ABSTRACT
Meiosis produces haploid gametes by accurately reducing chromosome ploidy through one round of DNA replication and two subsequent rounds of chromosome segregation and cell division. The cell divisions of female meiosis are highly asymmetric and give rise to a large egg and two very small polar bodies that do not contribute to development. These asymmetric divisions are driven by meiotic spindles that are small relative to the size of the egg and have one pole juxtaposed against the cell cortex to promote polar body extrusion. An additional unique feature of female meiosis is that fertilization occurs before extrusion of the second polar body in nearly all animal species. Thus sperm-derived chromosomes are present in the egg during female meiosis. Here, we explore the idea that the asymmetry of female meiosis spatially separates the sperm from the meiotic spindle to prevent detrimental interactions between the spindle and the paternal chromosomes.
KEYWORDS:
Introduction
In most animals, fertilization occurs during female meiosis and before extrusion of the second polar body. In many cases, fertilization itself functions to reactivate an arrested oocyte to resume cell-cycle progression through female meiosis [Citation1]. In other cases, an event that occurs at the same time as fertilization initiates oocyte reactivation [Citation2,Citation3]. The exact point during female meiosis when fertilization occurs varies between species. Fertilization occurs during metaphase II in vertebrates [Citation4], during metaphase I in ascidians [Citation5], Drosophila [Citation3], starfish [Citation2], some molluscs [Citation6] and the annelid Chaetopterus [Citation7], during prometaphase I in C. elegans [Citation8,Citation9] and during late prophase in Urechis [Citation10] and other molluscs [Citation11]. Thus the egg must tolerate the presence of sperm-derived chromosomes while actively trying to reduce its ploidy for time periods ranging from 35 minutes in C. elegans [Citation9] to 2 hours in mouse [Citation12]. Direct interaction between the sperm contents and the meiotic spindle could cause a variety of scenarios leading to aneuploid or polyploid embryos. In this review, we discuss the necessity for maintaining a safe distance between sperm contents and the oocyte meiotic spindle as well as the mechanisms that ensure this safe distance.
Sperm poses three threats to female meiosis
The concept that the sperm is invasive and may be harmful to the egg has been proposed before in the context of RNAs contributed by the sperm [Citation13]. Here, we discuss the threat of the sperm interfering with accurate chromosome segregation during female meiosis.
Ectopic spindle formation
Sperm chromosomes consist of single chromatids that are destined to be replicated into sister chromatid pairs before proper segregation during the first mitosis. Thus incorporation of sperm chromatids into either an ectopic spindle or the female meiotic spindle could lead to segregation of some paternal chromosomes into a polar body. This would lead to embryos with a single copy of some chromosomes, a condition called monosomy, which is typically lethal. After normal fertilization, sperm chromatin induces formation of an abortive polar body called a fertilization cone in mouse zygotes [Citation14]. These abortive polar bodies regress before expelling paternal chromosomes because the sperm chromatin does not assemble a bipolar spindle, which is required to activate abscission of the polar body [Citation15]. During normal mouse fertilization, sperm chromatin is introduced to the M-phase oocyte cytoplasm at the same time that Ca2+ signaling activates the eggs to progress through anaphase and complete extrusion of the second polar body 1–2 hours later [Citation12]. However, if the time that the sperm chromatin incubates in M phase oocyte cytoplasm is extended by injecting sperm chromatin into metaphase-arrested oocytes and waiting 2–3 hours before chemically activating cell-cycle progression, an ectopic bipolar spindle forms. These ectopic bipolar spindles frequently expel all of the paternal chromatin into a polar body [Citation15]. Injection of sperm chromatin from immature sperm with less compact chromatin resulted in much faster bipolar spindle assembly (20–30 minutes) [Citation15]. Thus sperm chromatin must be modified during late stages of spermatogenesis in a manner that inhibits ectopic spindle assembly and thus prevents expulsion of paternal chromosomes into a polar body. Because chromatin-induced spindle assembly is driven by a RanGTP gradient [Citation16,Citation17] established by the chromatin-associated Ran-GEF, RCC1 [Citation18-20], removal of RCC1 from chromatin during sperm maturation and inhibition of RCC1 recruitment from the egg cytoplasm might be sufficient for preventing abscission of an ectopic polar body. However, mouse sperm chromatin normally induces fertilization cone assembly, which is RanGTP dependent [Citation21] suggesting that there must be a different inhibition mechanism at work in mice.
