ABSTRACT
In previous work, we established an equine induced pluripotent stem cell line (E-iPSCs) from equine adipose-derived stem cells (ASCs) using a lentiviral vector encoding four transcription factors: Oct4, Sox2, Klf4, and c-Myc. In the current study, we attempted to differentiate these established E-iPSCs into mesenchymal stem cells (MSCs) by serial passaging using MSC-defined media for stem cell expansion. Differentiation of the MSCs was confirmed by analyzing expression levels of the MSC surface markers CD44 and CD29, and the pluripotency markers Nanog and Oct4. Results indicated that the E-iPSC-derived MSCs (E-iPSC-MSCs) retained the characteristics of MSCs, including the ability to differentiate into chondrogenic, osteogenic, or myogenic lineages. E-iPSC-MSCs were rendered suitable for therapeutic use by inhibiting immune rejection through exposure to transforming growth factor beta 2 (TGF-β2) in culture, which down-regulated the expression of major histocompatibility complex class I (MHC class I) proteins that cause immune rejection if they are incompatible with the MHC antigen of the recipient. We reported 16 cases of E-iPSC-MSC transplantations into injured horses with generally positive effects, such as reduced lameness and fraction lines. Our findings indicate that E-iPSC-MSCs can demonstrate MSC characteristics and be safely and practically used in the treatment of musculoskeletal injuries in horses.
Introduction
Stem cells have gained recent attention in veterinary biomedical research for their use as potential therapeutic agents for musculoskeletal injuries or disorders. Mesenchymal stem cells (MSCs), embryonic stem cells (ESCs), and induced pluripotent stem cells (iPSCs) have been investigated as potential stem cell therapy resources, with MSCs generating interest from the scientific community for use in regenerative medicine due to high yields, non-invasive collection methods, and suitable plasticity and immunomodulatory properties. Conversely, the use of undifferentiated iPSCs carries safety concerns that limit their therapeutic applicability [Citation1]. MSCs are multipotent stem cells present in adult tissues that can be collected easily from muscles, adipose, or other organ tissues. The iPSCs are the most promising of these cells owing to their pluripotency; these cells present with plasticity similar to that of embryonic stem cells (ESCs) but without the attendant controversial origins. These iPSCs can be differentiated into MSCs relatively easily [Citation2].
Pluripotent stem cells express a unique set of factors responsible for their pluripotency, and an interconnected network of regulatory genes is responsible for the development and maintenance of pluripotency in the embryo [Citation3]. These regulatory genes include four transcription factors, OCT4, Sox2, c-Myc, and Klf4 (OSKM), encoded in the genome of mouse and human cells [Citation4,Citation5]. The use of these reprogrammed iPSCs does not present ethical concerns nor problems with rejection after transplantation [Citation4,Citation6]. Currently, many diverse factors, such as integrative viral vectors and non-integrative adenovirus, episomal plasmids, proteins, mRNA, and chemical compounds, have been tried for implantation of iPSCs. The pluripotency of iPSCs is demonstrated by their ability to form teratomas in vivo and embryonic bodies in vitro. The molecular profile of iPSCs is similar to that of ESCs, but not the same, as iPSCs express the pluripotency markers OCT4, NANOG, SOX2, SSEA1, SSEA3, SSEA4, RA1-60, and TRA1-81 and possess ALP activities. Many studies have confirmed that these pluripotent markers are expressed in reprogrammed cells from different species, including humans, mice, rhesus monkeys, pigs, rabbits, sheep, cats, and equines [Citation7]. The generation of iPSCs requires the demethylation of specific transcriptional factors to ensure successful reprogramming of the cells [Citation8]. In a previous study, we generated iPSCs by expressing Sox2, Oct4, c-Myc, and Klf4 in source cells originating from equine adipose-derived stem cells (ASCs) [Citation9], and the reprogramming of MSCs into iPSCs using ASCs was 200 times more efficient than that observed with fibroblasts [Citation10].
MSCs can differentiate into several cell types from the mesodermal germ layer, including adipocytes, osteocytes, and chondrocytes [Citation11,Citation12], and their advantages for clinical application include their easy isolation, high yield, high plasticity, and lack of teratoma formation after engrafting, thus ensuring the safety of the host. MSCs are heterogeneous, have an immunophenotype profile, and plasticity that varies among species, sources, and passages. These cells also express the surface markers CD29, CD73, CD90, CD105, CD44, and CD166, and do not express CD14, CD31, CD34, and CD45 [Citation13]. As such, MSCs are frequently used in cell therapies, and the aim of our study was to obtain MSC-like cells from equine induced pluripotent stem cells (E-iPSCs) for use in equine injury treatment.
Horses are companion animals that are closely linked to humans. Historically, horses were raised for work, such as agriculture and transportation, and later for entertainment and sports. As horseracing and other sports with horses can be quite aggressive, these horses tend to develop injuries to the musculoskeletal system. Stem cells can differentiate into many cell types [Citation14], and multipotent stem cells have been used in many medical research applications and can be developed as potent therapeutic agents.
