ABSTRACT
Accumulating evidence reveals that nuclear phosphoinositides (PIs) serve as central signaling hubs that control a multitude of nuclear processes by regulating the activity of nuclear proteins. In response to cellular stressors, PIs accumulate in the nucleus and multiple PI isomers are synthesized by the actions of PI-metabolizing enzymes, kinases, phosphatases and phospholipases. By directly interacting with effector proteins, phosphoinositide signals transduce changes in cellular functions. Here we describe nuclear phosphoinositide signaling in multiple sub-nuclear compartments and summarize the literature that demonstrates roles for specific kinases, phosphatases, and phospholipases in the orchestration of nuclear phosphoinositide signaling in response to cellular stress. Additionally, we discuss the specific PI-protein complexes through which these lipids execute their functions by regulating the configuration, stability, and transcription activity of their effector proteins. Overall, our review provides a detailed landscape of the current understanding of the nuclear PI-protein interactome and its role in shaping the coordinated response to cellular stress.
Introduction
Phosphoinositides (PIs) are lipid messengers that play essential roles in the regulation of a wide array of cellular processes. The myo-inositol ring in phosphatidylinositol (PtdIns) can be phosphorylated on the 3, 4, and 5 hydroxyls in all possible combinations to generate 7 distinct PIs (). The monophosphorylated PtdIns phosphates (PtdIns3P, PtdIns4P, and PtdIns5P) are the simplest inositol phospholipids. They can be further phosphorylated forming PtdIns(3,4)P2, PtdIns(3,5)P2, PtdIns(4,5)P2, and PtdIns(3,4,5)P3. The PI signaling cycle was discovered by Lowell and Mabel Hokin in the 1950s [Citation1]. As the minor constituents of membranes, PIs are present in the plasma membrane, Golgi, endoplasmic reticulum, endosomes, lysosomes, as well as in the nucleus [Citation2]. Initially, research efforts focused on the biochemical and functional mechanisms of the cytosolic membrane-associated PIs.
Figure 1. Nuclear PI metabolism.
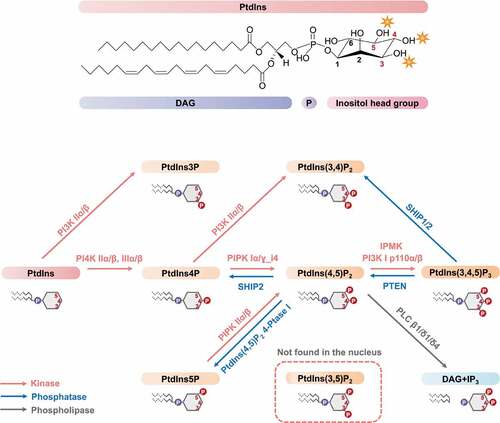
In 1965 and 1977, researchers demonstrated the existence of phospholipids in the nucleus [Citation3,Citation4]. Subsequently in 1983, Smith and Wells detected the activity of phosphatidylinositol kinase and phosphatidylinositol phosphate kinase in the nucleus [Citation5], which inspired the research work of Cocco et al. in 1987 to discover the generation of PIs in the nucleus [Citation6]. We demonstrated that multiple PIs and the kinases that synthesize them are localized to nuclear speckles containing pre-mRNA processing factors that are separate from membranes [Citation7]. These studies expanded the horizon of canonical cytoplasmic PI signaling into the nucleus. In the past 30 years, substantial evidence supports that multiple PIs are present in diverse sub-nuclear compartments along with kinases and phosphatases that generate different nuclear PI pools [Citation8–Citation10]. Additionally, it has become evident that nuclear PI signaling regulates proteins involved in diverse nuclear functions, particularly stress responses. This review will focus on nuclear PIs, their sub-nuclear localization, and their signaling in response to cell stress.
The nuclear PIs and their responses to cellular stress
As the precursor of all PIs (), PtdIns is the most abundant inositol lipid in mammalian cells, accounting for approximately 80% of total cellular PIs [Citation11]. PtdIns4P and PtdIns(4,5)P2 are the most abundant mono- and bi-phosphorylated PIs, which are present at roughly equal abundance in cells (2 ~ 5% of total cellular PIs), constituting approximately 90% of the phosphorylated PtdIns [Citation11–Citation14]. PtdIns3P makes up about 0.2 ~ 0.5% of the total cellular PIs, while PtdIns5P represents approximately 0.01 ~ 0.2% of the total cellular PIs [Citation11,Citation15]. The other 3-phosphorylated PIs, PtdIns(3,4)P2 (<0.1% of total cellular PIs), PtdIns(3,5)P2 (<2% of total cellular PIs), and PtdIns(3,4,5)P3 (<0.05% of total cellular PIs) are typically present at low level or remain undetectable in non-stimulated cells [Citation11,Citation12,Citation14].