Incorporation of sperm chromatin into the oocyte meiotic spindle
If the sperm chromatin came into close proximity with the oocyte meiotic spindle, single sperm-derived chromatids could theoretically be incorporated into the meiotic spindle. As with an ectopic spindle, this could lead to expulsion of some paternal chromatids into a polar body, which would lead to lethal monosomy or haploidy. Most of the published data supporting this possibility comes from experiments incubating demembranated Xenopus sperm in Xenopus meiotic egg extracts [Citation22], an in vitro scenario that mimics fertilization. In these assays, sperm chromatids can assemble kinetochores [Citation23,Citation24] which might be captured by meiotic spindle microtubules even if ectopic spindle assembly was suppressed. When sperm chromatin induces ectopic spindle assembly in Xenopus egg extracts, these spindles can fuse together if they become close enough [Citation25]. These in vitro observations suggest that sperm chromatin has the potential for incorporating into the oocyte meiotic spindle if it is close enough.
Premature sperm aster formation and capture
A third potential threat from the sperm is generated by paternal centrioles. In most animals other than rodents, the sperm contributes two centrioles that will recruit egg proteins to form centrosomes [Citation26]. These centrosomes nucleate microtubule asters (sperm asters) to eventually capture the female pronucleus after extrusion of the second polar body and completion of female meiosis (). Many animals prevent potentially detrimental interactions between the sperm asters and the meiotic spindle by either suppressing sperm aster formation, by maintaining a sufficient distance between the sperm aster and the meiotic spindle, or both. In starfish, the sperm asters are limited in size until late meiosis [Citation27-28], whereas C. elegans, Urechis and oyster completely suppress aster formation [Citation27,Citation29,Citation30]. Inhibition of MAPK activation in starfish, oyster and Urechis all result in premature sperm aster formation [Citation27,Citation29]. In some cases, the sperm aster connected to the meiotic spindle [Citation27] but the overall consequences have not been evaluated because MAPK is required for multiple aspects of meiosis. The sperm-derived centrioles in C. elegans do not recruit egg proteins to form a centrosome and do not form a sperm aster until after extrusion of the second polar body (). This suppression of the centrioles requires kinesin-1 and its binding partner, KCA-1, both of which localize to a shell that surrounds the sperm DNA and centrioles during meiosis [Citation30]. Loss of kinesin-1 or KCA-1 results in premature centrosome maturation and sperm aster formation early in meiosis [Citation30] (). In the majority of kinesin-1/KCA-1-depleted zygotes, the sperm aster did not interact with the meiotic spindle because of the large distance between them. However, in a subset of zygotes, the premature sperm aster came within a 17 μm threshold distance of the meiotic spindle. In these cases, the spindle moved rapidly toward the sperm aster (). These spindle-capture events prevented extrusion of the second polar body thus generating triploid embryos [Citation30] (). These observations demonstrate the importance of maintaining a safe distance between the sperm aster and the meiotic spindle.