Stem cells have gained recent attention in human and veterinary biomedical research due to their potential as therapeutic agents for musculoskeletal injuries and disorders, and MSCs, ESCs, and iPSCs have all been actively studied as cell sources for use in horses. In addition to their promise for use in veterinary medicine, E-iPSCs have opened up the possibility of using horses for validating stem cell-based therapies before their application in human clinical settings. Research on the differentiation of iPSCs into other cell types, including osteoblasts and myocytes, is ongoing, in efforts to advance the field of regenerative medicine for application to musculoskeletal injuries [Citation15,Citation16].
Skeletal muscles comprise roughly 40% of the mass of the human body and are important for normal body function and homeostasis. These muscles produce force for locomotion, breathing, and postural support, and provide heat during cold stress. Skeletal muscle is also a major regulator of blood glucose levels, and impaired insulin signaling in skeletal muscles can lead to type II diabetes [Citation17–Citation19].
The frequency of orthopedic diseases and injuries in horses has rendered them the primary focus of clinical studies on equine stem cells. Orthopedic tissues, including tendons and cartilage, have poor intrinsic regenerative capacities and frequently produce fibrotic tissue after injury or inflammation. Fibrotic tissue impairs the proper function of native tissues and can lead to recurring injuries and compromised athletic performance. Current biomedical researchers have investigated stem cell therapies as therapeutic tools in efforts to improve tissue regeneration and mitigate or prevent fibrosis [Citation20,Citation21].
In this study, we established MSC-like cells from E-iPSCs by culturing them in a specialized defined medium for 35 days. We then isolated these MSC-like cells and assessed whether they were functionally similar to MSCs, to develop cells that were safe for transplantation in horses for treating muscle injuries. MSCs derived from iPSCs are an excellent source of multipotent cells, as iPSCs incorporate both iPSCs and traditional MSC cells. The E-iPSC-derived MSCs demonstrated favorable efficiency in terms of proliferation and differentiation and possessed immunomodulatory properties similar to those of traditional MSCs. The E-iPSC-MSC cell line in this study was established for stem cell therapies following in vitro pre-differentiation before engraftment, thus ensuring their safe use.
Materials and methods
Generation of E-iPSCs from E-ASCs
Equine adipose-derived stem cells (E-ASCs) were isolated from the equine abdominal area, and E-iPSCs were generated as previously reported [Citation9]. Briefly, plasmid TetO-FUW-OSKM containing the Oct4, Sox2, Klf4, and c-Myc genes and plasmid FUW-M2rtTA containing the M2rtTA gene were purchased from Addgene (Cambridge, USA). Then, we developed lentiviral vectors carrying OSKM and M2rtTA using the ViraPowerTM Lentiviral Packaging Mix (Invitrogen, USA) in accordance with the manufacturer’s instructions. For viral transduction, 1 × 105 equine ASCs were seeded onto a 100 mm culture dish and incubated overnight with equal volumes of lentiviral supernatants (OSKM and M2rtTA) and 10 ng/mL polybrene (Santa Cruz Biotechnology, USA). Transduced equine ASCs were cultured in ESC medium containing high-glucose DMEM (Gibco, USA), 20% FBS, 1 U of 1% penicillin/streptomycin solution, 1X MEM non-essential amino acids solution (MEM-NEAA; Gibco, USA), 1X GlutaMAX (Gibco, USA), LIF (Millipore, Australia), 0.1% mercaptoethanol (Gibco, USA), and 2 μg/mL doxycycline hyclate (Dox; Sigma-Aldrich, USA). The medium was refreshed every other day after viral transduction, and colonies were selected and plated onto mitomycin C (Sigma-Aldrich, USA)-treated mouse embryonic fibroblast (MEF)-seeded culture dishes for 18 to 30 days after viral transduction. A total of 10 mg/mL Type IV collagenase (Gibco, USA) in DPBS was used for the passages [Citation8].
Differentiation of E-iPSCs into E-MSC-like cells
A serial plating strategy was used to differentiate E-iPSCs into MSC-like cells [Citation22]. Briefly, E-iPSCs were seeded onto gelatin-coated plates at 1 × 104 cells/cm2 in MSC induction media (CEFO, South Korea) comprising 500 to 1000 mg/L of glucose, 3 to 5 mg/L of insulin, 0.3 to 5 pg/L of sodium selenite, 2.7 to 3.6 mg/L of transferrin, 0.01 to 0.03 mg/L of VEGF, and 0.1 to 0.5 mg/L of biotin. The cells were cultured to 80% confluence and then passaged onto a new dish. For passaging, trypLE select (1X) was added to the cells and followed by incubation for 3 to 5 min at 37ºC.
Osteogenic, chondrogenic, and myogenic differentiation of E-MSC-like cells
Tri-lineage differentiation experiments were performed wherein equine MSC-like cells were challenged to undergo osteogenesis, chondrogenesis, and myogenesis to demonstrate the differentiation capacity of equine MSC-like cells generated from E-iPSCs. For myogenic differentiation, 5% horse serum, 1% antibiotics, 10 ng/mL basic FGF, and 100 nM dexamethasone supplemented with high-glucose DMEM, and the cells were passaged into new dishes when their proliferation reached 80% confluence. For osteogenic differentiation, a StemPro Osteogenesis kit (Thermo Fisher, USA) was used, and for chondrogenic differentiation, a StemPro Chondrogenesis kit (Thermo Fisher, USA) was used.