Figure 2. Abundance of PIs in eukaryotic cells.
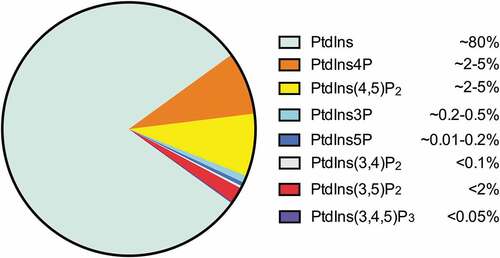
In contrast to the cytosolic PIs which are mainly associated with membranes, the nuclear PIs localize to both membrane and non-membrane structures [Citation16–Citation19] (). To date, all PIs except PtdIns(3,5)P2 have been detected in the nucleus [Citation8,Citation20,Citation21] (). The different compartmentalization of nuclear PIs suggests their broad involvement in multiple signaling pathways.
Table 1. Nuclear targeted PIs: types, locations, and response to cell stress
Figure 3. Sub-nuclear localizations of PIs, kinases, phosphatases, phospholipases and PI-binding proteins.
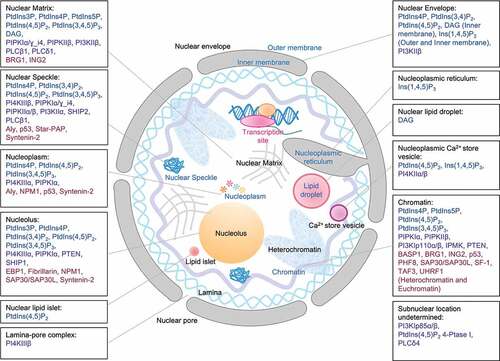
PtdIns3P
PtdIns3P was detected in the dense fibrillar component within nucleoli by PtdIns3P-binding FYVE domains using electron/fluorescent microscopy [Citation22,Citation23]. Newly-synthesized PtdIns3P was found in membrane-depleted nuclei of liver cells post partial hepatectomy, which is proposed to trigger oxidative stress and liver injury [Citation24]. These observations indicate PtdIns3P localization in the nuclear matrix, the structure remaining after harsh nuclear extraction, which includes but is not limited to the nucleoskeleton, and suggest the involvement of PtdIns3P in stress response. Our recent work shows that cisplatin increases the nuclear level of PtdIns3P identified by immunofluorescent staining [Citation25], suggesting a potential role of PtdIns3P in response to genotoxic stress.
PtdIns4P
Newly-synthesized PtdIns4P was detected in the nucleus by incorporating radiolabeled phosphate in purified liver nuclei [Citation24]. The incorporation of 32P into PtdIns4P was also detected in membrane-depleted liver nuclei and after addition of exogenous PtdIns as the substrate [Citation24], suggesting the de novo synthesis of PtdIns4P in the nuclear matrix and the nuclear activation of PtdIns 4-kinases. Using a specific antibody and immunofluorescence, PtdIns4P localized within nuclear speckles, small foci in the nucleoplasm, and nucleoli [Citation23]. Moreover, PtdIns4P was detected at larger foci in the nucleoplasm and nuclear speckles using purified PtdIns4P-binding domain OSH1-PH in fluorescence microscopy [Citation23]. Interestingly, the distribution pattern of PtdIns4P resembles that of nuclear PtdIns(4,5)P2 [Citation23]. Additionally, during the cell cycle, nuclear PtdIns4P levels peak at G1 and then decreases through S phase [Citation26]. The nuclear PtdIns(4,5)P2 turnover also fluctuates through the cell cycle with a peak at the G1/S transition [Citation26]. These studies suggest nuclear PtdIns4P serves as a precursor for PtdIns(4,5)P2.