Figure 1. Sperm centriole and aster suppression, maturation and capture. (A-D) Sperm centrosome maturation in a wildtype C. elegans embryo. (A) Metaphase I meiotic embryo with silenced sperm centrioles. (B) Anaphase II meiotic embryo with silenced sperm centrioles. (C) Pronuclear stage embryo with mature sperm centrosomes and microtubule asters capturing the female pronucleus. (D) Pronuclear meeting with one pronucleus from each parent. (E-H) Premature centrosome maturation in a kca-1(RNAi) treated embryo. (E) Metaphase I meiotic embryo with sperm centrosomes starting to mature. (F) Anaphase II meiotic embryo with mature sperm centrosomes and microtubule asters forming. (G) Anaphase II meiotic embryo with mature sperm centrosomes and microtubule asters capturing the meiotic spindle and preventing polar body formation. (H) Pronuclear stage embryo with one male pronucleus and two female pronuclei from failed polar body formation.
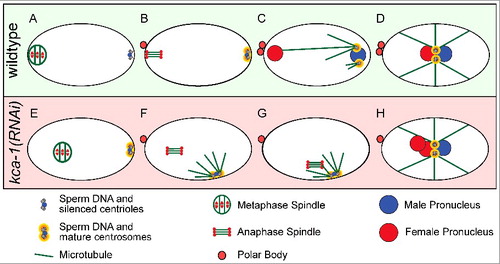
The regulation of sperm entry site
In fish and insects, the egg forms a narrow canal or micropyle through a thick envelope surrounding the egg to facilitate sperm entry at that precise location [Citation31,Citation32]. Whereas the micropyle is often precisely positioned, detailed studies of the positioning of the oocyte meiotic spindle relative to the micropyle have not been reported for most species. Studies of C. elegans and mouse, however, have revealed elegant but distinct mechanisms for ensuring a minimum initial distance between the sperm contents and the meiotic spindle.
C. elegans
In C. elegans, sperm are stored in a structure called the spermatheca, which physically separates the oocytes and the uterus (). The oldest unfertilized diakinesis-stage oocyte (-1 position) is adjacent to the spermatheca and matures in response to a secreted signal from the sperm [Citation33]. The germinal vesicle in the -1 oocyte first migrates away from the spermatheca in a kinesin-1 dependent manner [Citation34,Citation35] to assume a cortical position far from the spermatheca (). The spermathecal valve proximal to the oocyte then dilates to allow egg entry into the spermatheca and fertilization occurs at the point on the oocyte surface that first enters the spermathecal valve [Citation8]. The site of fertilization is thus on the opposite side of the zygote from the germinal vesicle. Nuclear envelope breakdown occurs before and during ovulation and the meiotic spindle assembles within the volume of the germinal vesicle and translocates to the closest point on the cortex [Citation9]. By the time the zygote passes the second spermathecal valve, the meiotic spindle is cortically positioned at the opposite end of the zygote from the sperm entry site (). Thus nuclear migration away from the spermatheca, and site of future sperm fusion, sets the initial distance between the sperm contents and the meiotic spindle.
Figure 2. Mechanisms governing sperm entry site. (A) Schematic of a C. elegans germline depicting the spatial separation of oocytes from sperm. Oocytes mature and increase in volume as they become closer to the spermatheca. Once an oocyte becomes the -1 oocyte, the germinal vesicle migrates to the cortex away from the spermatheca. The sperm will fertilize at the cortex closest to the spermathecal valve as it dilates. The sperm is on the opposite side of the meiotic spindle. Despite the cytoplasmic flows around the embryo, the sperm stays at that position. (B) Schematic of a metaphase II mouse oocyte. The meiotic spindle is positioned at the cortex by cytoplasmic flows. The cortical localization of the meiotic spindle prevents microvilli formation and sperm entry. (B) Schematic of a fertilized metaphase II mouse embryo depicting the sperm underlying the microvilli during cytoplasmic streaming.