Fluorescence-activated cell sorting (FACS) analysis
FACS analysis was performed to evaluate the differentiation efficiency of E-iPSCs into MSC-like cells. Ten days after the induction of differentiation, differentiated E-iPSCs were harvested from the culture dish, and 5 × 105 cells were resuspended in 200 μL of PBS. The cells were stained using an anti-human/equine integrin β1/CD29 monoclonal antibody (R&D Systems, USA), followed by a fluorescein isothiocyanate (FITC)-conjugated anti-mouse IgG secondary antibody (Invitrogen, USA), and FITC rat anti-mouse CD44 (BD Bioscience, USA). Isotype-matched normal rat IgG was used as the control.
RT-PCR and quantitative PCR
Total RNA was extracted from cells using Trizol reagent (Invitrogen, USA), and cDNA was then synthesized from the extracted RNA using the Maxima Enzyme Mix (Thermo Fisher, USA) in accordance with the manufacturer’s instructions. Expression levels of genes related to pluripotency, MSC surface presentation, and tri-lineage (myogenic, chondrogenic, or osteogenic) differentiation were assessed via reverse transcription-polymerase chain reaction (RT-PCR) and quantitative-PCR (qPCR) using the primers listed in .
Table 1. PCR primers
Immunoblot analysis
Immunoblot analysis was used to confirm expression of the appropriate markers for E-ASCs, E-iPSCs, and E-iPSC-MSCs. Each type of cell was incubated with a blocking solution for 1 h and then with primary antibodies overnight. The next day, after washing, cells were incubated with HRP-conjugated secondary antibodies for 1 h before detection. The primary antibodies used were CD44 (OriGene, USA), CD29 (R&D Systems, USA), Sox2 (Santa Cruz, USA), SSEA-1, RUNX2 (Santa Cruz, USA), aggrecan (Invitrogen, USA), and Myf5 (Santa Cruz, USA) at a dilution ratio of 1:1000. For secondary antibodies, we used goat anti-mouse IgM-HRP (Cell Signaling, USA) and anti-rabbit IgM-HRP (Cell Signaling, USA) at a dilution ratio of 1:2000 to 1:4000.
Immunofluorescence
Immunofluorescence was used to evaluate the expression of iPSC- and MSC-related markers in E-iPSCs and E-iPSC-MSCs, respectively. Cells were cultured up to 70% confluency on a coverslip and then fixed using 4% paraformaldehyde. A blocking solution of 3% BSA in PBS was applied, and primary antibodies were diluted in PBS containing 1% BSA. The antibodies used for assessing MSC markers were CD44 (OriGene, USA) and CD29 (R&D System, USA). The antibodies used for assessing the iPSC markers were SSEA-1 (Santa Cruz, USA) and SOX2 (Santa Cruz, USA). The antibodies used for assessing the tri-lineage differentiation were OPN (Santa Cruz, USA), aggrecan (Invitrogen, USA), and myogenin (Santa Cruz, USA). The fluorescence-conjugated secondary antibodies used were anti-mouse Alexa Fluor 488, anti-rat Alexa Fluor 288, anti-rabbit Alexa Fluor 555, and donkey anti-goat Alexa Fluor 555.
Cell preparation for transplantation
Equine cells were prepared for transplantation by culturing in a 100 mm cell culture dish for 2 to 3 days after seeding. About 1.0 × 107 cells were transferred to a syringe. Cells were treated with 3 mL of trypsin-EDTA for 3 to 5 min at 37ºC to detach them after aspirating the media. Cells were then neutralized with high-glucose DMEM containing 10% FBS with 1% P/S and centrifuged for 3 min at 2500 rpm. The total number of cells was counted with a hemocytometer up to 1.0 × 107. Cells then were suspended into CB-CEFO-vive (CEFO, Republic of Korea), a cell storage solution with PBS at a ratio of 1:2 to 1:3, and subsequently transferred to a 10 mL syringe for delivery. Cell injection was performed by a licensed veterinarian from the Korean Horse Affairs Association. Animal experiments were conducted in accordance with the NIH guidelines for the care and use of laboratory animals and with approval from the Institutional Animal Care and Use Committee of the Kyungpook National University (KNU 2013–0082). Sixteen thoroughbreds owned by the Korea Racing Authority (KRA) were included in the study, comprising 11 males and 6 females, all 1 to 18 years of age. All injections were performed by the same veterinarian. Individuals were sedated prior to the injection via IV administration of detomidine at 0.01 mg/kg after aseptic preparation of the skin. Injections were performed into each horse’s lesion with a 20-gauge needle. Each horse received 10 mL containing E-iPSC-MSCs in a transport medium. All horses received regular clinical follow-ups with radiographic and ultrasonographic examinations over the four months following treatment.
Statistical analysis
All values are presented as mean ± SEM. Statistical analysis were determined using one-way analysis of variance (ANOVA) followed by Tukey’s multiple comparison test. Values for statistical significance were set at *P less than 0.05, **P less than 0.01, or ***P less than 0.001.