PtdIns4P also functions at the nuclear envelope, where it serves as the substrate for the perinuclear phospholipase Cε (PLCε) to generate diacylglycerol (DAG), which further activates nuclear protein kinase D (PKD) [Citation27]. This pathway is activated by hypertrophic stimuli and is responsible for the stress-induced pathological hypertrophy [Citation27]. Upon UV irradiation, PtdIns4P detected by SidM-P4M domain rapidly accumulated at DNA damage sites, and the sequestration of PtdIns4P by the SidM-P4M domain partially suppressed Ataxia telangiectasia and Rad3-related protein (ATR)-dependent DNA damage signaling [Citation28], suggesting an association of PtdIns4P with chromatin and a role in DNA damage repair.
PtdIns5P
Nuclear PtdIns5P was first detected in murine erythroleukaemia cells with a 20-fold increase at G1 phase [Citation26]. In 2003, ING2 (inhibitor of growth protein-2) was identified as a PtdIns5P-binding protein through the plant homeodomain (PHD) finger domain in membrane-stripped nuclei, suggesting an association of PtdIns5P-ING2 complex with the nuclear matrix and chromatin [Citation29]. Strikingly, this localization was decreased upon overexpression of PIPKIIβ, which controls the PtdIns5P pool by catalyzing its conversion into PtdIns(4,5)P2 [Citation29]. This finding suggests the complex with PtdIns5P is required for the nuclear retention of ING2. Genotoxic stress (UV irradiation and etoposide) and oxidative stress (H2O2 and L-buthionine[S,R]-sulphoximine (BSO)) specifically induce the accumulation of PtdIns5P in the nucleus [Citation30,Citation31]. In contrast, attenuation of oxidative stress by the antioxidant N-acetyl-cystine (NAC) lowers the level of nuclear PtdIns5P [Citation30]. There is also evidence that activation of the nuclear ubiquitin ligase Cul3-SPOP by the generation of PtdIns5P and its conversion to PtdIns(4,5)P2 by PIPKIIβ [Citation32]. The connection between cellular stressors and nuclear PtdIns5P levels points to multiple roles of nuclear PtdIns5P in stress signaling [Citation33].
PtdIns(3,4)P2
PtdIns(3,4)P2 is actively involved in cellular stress responses. Proliferative stimuli, oxidative stress, and osmotic stress all induce PtdIns(3,4)P2 generation in cells [Citation34]. However, little is known about nuclear PtdIns(3,4)P2 and its role in stress. Incubation of radiolabeled PtdIns(3,4,5)P3 with nuclear extract resulted in the detection of radiolabeled PtdIns(3,4)P2 [Citation35], suggesting de novo synthesis of PtdIns(3,4)P2 and activation of PtdIns 5-phosphatases in the nucleus. Indeed, Src homology 2 domain-containing inositol phosphatase isoform 2 (SHIP2) is the primary enzyme for metabolizing PtdIns(3,4,5)P3 into PtdIns(3,4)P2 in the nucleus [Citation35]. The detection of SHIP2 in nuclear speckles [Citation35] and another PtdIns(3,4,5)P3 5-phosphatase, SHIP1, in nucleoli [Citation36] suggests the localization of PtdIns(3,4)P2 in those sub-nuclear compartments. Using a specific monoclonal antibody, PtdIns(3,4)P2 was detected at the nuclear envelope [Citation37], while its potential intranuclear localization could not be assessed in this study because the nuclear membrane was not permeabilized. Interestingly, H2O2 induced the nuclear surface staining of PtdIns(3,4)P2 [Citation37], suggesting the involvement of PtdIns(3,4)P2 in oxidative stress response.