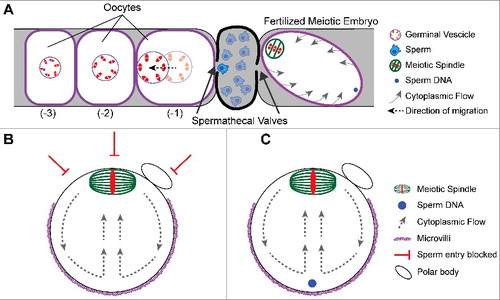
Mouse
Mouse oocytes initially have a centrally positioned germinal vesicle within an oocyte that is uniformly covered in microvilli. At germinal vesicle breakdown, the meiotic spindle assembles and migrates towards the cortex. This migration relies on dynamic cytoplasmic actin filaments [Citation36-38], where slow initial transport relies on nucleation by Formin-2 [Citation39-43] and Spire-1/Spire-2 [Citation44]. The spindle then migrates in a fast and directed manner that depends on cytoplasmic streaming induced by Arp2/3 actin filament nucleation [Citation45,Citation46] (). The cortical proximity of the chromosomes induces local reorganization of F-actin, which includes local disassembly of microvilli overlying the meiotic spindle. The spindle thus causes the cortex to polarize into two functional domains: the microvillar-rich zone and the microvillar-free zone over the spindle [Citation47,Citation48] (). Early studies identified the site of sperm-egg fusion within the microvillar-rich region of the egg surface [Citation38], and a later study showed by scanning EM that sperm only bind the microvillar region [Citation49]. Oocytes with altered or no microvilli exhibit impaired gamete fusion [Citation49], and oocytes that lack a microvillar-free zone, due to inhibition of FYN kinase, fuse with sperm proximal to the meiotic spindle [Citation50]. Thus, in mouse, migration of the meiotic spindle to the cortex generates a “do not fertilize here” zone and subsequently a distance from the sperm contents immediately after gamete fusion ().
Sperm positioning following fertilization
Despite initial positioning of the sperm contents far from the meiotic spindle, this positioning must be maintained in a manner that resists cytoplasmic streaming, a phenomenon that has been reported in oocytes of several species [Citation35,Citation46]. In C. elegans, microtubule-dependent cytoplasmic flows move the endoplasmic reticulum and yolk granules around the zygote in a circular pattern [Citation35,Citation51], whereas mouse oocytes utilize the actin cytoskeleton to drive cytoplasmic flows [Citation45,Citation46]. Cytoplasmic streaming in C. elegans stochastically changes direction with streaming occurring faster at the cortex [Citation51,Citation52]. In contrast, mouse cytoplasmic streaming is characterized by fountain flows, where cytoplasm pushes through the middle of the oocyte directly at the cortical meiotic spindle and then flows along the perimeter [Citation46]. The direction, velocity and duration of cytoplasmic streaming in both species would be sufficient to drive the sperm contents into the meiotic spindle in the absence of a mechanism to restrain movement of the sperm contents.
A recent study demonstrated that the sperm contents are tethered to the cortex to prevent transport with cytoplasmic flows in C. elegans [Citation52]. This cortical tether is comprised of actin filaments, which require some members of the formin family, but not non-muscle myosin. In control zygotes, the sperm DNA moved within a restricted volume near the site of fertilization as yolk granules streamed by. When actin was depolymerized, the sperm DNA moved long distances with the yolk granules. In the most severe cases of actin filament depletion, the sperm DNA could be found a few microns from the meiotic spindle, rather than the normal distance of 70% egg length away from the meiotic spindle. Under conditions that prevent streaming, the sperm stayed at the site of fertilization. The consequences of close sperm could not be properly assessed, since polar body formation does not occur in the absence of actin filaments. This study also revealed that the sperm DNA, centrioles and membranous organelles stay together as a unit during transport by cytoplasmic streaming when actin is depolymerized. This surprising result raises a number of questions. How are the sperm contents held together despite not being membrane bound? How do the sperm contents interact with maternal F-actin to become tethered? Is there a biological purpose for maintaining the sperm DNA, centrioles and its membranous organelles as a discrete “cell within a cell” during female meiosis? The cohesion of the sperm contents could function as a gelatinous barrier to prevent incorporation of sperm DNA into the meiotic spindle or to prevent expulsion of sperm chromatids into a polar body. The 5 μm average diameter of the cloud of sperm-derived organelles is similar the closest distance observed between untethered sperm DNA and the meiotic spindle. In these embryos, the cloud of sperm contents was likely in direct contact with the meiotic spindle but sperm chromatids were still not incorporated into the spindle.
