human myelomonocytic cells and human endometrial stromal cells. Mol Endocrinol. 2005;19:2222–2233. doi:10.1210/me.2004-0336. PMID:15905361
- Shin JS, Choi MY, Longtine MS, et al. Vitamin D effects on pregnancy and the placenta. Placenta. 2010;31:1027–1034. doi:10.1016/j.placenta.2010.08.015. PMID:20863562
- Nguyen TP, Yong HE, Chollangi T, et al. Placental vitamin D receptor expression is decreased in human idiopathic fetal growth restriction. J Mol Med. 2015;93:795–805. doi:10.1007/s00109-015-1267-1. PMID:25716068
- Yagel S, Parhar RS, Jeffrey JJ, et al. Normal nonmetastatic human trophoblast cells share in vitro invasive properties of malignant cells. J Cell Physiol. 1988;136:455–462. doi:10.1002/jcp.1041360309. PMID:3170642
- Fisher SJ, Damsky CH. Human cytotrophoblast invasion. Semin Cell Biol. 1993;4:183–188. doi:10.1006/scel.1993.1022. PMID:8347835
- Strickland S, Richards WG. Invasion of the trophoblasts. Cell. 1992;71:355–357. doi:10.1016/0092-8674(92)90503-5. PMID:1423599
- Finlay CA, Hinds PW, Levine AJ. The p53 proto-oncogene can act as a suppressor of transformation. Cell. 1989;57:1083–1093. doi:10.1016/0092-8674(89)90045-7. PMID:2525423
- Karp JE, Broder S. Molecular foundations of cancer: new targets for intervention. Nat Med. 1995;1:309–320. doi:10.1038/nm0495-309. PMID:7585060
- Huppertz B, Frank HG, Kingdom JC, et al. Villous cytotrophoblast regulation of the syncytial apoptotic cascade in the human placenta. Histochem Cell Biol. 1998;110:495–508. doi:10.1007/s004180050311. PMID:9826129
- Lyall F, Bulmer JN, Kelly H, et al. Human trophoblast invasion and spiral artery transformation: the role of nitric oxide. Am J Pathol. 1999;154:1105–1114. doi:10.1016/S0002-9440(10)65363-1. PMID:10233849
- Muñoz-Alonso M, Leon J. G1 phase control and cell differentiation. In: Boonstra J, editor. G1 phase progression. Georgetown (TX): Landes Bioscience; 2003. p. 236–264.
- Budirahardja Y, Gonczy P. Coupling the cell cycle to development. Development. 2009;136:2861–2872. doi:10.1242/dev.021931. PMID:19666818
- Genbacev O, McMaster MT, Fisher SJ. A repertoire of cell cycle regulators whose expression is coordinated with human cytotrophoblast differentiation. Am J Pathol. 2000;157:1337–1351. doi:10.1016/S0002-9440(10)64648-2. PMID:11021837
- Morrish DW, Dakour J, Li H. Functional regulation of human trophoblast differentiation. J Reprod Immunol. 1998;39:179–195. doi:10.1016/S0165-0378(98)00021-7. PMID:9786461
- Rossant J, Cross JC. Placental development: lessons from mouse mutants. Nat Rev Genet. 2001;2:538–548. doi:10.1038/35080570. PMID:11433360
- Sapin V, Blanchon L, Serre AF, et al. Use of transgenic mice model for understanding the placentation: towards clinical applications in human obstetrical pathologies? Transgenic Res. 2001;10:377–398. doi:10.1023/A:1012085713898. PMID:11708649
- Chui A, Kalionis B, Abumaree M, et al. Downstream targets of the homeobox gene DLX3 are differentially expressed in the placentae of pregnancies affected by human idiopathic fetal growth restriction. Mol Cell Endocrinol. 2013;377:75–83. doi:10.1016/j.mce.2013.06.032. PMID:23831639
- Cross JC, Simmons DG, Watson ED. Chorioallantoic morphogenesis and formation of the placental villous tree. Ann N Y Acad Sci. 2003;995:84–93. doi:10.1111/j.1749-6632.2003.tb03212.x. PMID:12814941
- Pepper MS. Transforming growth factor-beta: vasculogenesis, angiogenesis, and vessel wall integrity. Cytokine Growth Factor Rev. 1997;8:21–43. doi:10.1016/S1359-6101(96)00048-2.