Results
E-ipsc colony formation and MSC differentiation
We introduced the four factors Oct4, Sox2, Klf4, and c-Myc to develop E-iPSCs into E-ASCs via a lentiviral vector and plated the cells on an MEF feeder layer. Transduced cells aggregated on feeder cells and started forming colonies at day 9 after transduction [Citation9], and colonies were grown and proliferated to undifferentiated status [Citation8].
E-iPSC colonies were isolated and passaged every 5 days to generate MSCs from E-iPSCs and differentiation of iPSCs into MSC-like cells was observed; these cells exhibited altered cell morphology with spindle shape and comparably larger cytoplasm 10 days after applying the MSC induction media (CEFO, Republic of Korea) ().
Figure 1. Differentiation of E-iPSCs into MSC-like E-iPSC-MSCs.
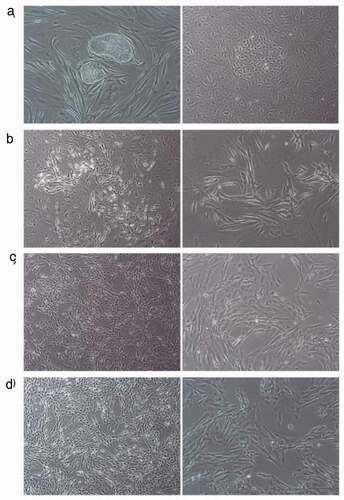
Confirmation of E-iPSC-MSC mesenchymal characteristics via mRNA analysis
Differentiation into E-MSCs from E-iPSCs was confirmed by assessing the expression of marker genes for each cell type: E-ASCs, E-iPSCs, and E-iPSC-MSCs (). Pluripotency of the iPSCs and differentiation into MSCs were determined by testing for the expression of Nanog and Oct4, and of CD44 and CD29, respectively, through RT-PCR analysis. Expression of CD44 and CD29 was apparent in E-ASCs and E-MSCs, whereas bands for Nanog and Oct4 were observed in the E-iPSCs (). These results indicated a successful establishment of E-MSCs from E-iPSCs at the mRNA level.
Figure 2. mRNA expression of iPSC and MSC markers.
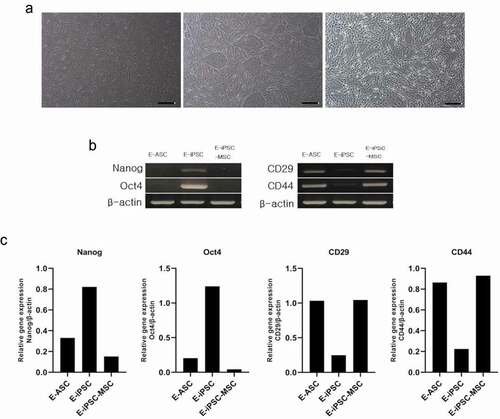
Confirmation of E-iPSC-MSC mesenchymal characteristics via protein analysis
To verify whether the E-iPSC-induced E-MSCs possessed MSC characteristics, we analyzed the expression of the MSC-associated genes CD29 and CD44 at the protein level. Clear CD44 bands in E-ASCs and E-MSCs confirmed that the E-iPSCs generated from E-ASCs had differentiated into MSCs. We also analyzed the expressions of the pluripotency markers Oct3/4 and SSEA-1 and the hematopoietic stem cell marker CD34, and expression of Oct3/4 and SSEA-1 was only detected in the E-iPSCs; E-ASCs, E-iPSCs, and E-iPSC-MSCs did not express CD34 (). We used FACS analysis to confirm the characteristics of equine cells and found that more than 90% of the cells were positive for CD29 and CD44 (). We then checked the expression of these proteins via immunofluorescence and obtained similar results, in that both E-ASC and E-iPSC-MSC were positive for CD29 and CD44 expression, whereas SSEA-1 was not expressed in either cell type (). These results confirmed that the E-iPSCs had differentiated into E-MSCs.
Figure 3. Protein expression of iPSC and MSC markers.
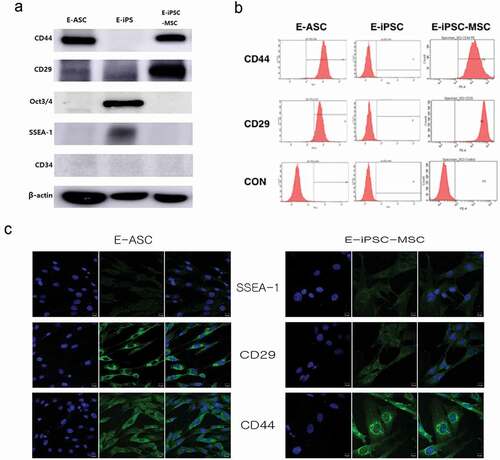
E-iPSC-MSCs are more proliferative than E-ASCs
We performed cell cycle analysis using flow cytometry to evaluate the proliferative capacity of E-iPSC-MSCs. The cell cycle statuses of E-ASCs and E-iPSC-MSCs were assessed by analyzing the cells using flow cytometry, and a 60.9% G0/G1 ratio in E-iPSC-MSCs and 71.0% in E-ASCs were observed. Lower G0/G1 percentages indicate a more proliferative status, and we thus concluded that E-iPSC-MSCs were more proliferative than E-ASCs ().