While this C. elegans study is the first to describe a mechanism maintaining sperm position after fertilization but before polar body extrusion, there are hints in the literature that different mechanisms exist in other organisms. A study in ascidian eggs revealed that sperm entry occurs at the animal pole, which is also the location of the meiotic spindle [Citation53]. However, five minutes after fertilization the sperm DNA is found in the vegetal hemisphere and away from the meiotic spindle. This suggests that the sperm DNA is actively transported away from the meiotic spindle in ascidians. Another study in starfish zygotes found that the distance between the sperm contents and the meiotic spindle remained constant even when the zygote was flattened by compression. This result suggested that starfish zygotes have a mechanism for maintaining a fixed distance between the meiotic spindle and sperm contents [Citation28]. Lastly, the actin-based fertilization cone [Citation14] in mouse may be a structure functioning similarly to the cortical actin tether in C. elegans [Citation52].
Conclusions and perspectives
While other mechanisms maintaining a minimum distance between the sperm contents and the meiotic spindle have yet to be investigated in other species, we anticipate that the general phenomenon of maintaining this distance will be conserved among animals. Eggs that are fertilized while they have a centrally positioned germinal vesicle, like Spisula solidissima [Citation54],might move the meiotic spindle away from the sperm contents. Alternatively, animals with large eggs might utilize the sperm asters to position the sperm contents in the center of the zygote, by mechanisms known to self center sperm asters after meiosis [Citation55]. This would ensure that the sperm contents are always half the egg diameter away from the cortical meiotic spindle. It will be interesting to see other mechanisms of sperm positioning during female meiosis emerge in the field.
Disclosure of Potential Conflicts of Interest
No potential conflicts of interest were disclosed.
Additional information
Funding
References
- Kashir J, Deguchi R, Jones C, et al. Comparative biology of sperm factors and fertilization-induced calcium signals across the animal kingdom. Mol Reprod Dev. 2013;80:787–815. 10.1002/mrd.22222. PMID:23900730
- Harada K, Oita E, Chiba K. Metaphase I arrest of starfish oocytes induced via the MAP kinase pathway is released by an increase of intracellular pH. Development. 2003;130:4581–4586. 10.1242/dev.00649. PMID:12925585
- Heifetz Y, Yu J, Wolfner MF. Ovulation triggers activation of Drosophila oocytes. Dev Biol. 2001;234:416–424. 10.1006/dbio.2001.0246. PMID:11397010
- Tunquist BJ, Maller JL. Under arrest: cytostatic factor (CSF)-mediated metaphase arrest in vertebrate eggs. Genes Dev. 2003;17:683–710. 10.1101/gad.1071303. PMID:12651887
- Marino M, Wilding M, Dale B. Interaction of cell cycle kinases, microtubules, and chromatin in ascidian oocytes during meiosis. Mol Reprod Dev. 2000;56:155–162. 10.1002/(SICI)1098-2795(200006)56:2%3c155::AID-MRD6%3e3.0.CO;2-1. PMID:10813847
- Deguchi R, Osanai K. Repetitive intracellular Ca2+ increases at fertilization and the role of Ca2+ in meiosis reinitiation from the first metaphase in oocytes of marine bivalves. Dev Biol. 1994;163:162–174. 10.1006/dbio.1994.1132. PMID:8174771