- Aboagye-Mathiesen G, Zdravkovic M, Toth FD, et al. Altered expression of the tumor suppressor/oncoprotein p53 in SV40 Tag-transformed human placental trophoblast and malignant trophoblast cell lines. Early Pregnancy: Biology and Medicine: The Official Journal of the Society for the Investigation of Early Pregnancy. 1996;2:102–112. PMID:9363207
- Miettinen PJ, Ebner R, Lopez AR, et al. TGF-beta induced transdifferentiation of mammary epithelial cells to mesenchymal cells: involvement of type I receptors. J Cell Biol. 1994;127:2021–2036. doi:10.1083/jcb.127.6.2021. PMID:7806579
- Caniggia I, Grisaru-Gravnosky S, Kuliszewsky M, et al. Inhibition of TGF-beta 3 restores the invasive capability of extravillous trophoblasts in preeclamptic pregnancies. J Clin Invest. 1999;103:1641–1650. doi:10.1172/JCI6380. PMID:10377170
- Lyall F, Simpson H, Bulmer JN, et al. Transforming growth factor-beta expression in human placenta and placental bed in third trimester normal pregnancy, preeclampsia, and fetal growth restriction. Am J Pathol. 2001;159:1827–1838. doi:10.1016/S0002-9440(10)63029-5. PMID:11696443
- Murthi P, Doherty V, Said J, et al. Homeobox gene HLX1 expression is decreased in idiopathic human fetal growth restriction. Am J Pathol. 2006;168:511–518. doi:10.2353/ajpath.2006.050637. PMID:16436665
- Murthi P, Doherty VL, Said JM, et al. Homeobox gene ESX1L expression is decreased in human pre-term idiopathic fetal growth restriction. Mol Hum Reprod. 2006;12:335–340. doi:10.1093/molehr/gal037. PMID:16613891
- Murthi P, Said JM, Doherty VL, et al. Homeobox gene DLX4 expression is increased in idiopathic human fetal growth restriction. Mol Hum Reprod. 2006;12:763–769. doi:10.1093/molehr/gal087. PMID:17062780
- Pathirage NA, Cocquebert M, Sadovsky Y, et al. Homeobox gene transforming growth factor beta-induced factor-1 (TGIF-1) is a regulator of villous trophoblast differentiation and its expression is increased in human idiopathic fetal growth restriction. Mol Hum Reprod. 2013;19:665–675. doi:10.1093/molehr/gat042. PMID:23761267
- Rajaraman G, Murthi P, Pathirage N, et al. Downstream targets of homeobox gene HLX show altered expression in human idiopathic fetal growth restriction. Am J Pathol. 2010;176:278–287. doi:10.2353/ajpath.2010.090187. PMID:20008130
- Guaran RL, Wein P, Sheedy M, et al. Update of growth percentiles for infants born in an Australian population. Aust N Z J Obstet Gynaecol. 1994;34:39–50. doi:10.1111/j.1479-828X.1994.tb01037.x.
- Borges M, Bose P, Frank HG, et al. A two-colour fluorescence assay for the measurement of syncytial fusion between trophoblast-derived cell lines. Placenta. 2003;24:959–964. doi:10.1016/S0143-4004(03)00173-5. PMID:14580378
- Graham CH, Hawley TS, Hawley RG, et al. Establishment and characterization of first trimester human trophoblast cells with extended lifespan. Exp Cell Res. 1993;206:204–211. doi:10.1006/excr.1993.1139. PMID:7684692
- Livak KJ, Schmittgen TD. Analysis of relative gene expression data using real-time quantitative PCR and the 2(-Delta Delta C(T)) Method. Methods. 2001;25:402–408.