PtdIns(4,5)P2
Newly-synthesized PtdIns(4,5)P2 was detected in the nucleus by incorporating radiolabeled phosphate in highly purified liver nuclei [Citation24]. The incorporation of 32P into PtdIns(4,5)P2 was also detected in membrane-depleted liver nuclei and after addition of exogenous PtdIns4P as the substrate [Citation24], suggesting the de novo synthesis of PtdIns(4,5)P2 in the nuclear matrix and the nuclear activation of PtdIns4P 5-kinases. Using a specific antibody, PtdIns(4,5)P2 was detected by immunofluorescence at nuclear speckles as well as weak staining in nucleoli [Citation23,Citation38]. Also, a monoclonal antibody detected PtdIns(4,5)P2 at Ins(1,4,5)P3-sensitive nucleoplasmic vesicles, serving as the precursor for Ins(1,4,5)P3 generation, which controls the nuclear Ca2+ pool [Citation39]. Interestingly, while purified PtdIns(4,5)P2-binding domain-Tubby detected PtdIns(4,5)P2 predominantly in the nucleoli, the other purified PtdIns(4,5)P2-binding domain PLCδPH recognized PtdIns(4,5)P2 in both nucleoli and nuclear speckles by fluorescence microscopy [Citation23]. Moreover, using purified PLCδPH followed by immunoelectron microscopy, PtdIns(4,5)P2 labeling was concentrated at heterochromatin with weaker labeling at the nuclear envelope [Citation40]. Additionally, a recent study shows PtdIns(4,5)P2 decorates nuclear lipid islets, where it associates with Pol II transcription machinery, pointing to a potential role in transcriptional regulation [Citation41].
Genotoxic stress, including UV irradiation, the radiomimetic alkylating reagent MMS, and cisplatin increase nuclear PtdIns(4,5)P2 levels [Citation25,Citation28]. Furthermore, the DNA damage agent etoposide and oxidative stress inducer-tert-Butylhydroquinone (tBHQ) specifically promote the generation of PtdIns(4,5)P2 in the nuclear speckles [Citation42,Citation43]. When exposed to UV irradiation, PtdIns(4,5)P2 detected by PLCδPH domain rapidly accumulated at DNA damage sites, and the sequestration of nuclear PtdIns(4,5)P2 by PLCδPH domain suppressed ATR-dependent DNA damage signaling [Citation28]. This is in accord with our recent results that cisplatin treatment induced the local enrichment of PtdIns(4,5)P2 at DNA damage sites [Citation25], suggesting a role of PtdIns(4,5)P2 upon DNA damage stress. In plants, heat shock rapidly induced the generation of PtdIns(4,5)P2 with a specific accumulation at the nuclear envelope and nucleoli [Citation44], suggesting a functional role of PtdIns(4,5)P2 in the heat shock response, which maintains plant homeostasis and facilitates survival during periods of elevated temperatures.
PtdIns(3,4,5)P3
Using the purified PtdIns(3,4,5)P3-binding GST-Grp1-PH domain and electron microscopy, PtdIns(3,4,5)P3 was detected in the nuclear matrix [Citation45]. Consistently, dephosphorylation of [32P]PtdIns(3,4,5)P3 by its 5-phosphatase SHIP2 and 3-phosphatase PTEN (phosphatase and tensin homolog) was found in membrane-depleted nuclei [Citation35], supporting PtdIns(3,4,5)P3 localization in the nuclear matrix. Additionally, SHIP2 colocalized with the SC35 splicing factor [Citation35], suggesting the presence of PtdIns(3,4,5)P3 in nuclear speckles. Moreover, PtdIns(3,4,5)P3 was detected in the nucleoplasm and nucleoli by immunofluorescence [Citation46].
Newly-synthesized PtdIns(3,4,5)P3 was detected in the nucleus by incorporating radiolabeled phosphate in highly purified liver nuclei [Citation24]. Interestingly, when nuclei were depleted of their membrane, and after addition of exogenous PtdIns(4,5)P2 as the substrate, no radiolabeling of PtdIns(3,4,5)P3 was detected [Citation24], suggesting either the nuclear PtdIns(4,5)P2-3 kinases are membrane-associated or they function only on anchored substrates.
Genotoxic stress by cisplatin [Citation25] and proliferative stimulation by platelet-derived growth factor (PDGF) [Citation45] have been reported to increase nuclear PtdIns(3,4,5)P3. Upon UV irradiation, PtdIns(3,4,5)P3 detected by Btk-PH domain was rapidly accumulated at DNA damage sites, and the sequestration of nuclear PtdIns(3,4,5)P3 by Btk-PH domain suppressed ATR-dependent DNA damage signaling [Citation28], suggesting an association of PtdIns(3,4,5)P3 with chromatin and a role in DNA damage repair. Other cellular stimuli such as oxidative stress (H2O2) and proliferative stimuli (nerve growth factor (NGF) and PDGF) have also been reported to induce the generation of PtdIns(3,4,5)P3 in the nucleus [Citation47].