- Eckberg WR, Miller AL. Propagated and nonpropagated calcium transients during egg activation in the annelid, Chaetopterus. Dev Biol. 1995;172:654–664. 10.1006/dbio.1995.8043. PMID:8612979
- Takayama J, Onami S. The sperm TRP-3 channel mediates the Onset of a Ca(2+) Wave in the fertilized C. elegans Oocyte Cell Rep. 2016;15:625–637. PMID:27068469
- Yang HY, McNally K, McNally FJ. MEI-1/katanin is required for translocation of the meiosis I spindle to the oocyte cortex in C elegans. Dev Biol. 2003;260:245–259. 10.1016/S0012-1606(03)00216-1. PMID:12885567
- Stephano JL, Gould MC. The intracellular calcium increase at fertilization in Urechis caupo oocytes: activation without waves. Dev Biol. 1997;191:53–68. 10.1006/dbio.1997.8709. PMID:9356171
- Deguchi R, Osanai K. Meiosis reinitiation from the first prophase is dependent on the levels of intracellular Ca2+ and pH in oocytes of the bivalves Mactra chinensis and Limaria hakodatensis. Dev Biol. 1994;166:587–599. 10.1006/dbio.1994.1339. PMID:7813778
- Deguchi R, Shirakawa H, Oda S, et al. Spatiotemporal analysis of Ca(2+) waves in relation to the sperm entry site and animal-vegetal axis during Ca(2+) oscillations in fertilized mouse eggs. Dev Biol. 2000;218:299–313. 10.1006/dbio.1999.9573. PMID:10656771
- Miller D. Confrontation, consolidation, and recognition: The Oocyte's perspective on the incoming sperm. Cold Spring Harb Perspect Med. 2015;5:a023408. 10.1101/cshperspect.a023408. PMID:25957313
- Maro B, Johnson MH, Pickering SJ, et al. Changes in actin distribution during fertilization of the mouse egg. J Embryol Exp Morphol. 1984;81:211–237. PMID:6540795
- Deng M, Li R. Sperm chromatin-induced ectopic polar body extrusion in mouse eggs after ICSI and delayed egg activation. PLoS One. 2009;4:e7171. 10.1371/journal.pone.0007171. PMID:19787051
- Kalab P, Weis K, Heald R. Visualization of a Ran-GTP gradient in interphase and mitotic Xenopus egg extracts. Science. 2002;295:2452–2456. 10.1126/science.1068798. PMID:11923538
- Ohba T, Nakamura M, Nishitani H, et al. Self-organization of microtubule asters induced in Xenopus egg extracts by GTP-bound Ran. Science. 1999;284:1356–1358. 10.1126/science.284.5418.1356. PMID:10334990
- Halpin D, Kalab P, Wang J, et al. Mitotic spindle assembly around RCC1-coated beads in Xenopus egg extracts. PLoS Biol. 2011;9:e1001225. 10.1371/journal.pbio.1001225. PMID:22215983
- Li HY, Zheng Y. Phosphorylation of RCC1 in mitosis is essential for producing a high RanGTP concentration on chromosomes and for spindle assembly in mammalian cells. Genes Dev. 2004;18:512–527. 10.1101/gad.1177304. PMID:15014043
- Carazo-Salas RE, Guarguaglini G, Gruss OJ, et al. Generation of GTP-bound Ran by RCC1 is required for chromatin-induced mitotic spindle formation. Nature. 1999;400:178–181. 10.1038/22133. PMID:10408446
- Deng M, Suraneni P, Schultz RM, et al. The Ran GTPase mediates chromatin signaling to control cortical polarity during polar body extrusion in mouse oocytes. Dev Cell. 2007;12:301–308. 10.1016/j.devcel.2006.11.008. PMID:17276346
- Heald R, Tournebize R, Blank T, et al. Self-organization of microtubules into bipolar spindles around artificial chromosomes in Xenopus egg extracts. Nature. 1996;382:420–425. 10.1038/382420a0. PMID:8684481