E-iPSC-MSCs differentiate into osteogenic, chondrogenic, and myogenic cells
A significant feature of MSCs is multi-potency, or the capacity to differentiate into multiple cell types, including osteocytes, chondrocytes, myocytes, and adipocytes [Citation14]. We attempted to differentiate our E-iPSC-MSCs into osteogenic, chondrogenic, and myogenic cell lineages using osteogenic, chondrogenic, and myogenic differentiation media, respectively, to check if our E-iPSC-MSCs qualified as functional MSCs,. Differentiation was determined by qPCR analysis using specific markers for each cell type. Specifically, we assessed the expression of RUNX2 and osteonectin for osteogenic differentiation, the expression of aggrecan and Sox9 for chondrogenic differentiation, and the expression of Pax7, myogenin, and myoD for myogenic differentiation (). Results indicated that the expression of Runx2 had increased more than two-fold at 20 h after treatment with osteogenic differentiation media. The expression of osteonectin also showed a marginal increase of about 1.5-fold at 20 h after treatment. Expressions of aggrecan and Sox9 increased six- and 2.5-fold, respectively at 40 h after treatment with chondrogenic differentiation media. The myogenesis marker Pax7 was up-regulated by two-fold at 40 h after treatment with myogenic differentiation media, and myogenin expression increased about three-fold over untreated cells. MyoD expression had increased about 1.5-fold at 40 h treatment. Protein levels of these cell type-specific markers were then evaluated through immunofluorescence and Western blot. The expression of RUNX2 and OPN increased after osteogenic differentiation medium treatment compared to untreated control. Immunoblot results showed a seven-fold increase in RUNX2 after osteogenic differentiation medium exposure as compared to untreated cells. Protein expression of aggrecan was also increased after exposure to chondrogenic differentiation medium, as evidenced by a six-fold increase in band intensity in differentiation medium-treated cells. Expressions of myogenin and Myf5 also increased after treatment with myogenic differentiation medium as compared to untreated cells, and Western blot analysis of Myf5 revealed a four- to five-fold increase in expression of this protein in myogenic differentiation medium-treated cells (). Protein expression levels of these cell-specific markers indicated successful differentiation of E-iPSC-MSCs into osteogenic, chondrogenic, and myogenic cells.
Figure 5. Confirmation of tri-lineage differentiation of E-iPSC-MSCs.
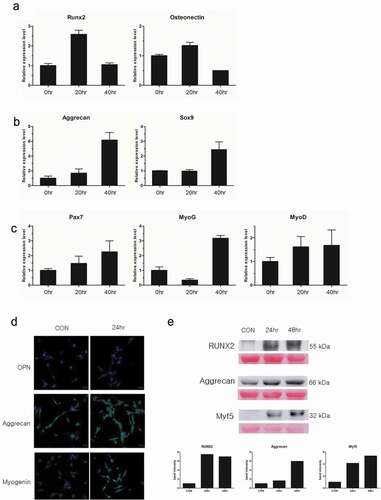
TGF-β2 downregulates MHC class I expression
Mesenchymal stem cells (MSCs) are well suited to clinical use due to their multipotency and immunosuppressive properties, even when used for allogenic cell injection. However, several cases of unfavorable outcomes after allogenic MSC therapy have been reported due to MHC mismatch causing de novo MSCs to produce anti-MHC antibodies. These anti-MHC antibodies exert cytotoxic actions on the injected MSCs, significantly reducing the efficacy of the therapies [Citation23]. We precluded immune rejection after allogenic injection of E-iPSC-MSCs through the application of TGF-β2, which is prominently expressed in immune-privileged tissues. TGF-β2 down-regulates MHC class I levels to prevent autoantigen presentation from inducing an immune response [Citation24]. Concentration and duration of TGF-β2 treatment was optimized by incubating E-iPSC-MSCs with TGF-β2 for different durations and in different amounts, followed by MHC class I expression analysis. TGF-β2 treatment did not alter the morphology of the cells (), and the expression of MHC class I was down-regulated by up to 75% during sustained TGF-β2 treatment as evidenced by mRNA level analysis (). We also assessed the expression of MHC class I in TGF-β2-treated cells at the protein level via immunoblot analysis, and results indicated that TGF-β2-treated E-iPSC-MSCs presented with 50% less expression of MHC class I as treatment progressed ().
Figure 6. Effects of TGF-β2 treatment on E-iPSC-MSCs.
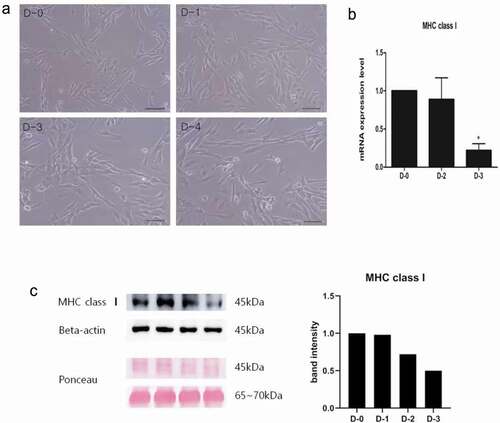
E-iPSC-MSC transplantations showed improvements on musculoskeletal injuries
E-iPSC-MSCs were injected into two normal horses and fourteen injured horses to determine whether our E-iPSC-MSCs were safe for injection. As shown in , E-iPSC-MSCs were transplanted into two healthy horses to verify that they are safe and not causing any adverse effects. E-iPSC-MSC injection into an injured area was generally associated with improvements in the condition of the injured horse. These improvements included reduced lameness, fever, and fracture lines. Although the effects of the injection were usually beneficial, adverse effects after injection were observed in a few animals, such as hot flush and edema. E-iPSC-MSCs were then cultured for two days with TGF-β2, which alleviates immune rejection from mismatched MHC class I antigens of the host (recipient) by downregulating levels of MHC class I, to enhance the stability and safety of these cells. ().