DAG and Ins(1,4,5)P3
Hydrolysis of PtdIns(4,5)P2 by PI-PLC (PI-specific phospholipase C) leads to the generation of diacylglycerol (DAG) and Ins(1,4,5)P3, and subsequent activation of Ins(1,4,5)P3 receptors for Ca2+ release and the DAG-mediated protein kinase C (PKC) pathway [Citation48,Citation49]. DAG localizes with the nuclear envelope [Citation27] (especially at the inner membrane [Citation50]), nuclear lipid droplets [Citation50], and the nuclear matrix [Citation51], whereas Ins(1,4,5)P3 receptors localize with the nuclear envelope [Citation52], including both outer and inner membrane [Citation52,Citation53], nucleoplasmic reticulum [Citation54], and nucleoplasmic Ca2+ store vesicles [Citation39], mostly governing the intranuclear calcium signaling for cell survival. The presence of PLCβ1 at nuclear speckles implicates that its activity generates DAG and Ins(1,4,5)P3 at these sites [Citation55]. The nuclear DAG level increases in response to proliferative signals such as insulin-like growth factor-I (IGF-I) and differentiation stimuli such as dimethyl sulfoxide [Citation56]. The nuclear DAG and Ins(1,4,5)P3 are actively involved in Endothelin-1 (ET1)-induced hypertrophic stress as ET-1 elevates nuclear DAG levels [Citation57] and induces the synthesis of DAG by PLCε-dependent hydrolysis of PtdIns4P at the nuclear envelope [Citation27]. Moreover, the induction of nuclear Ins(1,4,5)P3 mediates ET1-induced hypertrophy for pathological cardiomyocyte growth [Citation58].
The nuclear PI kinases, phosphatases, and phospholipases
Nuclear PI metabolism is tightly regulated by multiple kinases, phosphatases, and phospholipases that allow dynamic lipid turnover that is temporally and spatially regulated. The different compartmentalized enzymes actively participate in nuclear signaling and stress response ( and ).
Table 2. Nuclear PI-metabolizing enzymes, their substrates, nuclear location and response to cell stress
PtdIns 4-kinases
PtdIns4P is primarily produced by the action of type II (α and β) and III (α and β) PtdIns 4-kinases [Citation59]. The missing type I was a historical artifact which turned out to be PtdIns 3-kinase [Citation60]. Although PtdIns 4-kinase activity has been detected in the nucleus [Citation24], very little is known about the nuclear PtdIns 4-kinases. Using immunofluorescence, PI4KIIIα was detected in the nucleoplasm and nucleoli, whereas PI4KIIα displayed moderate cytoplasmic localization and more intense nuclear staining [Citation61]. PI4KIIα and PI4KIIβ have been detected on nucleoplasmic Ca2+ store vesicles, where they produce PtdIns4P as the precursor for PtdIns(4,5)P2 and downstream Ins(1,4,5)P3 generation for the control of nucleoplasmic Ca2+ concentration [Citation39]. Through subcellular fractionation, PI4KIIIβ was detected at the nuclear lamina-pore complexes, and the inhibition of nuclear export by leptomycin B resulted in its accumulation in the nucleus [Citation62]. Consistently, the association of specifically-phosphorylated forms of PI4KIIIβ (Ser-496/Thr-504) with nuclear speckles also supports the nuclear localization of PI4KIIIβ [Citation63]. All PtdIns 4-kinases contain a nuclear localization signal (NLS) and nuclear export signal (NES) [Citation64], suggesting their involvement in nucleo-cytoplasmic traffic. Although the functional role of nuclear PtdIns 4-kinases remain unclear, the involvement of nuclear PtdIns4P in stress responses suggests a role in nuclear signaling.