- Desai A, Deacon HW, Walczak CE, et al. A method that allows the assembly of kinetochore components onto chromosomes condensed in clarified Xenopus egg extracts. Proc Natl Acad Sci USA. 1997;94:12378–12383. 10.1073/pnas.94.23.12378. PMID:9356457
- Emanuele MJ, Lan W, Jwa M, et al. Aurora B kinase and protein phosphatase 1 have opposing roles in modulating kinetochore assembly. J Cell Biol. 2008;181:241–254. 10.1083/jcb.200710019. PMID:18426974
- Gatlin JC, Matov A, Groen AC, et al. Spindle fusion requires dynein-mediated sliding of oppositely oriented microtubules. Curr Biol. 2009;19:287–296. 10.1016/j.cub.2009.01.055. PMID:19230671
- Schatten G. The centrosome and its mode of inheritance: the reduction of the centrosome during gametogenesis and its restoration during fertilization. Dev Biol. 1994;165:299–335. 10.1006/dbio.1994.1256. PMID:7958403
- Stephano JL, Gould MC. MAP kinase, a universal suppressor of sperm centrosomes during meiosis? Dev Biol. 2000;222:420–428. 10.1006/dbio.2000.9726. PMID:10837129
- Kitajima A, Hamaguchi Y. Determination of first cleavage plane: the relationships between the orientation of the mitotic apparatus for first cleavage and the position of meiotic division-related structures in starfish eggs. Dev Biol. 2005;280:48–58. 10.1016/j.ydbio.2004.12.033. PMID:15766747
- Gould MC, Stephano JL. MAP kinase, meiosis, and sperm centrosome suppression in Urechis caupo. Dev Biol. 1999;216:348–358. 10.1006/dbio.1999.9468. PMID:10588884
- McNally KL, Fabritius AS, Ellefson ML, et al. Kinesin-1 prevents capture of the oocyte meiotic spindle by the sperm aster. Dev Cell. 2012;22:788–798. 10.1016/j.devcel.2012.01.010. PMID:22465668
- Hart N, Donovan M. Fine structure of the chorion and site of sperm entry in the egg of Brachydanio. Journal of Experimental Zoology.1983:277–296.
- Yanagimachi R, Cherr G, Matsubara T, et al. Sperm attractant in the micropyle region of fish and insect eggs. Biol Reprod. 2013;88:47. 10.1095/biolreprod.112.105072. PMID:23303675
- Miller MA, Nguyen VQ, Lee MH, et al. A sperm cytoskeletal protein that signals oocyte meiotic maturation and ovulation. Science. 2001;291:2144–2147. 10.1126/science.1057586. PMID:11251118
- McCarter J, Bartlett B, Dang T, et al. On the control of oocyte meiotic maturation and ovulation in Caenorhabditis elegans. Dev Biol. 1999;205:111–128. 10.1006/dbio.1998.9109. PMID:9882501
- McNally KL, Martin JL, Ellefson M, et al. Kinesin-dependent transport results in polarized migration of the nucleus in oocytes and inward movement of yolk granules in meiotic embryos. Dev Biol. 2010;339:126–140. 10.1016/j.ydbio.2009.12.021. PMID:20036653
- Azoury J, Lee KW, Georget V, et al. Spindle positioning in mouse oocytes relies on a dynamic meshwork of actin filaments. Curr Biol. 2008;18:1514–1519. 10.1016/j.cub.2008.08.044. PMID:18848445
- Longo FJ, Chen DY. Development of cortical polarity in mouse eggs: involvement of the meiotic apparatus. Dev Biol. 1985;107:382–394. 10.1016/0012-1606(85)90320-3. PMID:4038667
- Verlhac MH, Lefebvre C, Guillaud P, et al. Asymmetric division in mouse oocytes: with or without Mos. Curr Biol. 2000;10:1303–1306. 10.1016/S0960-9822(00)00753-3. PMID:11069114