Figure 7. E-iPSC-MSC injection cases.
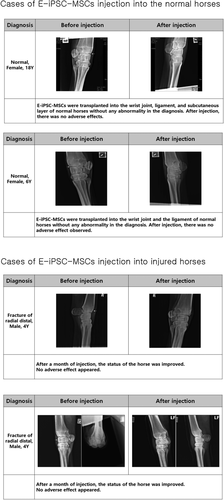
Discussion
Research on horses is an important aspect of veterinary medicine, particularly in the field of sports medicine, and E-iPSCs for potential injury rehabilitation have been established by our group and many other research teams [Citation7,Citation9,Citation16,Citation25,Citation26]. In this study, E-iPSCs were differentiated into MSCs for cell transplantation to treat musculoskeletal injuries in horses (). MSCs are considered a safe cell-based therapy for horses due to their self-renewal and multi-lineage differentiation abilities and their low potential for teratoma formation compared to iPSCs, MSC transplantations, usually harvested from the bone marrow or umbilical cord, have been performed in humans, canines, and many other animals, including horses [Citation27,Citation28]. Until now, among these various cell types, bone marrow-derived mesenchymal stem cells have been the main resource for cell transplantation. However, obtaining BMSCs is comparatively invasive, and their differentiation and proliferation potentials decrease as the age of the donor increases. The need for safer and more efficient cells led us to focus on developing MSCs derived from iPSCs that presented with the same characteristics as other MSCs but maintained their proliferative ability regardless of the age of the donor. Our findings indicate that our E-iPSC-MSCs can be easily obtained and developed and have therapeutic effects.
Figure 8. Establishment of MSC-like cells.
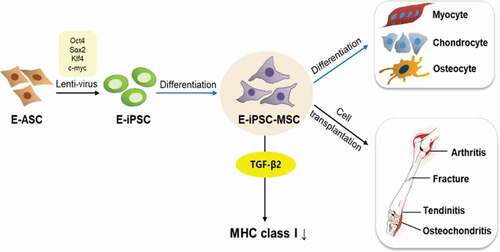
There have been intense efforts by many research groups to establish E-iPSCs using different cell sources, usually fibroblasts, via the implementation of viral vectors [Citation9,Citation25,Citation26,Citation29]. Although others have studied equine iPSC-derived MSCs for implantation in equines for articular defects, tendonitis, and other conditions [Citation30,Citation31], the injection of iPSC-derived MSCs has yet to be reported. Our E-iPSC-MSCs demonstrated higher proliferation rates than conventional E-ASCs, enhancing their potential for use as a source of stem cell therapy. We evaluated the effects of transplantation of E-iPSC-MSCs into spontaneously occurring musculoskeletal injuries in horses. Other stem cell therapies for equine musculoskeletal disorders, such as equine microRNAs secreted from E-iPSCs and E-MSCs, are also under development [Citation27,Citation31–Citation36].
We differentiated our E-iPSCs into MSC-like cells using a specific medium containing supplements, such as glucose, insulin, sodium selenite, transferrin, VEGF, and biotin and employed a serial passaging method[Citation22]. Differentiation of iPSCs into MSC-like cells has been undertaken in human cells [Citation29], with the differentiated cells presenting the following phenotypes: CD29+, CD44+, CD73+, CD90+, CD105+, CD34-, CD31-, and CD45-. Our analyses of equine cells recapitulated these results, suggesting that our E-iPSC-MSCs were successfully differentiated into MSC-like cells. The aim of the current study was to establish MSC-like cells from E-iPSCs for the treatment of musculoskeletal injuries, and as such, the general features of MSCs and their ability to differentiate into other types of cells besides adipocytes, including chondrocytes, osteocytes, or myocytes, were evaluated. Since most musculoskeletal injuries arise in the bone, cartilage, or muscle rather than the fatty part of an organism, we assessed tri-lineage differentiation with E-iPSC-MSCs for generating chondrocytes, osteocytes, and myocytes. Differentiation into each type of cell was induced by culturing in different types of media (). Tests following differentiation confirmed that each cell type was produced, depending on the type of media used.
Although E-iPSCs established by other groups have not yet been applied therapeutically, our E-iPSC-MSCs have been used in clinical therapy and exhibit specific MSC-like functions, such as the capacity for self-renewal and proliferation, as shown in our cell cycle analysis data (). We surmise that our E-iPSC-MSCs might be more effective than bone marrow-derived MSC or other MSCs for clinical purposes. The injection of other types of equine cells cultured for cell therapy, such as E-ASCs, E-PB-MSCs, and equine umbilical cord MSCs did not exhibit a significant effect (data not shown), and E-iPSC-MSCs are currently considered the most effective treatment for musculoskeletal injuries in horses.