PIP kinases
Both type I and II PIP kinases contain α, β, and γ isoforms. While type I PIP kinases primarily catalyze the conversion of PtdIns4P into PtdIns(4,5)P2, type II PIP kinases predominantly catalyze synthesis of PtdIns(4,5)P2 from PtdIns5P. To date, PIPKIα, PIPKIγ_i4, PIPKIIα, and PIPKIIβ have all been found in the nucleus in association with nuclear matrix [Citation7,Citation8,Citation25,Citation43,Citation65]. PIPKIα and PIPKIIα localize to nuclear speckles, where they colocalize with PtdIns(4,5)P2 and mRNA-processing machinery [Citation7]. Moreover, immunofluorescence shows the enrichment of PIPKIα in nucleoli at G1/S phase of the cell cycle [Citation66]. PIPKIα contains a nuclear speckle-targeting region (440–562) and accumulates in nuclear speckles upon oxidative stress where it associates with the poly (A) polymerase Star-PAP, controlling 3ʹ-end processing of select stress-induced mRNAs [Citation43]. In response to genotoxic stress, PIPKIα localizes to sites of DNA damage, suggesting a role in DNA damage repair consistent with an accumulation of phosphoinositides at these sites [Citation25,Citation28]. PIPKIγ generates PtdIns(4,5)P2 in focal adhesion sites and adherens junctions [Citation65]. There are at least four PIPKIγ splicings in humans, i1, i2, i4, and i5 [Citation65]. Among them, PIPKIγ_i4 is found in both the cytosol and the nucleus, and in nuclei colocalizes with speckle markers [Citation65]. PIPKIIβ is enriched in nuclear speckles [Citation7,Citation32,Citation67,Citation68]. Three-dimensional structural analyses and point mutations indicate that α-helix 7 and insert region of PIPKIIβ is essential for its nuclear localization and its interaction with the speckle-type POZ domain protein (SPOP) a component of Cul3-based ubiquitin ligases [Citation32,Citation67]. Upon treatment with the anti-cancer vitamin D metabolite-1α,25(OH)2D3, nuclear PIPKIIβ is activated and generates PtdIns(4,5)P2, leading to E-cadherin upregulation and inhibition of cell motility [Citation69]. PIPKIIβ functions in the PtdIns5P pathway [Citation32], PtdIns5P responds to the genotoxic agent etoposide and regulates the expression of myogenic genes during myoblast differentiation [Citation70].
PtdIns 3-kinases
PtdIns 3-kinases (PI3Ks) are divided into three classes. Class I PI3Ks are the dominant kinases that synthesize PtdIns(3,4,5)P3, and class III PI3Ks are responsible for synthesizing PtdIns3P. Class II PI3Ks synthesize both PtdIns3P and PtdIns(3,4)P2 to a lesser extent [Citation71].
Class I PI3Ks functions as heterodimers consisting of a regulatory subunit (p85⍺ or its two splicing variants (p55⍺ and p50⍺), p85β, p55γ, p101 or p84/p87) and a catalytic subunit (p110⍺, p110β, p110δ or p110γ). The class I PI3Ks are involved in nuclear signaling and stress response. Upon H2O2-induced oxidative stress, the nuclear fraction of p85⍺ is markedly increased with considerable tyrosine phosphorylation [Citation47]. p85β contains an NES whereas p110β contains an NLS in its C2 domain [Citation72]. Together these signals regulate the nuclear localization of p85β/p110β and control cell survival [Citation72,Citation73]. While p110β is mainly nuclear in starved cells, proliferative stimuli (NGF, serum, and PDGF) increase its association with chromatin [Citation72]. Consistently, both p110⍺ and p110β are associated with Akt and proliferating cell nuclear antigen (PCNA) in the nucleus, with p110β being the dominant isoform that regulates PCNA loading onto chromatin for DNA replication and cell proliferation [Citation74]. Moreover, p110β and its product-PtdIns(3,4,5)P3 accumulate at sites of DNA damage following UV irradiation, where p110β senses double-strand breaks (DSB) and recruits the Nbs1 sensor protein to damaged DNA [Citation73]. Inhibiting the kinase activity of p110β inhibits DNA damage repair [Citation73], indicating a functional role of PtdIns(3,4,5)P3 in genotoxic stress signaling.
The C2 domain of the class II PI3K-CIIα also contains an NLS, which is essential for its nuclear localization [Citation75]. Indeed, PI3K-CIIα concentrates in nuclear speckles and colocalizes with snRNA [Citation75]. Treating cells with transcription inhibitors α-amanitin and actinomycin D results in the phosphorylation of PI3K-CIIα [Citation75]. Similarly, the C2 domain of PI3K-CIIβ also contains an NLS [Citation76]. PI3K-CIIβ co-localizes with lamin A/C in the nuclear matrix and is activated in membrane-depleted nuclei [Citation24,Citation76]. PI3K-CIIβ is also found in the nuclear envelope where it exhibits robust activity [Citation75]. Stimulation with epidermal growth factor augments PI3K-CIIβ expression and kinase activity in the nucleus [Citation76]. Post partial hepatectomy, PI3K-CIIβ activation occurs in membrane-depleted nuclei, leading to PtdIns3P production during compensatory liver growth [Citation24], suggesting a role of PI3K-CIIβ in stress responses.