- Azoury J, Lee KW, Georget V, et al. Symmetry breaking in mouse oocytes requires transient F-actin meshwork destabilization. Development. 2011;138:2903–2908. 10.1242/dev.060269. PMID:21653611
- Dumont J, Million K, Sunderland K, et al. Formin-2 is required for spindle migration and for the late steps of cytokinesis in mouse oocytes. Dev Biol. 2007;301:254–265. 10.1016/j.ydbio.2006.08.044. PMID:16989804
- Leader B, Lim H, Carabatsos MJ, et al. Formin-2, polyploidy, hypofertility and positioning of the meiotic spindle in mouse oocytes. Nat Cell Biol. 2002;4:921–928. 10.1038/ncb880. PMID:12447394
- Schuh M, Ellenberg J. A new model for asymmetric spindle positioning in mouse oocytes. Curr Biol. 2008;18:1986–1992. 10.1016/j.cub.2008.11.022. PMID:19062278
- Li H, Guo F, Rubinstein B, et al. Actin-driven chromosomal motility leads to symmetry breaking in mammalian meiotic oocytes. Nat Cell Biol. 2008;10:1301–1308. 10.1038/ncb1788. PMID:18836438
- Pfender S, Kuznetsov V, Pleiser S, et al. Spire-type actin nucleators cooperate with Formin-2 to drive asymmetric oocyte division. Curr Biol. 2011;21:955–960. 10.1016/j.cub.2011.04.029. PMID:21620703
- Yi K, Rubinstein B, Unruh JR, et al. Sequential actin-based pushing forces drive meiosis I chromosome migration and symmetry breaking in oocytes. J Cell Biol. 2013;200:567–576. 10.1083/jcb.201211068. PMID:23439682
- Yi K, Unruh JR, Deng M, et al. Dynamic maintenance of asymmetric meiotic spindle position through Arp2/3-complex-driven cytoplasmic streaming in mouse oocytes. Nat Cell Biol. 2011;13:1252–1258. 10.1038/ncb2320. PMID:21874009
- Eager DD, Johnson MH, Thurley KW. Ultrastructural studies on the surface membrane of the mouse egg. J Cell Sci. 1976;22:345–353. PMID:1033938
- Johnson MH, Eager D, Muggleton-Harris A, et al. Mosaicism in organisation concanavalin A receptors on surface membrane of mouse egg. Nature. 1975;257:321–322. 10.1038/257321a0. PMID:1172195
- Runge KE, Evans JE, He ZY, et al. Oocyte CD9 is enriched on the microvillar membrane and required for normal microvillar shape and distribution. Dev Biol. 2007;304:317–325. 10.1016/j.ydbio.2006.12.041. PMID:17239847
- Luo J, McGinnis LK, Kinsey WH. Fyn kinase activity is required for normal organization and functional polarity of the mouse oocyte cortex. Mol Reprod Dev. 2009;76:819–831. 10.1002/mrd.21034. PMID:19363790
- Kimura K, Mamane A, Sasaki T, et al. Endoplasmic-reticulum-mediated microtubule alignment governs cytoplasmic streaming. Nat Cell Biol. 2017;19:399–406. 10.1038/ncb3490. PMID:28288129
- Panzica MT, Marin HC, Reymann AC, et al. F-actin prevents interaction between sperm DNA and the oocyte meiotic spindle in C. elegans J Cell Biol. 2017;216:2273–2282. 10.1083/jcb.201702020. PMID:28637747
- Speksnijder JE, Jaffe LF, Sardet C. Polarity of sperm entry in the ascidian egg. Dev Biol. 1989;133:180–184. 10.1016/0012-1606(89)90309-6. PMID:2468542
- Allen RD. Fertilization and artificial activation in the egg of the surf-clam, Spisula solidissima. The Biological Bulletin. 1953;105:213–239. 10.2307/1538639.
- Mitchison T, Wuhr M, Nguyen P, et al. Growth, interaction, and positioning of microtubule asters in extremely large vertebrate embryo cells. Cytoskeleton (Hoboken). 2012;69:738–750. 10.1002/cm.21050. PMID:22786885