According to previous work by Berglund [Citation24,Citation37], allogenic MSCs are a promising source for stem cell therapy, but their use is limited by the risk of immune rejection. After establishing an E-iPSC-derived MSC-like cell line, we conducted tests to confirm whether or not these cells were safe for injection. Cells were assessed for signs of immune rejection after injection into an equine host (). TGF-β2 downregulates MHC class I, and in this study, TGF-β2 treatment resulted in the down-regulation of MHC class I presentation by our MSC-like cells (), which reduced the risk of immune rejection compared to that of allogenic MSC transplantation.
Horse injuries in this study were largely musculoskeletal, involving tendons, bone, cartilage, or ligaments, and occurred naturally. Follow-ups after injection suggested that overall symptoms and conditions were improved by treatment with E-iPSC-MSCs. However, severe adverse effects were observed in a few cases; these included acute pain with edema after the treatment and was perceived as immune rejection. Previous studies have also reported immune rejection after MSC transplantation [Citation37]. We assessed cells after treatment with TGF-β2 treatment and observed reductions in the expression of MHC class I in vitro (), a phenomenon that was recapitulated in in vivo experiments. The overall incidence of adverse effects following E-iPSC-MSC injection after incubation with TGF- β2 was lower than after untreated E-iPSC-MSC injection, with no occurrences of edema or hot flush in the TGF-β2-treated group (). These results indicated that use of TGF-β2 was associated with a reduced occurrence of immune rejection in vivo. However, we recommend further investigation into the clinical use of allogenic E-iPSC-MSCs to explore these findings in greater depth.
In this study, we demonstrated that E-iPSCs could differentiate into injection-safe, MSC-like cells. However, we would like to define the specific molecules (or genes) directly involved in regenerating the injured tissue, and the next step in our research will be to evaluate changes in the expression levels of smaller molecules, such as microRNAs, and other factors to uncover the potential mechanisms that underlie their therapeutic effects.
Our ultimate goal is to develop injection-safe cells for the treatment of musculoskeletal injuries in animals. Our findings establish E-iPSC-MSCs as a promising new therapeutic resource.
Acknowledgments
The research was supported by following grants: the Na Bio-industry Technology Development Program, Korea Institute of Planning and Evaluation for Technology in Food, Agriculture, Forestry and Fisheries (IPET) (312062-5), (815006-3) and the National Research Foundation of Korea (NRF-2017R1EA1A01072781).
Disclosure statement
No potential conflict of interest was reported by the authors.
Additional information
Funding
References
- Ambrosio C, Zomer H, Vidane A, et al. Mesenchymal and induced pluripotent stem cells: general insights and clinical perspectives. Stem Cells Cloning Adv Appl. 2015;8:125.
- Nichols J, Zevnik B, Anastassiadis K, et al. Formation of pluripotent stem cells in the mammalian embryo depends on the POU transcription factor oct4. Cell. 1998;95:379–391.
- Mitsui K, Tokuzawa Y, Itoh H, et al. The homeoprotein nanog is required for maintenance of pluripotency in mouse epiblast and ES cells. Cell. 2003;113:631–642.
- Takahashi K, Yamanaka S. Induction of pluripotent stem cells from mouse embryonic and adult fibroblast cultures by defined factors. Cell. 2006;126:663–676.
- Takahashi K, Tanabe K, Ohnuki M, et al. Induction of pluripotent stem cells from adult human fibroblasts by defined factors. Cell. 2007;131:861–872.
- Lam MT, Longaker MT. Comparison of several attachment methods for human iPS, embryonic and adipose-derived stem cells for tissue engineering. J Tissue Eng Regen Med. 2012;6:s80–s86.
- Nagy K, Sung H-K, Zhang P, et al. Induced pluripotent stem cell lines derived from equine fibroblasts. Stem Cell Rev Rep. 2011;7:693–702.
- Mikkelsen TS, Hanna J, Zhang X, et al. Dissecting direct reprogramming through integrative genomic analysis. Nature. 2008;454:49–56.
- Lee E-M, Kim A-Y, Lee E-J, et al. Generation of equine-induced pluripotent stem cells and analysis of their therapeutic potential for muscle injuries. Cell Transplant. 2016;25:2003–2016.
- Sugii S, Kida Y, Berggren WT, et al. Feeder-dependent and feeder-independent iPS cell derivation from human and mouse adipose stem cells. Nat Protoc. 2011;6:346.
- Sachs PC, Francis MP, Zhao M, et al. Defining essential stem cell characteristics in adipose-derived stromal cells extracted from distinct anatomical sites. Cell Tissue Res. 2012; 505–515. doi:https://doi.org/10.1007/s00441-012-1423-7.
- Du Y, Roh DS, Funderburgh ML, et al. Adipose-derived stem cells differentiate to keratocytes in vitro. Mol Vis. 2010; 16:2680–2689.
- Dey D, Evans GRD. Generation of induced pluripotent stem (iPS) cells by nuclear reprogramming. Stem Cell Int. 2011; 2011:619583.
- Wei X, Yang X, Han Z, et al. Mesenchymal stem cells : a new trend for cell therapy. Nat Publ Gr. 2013;34:747–754.
- Baird A, Lindsay T, Everett A, et al. Osteoblast differentiation of equine induced pluripotent stem cells. Biol Open. 2018;7:bio033514.
- Amilon KR, Cortes-araya Y, Moore B, et al. Generation of functional myocytes from equine induced pluripotent stem cells. Cell Reprogram. 2018;20(5):275–281.
- Patterson-Kane JC, Becker DL, Rich T. The pathogenesis of tendon microdamage in athletes: the horse as a natural model for basic cellular research. J Comp Pathol. 2012;147:227–247.
- DeFronzo RA, Tripathy D. Skeletal muscle insulin resistance is the primary defect in type 2 diabetes. Diabetes Care. 2009;32:S157 LP–S163.
- McCullagh KJA, Perlingeiro RCR. Coaxing stem cells for skeletal muscle repair. Adv Drug Deliv Rev. 2015;84:198–207.
- Burk J, Badylak SF, Kelly J, et al. Equine cellular therapy-from stall to bench to bedside? Cytom Part A. 2013;83(A):103–113.
- Ortved KF, Medicine R. Rehabilitation for tendinous and ligamentous injuries in sport horses. Vet Clin North Am Equine Pract. 2018;34:359–373.
- Lepage SI, Nagy K, Sung H-K, et al. Generation, characterization, and multilineage potency of mesenchymal-like progenitors derived from equine induced pluripotent stem cells. Stem Cells Dev. 2016;25:80–89.
- Watts AE, Yeager AE, Kopyov OV, et al. Fetal derived embryonic-like stem cells improve healing in a large animal flexor tendonitis model. Stem Cell Res Ther. 2011;2(1):4.
- Berglund AK, Fisher MB, Cameron KA, et al. Transforming growth factor-β2 downregulates Major Histocompatibility Complex (MHC) I and MHC II surface expression on equine bone marrow-derived mesenchymal stem cells without altering other phenotypic cell surface markers. Front Vet Sci. 2017;4:84.
- Quattrocelli M, Giacomazzi G, Broeckx S, et al. Equine-induced pluripotent stem cells retain lineage commitment toward myogenic and chondrogenic fates. Stem Cell Reports. 2016;6:55–63.
- Whitworth DJ, Ovchinnikov DA, Sun J, et al. Generation and characterization of leukemia inhibitory factor-dependent equine induced pluripotent stem cells from adult dermal fibroblasts. Stem Cells Dev. 2014;23:1515–1523.
- Carrade DD, Owens SD, Galuppo LD, et al. Clinicopathologic findings following intra-articular injection of autologous and allogeneic placentally derived equine mesenchymal stem cells in horses. Cytotherapy. 2011;13:419–430.
- Kaibuchi N, Iwata T, Onizuka S, et al. Allogeneic multipotent mesenchymal stromal cell sheet transplantation promotes healthy healing of wounds caused by zoledronate and dexamethasone in canine mandibular bones. Regen Ther. 2019;10:77–83.
- Kang R, Zhou Y, Tan S, et al. Mesenchymal stem cells derived from human induced pluripotent stem cells retain adequate osteogenicity and chondrogenicity but less adipogenicity. Stem Cell Res Ther. 2015;6:144.
- Wilke MM, Nydam DV, Nixon AJ. Enhanced early chondrogenesis in articular defects following arthroscopic mesenchymal stem cell implantation in an equine model. J Orthop Res. 2007;7–10. doi:https://doi.org/10.1002/jor.
- Guest DJ, Smith MRW, Allen WR. Equine embryonic stem-like cells and mesenchymal stromal cells have different survival rates and migration patterns following their injection into damaged superficial digital flexor tendon. Equine Vet J. 2010;42:636–642.
- Moro LN, Amin G, Furmento V, et al. MicroRNA characterization in equine induced pluripotent stem cells. PLoS One. 2018;13:1–17.
- Godwin EE, Young NJ, Dudhia J, et al. Implantation of bone marrow-derived mesenchymal stem cells demonstrates improved outcome in horses with overstrain injury of the superficial digital flexor tendon. Equine Vet J. 2012;44:25–32.
- Pacini S, Spinabella S, Trombi L, et al. Suspension of bone marrow–derived undifferentiated mesenchymal stromal cells for repair of superficial digital flexor tendon in race horses. Tissue Eng. 2007;13:2949–2955.
- Guest DJ, Smith MRW, Allen WR. Short communications monitoring the fate of autologous and allogeneic mesenchymal progenitor cells injected into the superficial digital flexor tendon of horses : preliminary study. Equine Vet J. 2008;40:178–181.
- Carrade DD, Affolter VK, Outerbridge CA, et al. Intradermal injections of equine allogeneic umbilical cord-derived mesenchymal stem cells are well tolerated and do not elicit immediate or delayed hypersensitivity reactions. Cytotherapy. 2011;13:1180–1192.
- Berglund AK, Fortier LA, Antczak DF, et al. Immunoprivileged no more: measuring the immunogenicity of allogeneic adult mesenchymal stem cells. Stem Cell Res Ther. 2017;8:288.