IPMK
Inositol polyphosphate multikinase (IPMK) functions as PI 3-kinase in the nucleus, converting PtdIns(4,5)P2 to PtdIns(3,4,5)P3 [Citation77,Citation78]. Overexpressed IPMK colocalizes with and increases nuclear PtdIns(3,4,5)P3 [Citation77]. IPMK converts nuclear receptor Steroidogenic Factor-1 (SF-1)-bound PtdIns(4,5)P2 into PtdIns(3,4,5)P3, and knockdown of IPMK results in decreased abundance of SF-1 targets [Citation79]. UV irradiation recruits IPMK to DNA damage sites, where it produces PtdIns(3,4,5)P3 and contributes to the ATR DNA damage repair machinery [Citation28]. The chemotherapy drug etoposide recruits IPMK to promoters of p53 target genes, PUMA (p53 up-regulated modulator of apoptosis), Bax (Bcl-2 associated X) and p21, where it interacts with p53 and regulates transcriptional control of select p53 targets [Citation80].
PTEN
PTEN is a phosphatase and key tumor suppressor [Citation81]. It preferentially dephosphorylates the D-3 position of PtdIns(3,4,5)P3 and PtdIns(3,4)P2, antagonizing PI3K signaling [Citation35]. PTEN is present in the nucleus, where its phosphatase activity was detected [Citation35]. Studies have demonstrated the presence of PTEN in nucleoli and chromatin by immunofluorescence, where depletion of PTEN induced nucleolar morphologic changes and spontaneous DSBs [Citation82,Citation83]. Both genotoxic and oxidative stress promote the chromatin association of PTEN in an inverse correlation with the amount of cytosolic PTEN [Citation84], suggesting nuclear import of PTEN in response to cellular stress. Significantly, PTEN interacts with Rad52, a key regulator of DNA DSB repair, at DNA damage sites [Citation84], suggesting its role in DNA damage repair. PTEN also interacts with and regulates p53 [Citation85–Citation90].
SHIP1 and SHIP2
SHIP1 and SHIP2 catalyze the dephosphorylation of PtdIns(3,4,5)P3 at the D-5 position in the nucleus [Citation35,Citation36]. SHIP1 is present in nucleoli, and its expression is induced in response to the proteasome inhibitor MG132 [Citation36]. Detailed studies show that 8 hours of MG132 treatment induced SHIP1-p53 colocalization in the nucleolar cavity; however, after 16 hours of treatment, SHIP1 and p53 start to localize to different regions in the nucleoli [Citation36], suggesting spatial and temporal roles of SHIP1 during stress responses. Immunofluorescence reveals the localization of SHIP2 in nuclear speckles [Citation35]. The transcriptional inhibitor ⍺-amanitin, causes SHIP2 to redistribute together with the speckle component SC35 into fewer and larger structures [Citation35]. Moreover, phospho-SHIP2 (Ser132) co-localizes with PtdIns(4,5)P2 in nuclear speckles, which demonstrate PtdIns(4,5)P2 phosphatase activity [Citation91]. As PtdIns(4,5)P2 responds to genotoxic and oxidative stresses in nuclear speckles [Citation42,Citation43], SHIP2 may play a role in nuclear stress signaling.
Type I PtdIns(4,5)P2 4-phosphatase
PtdIns(4,5)P2 4-phosphatases, including type I and II, dephosphorylate PtdIns(4,5)P2 to produce PtdIns5P. In response to genotoxic stress induced by etoposide or doxorubicin, type I PtdIns(4,5)P2 4-phosphatase translocates to the nucleus and augments PtdIns5P levels [Citation92]. The elevated nuclear PtdIns5P activates the ING2-p53 apoptotic pathway by facilitating ING2-dependent p53 acetylation and stabilization [Citation92]. Consistently, the pro-apoptotic effect of p53 can be inhibited by PIPKIIβ, an enzyme that converts PtdIns5P into PtdIns(4,5)P2 [Citation92], indicating a functionally important role of PtdIns5P, type I PtdIns(4,5)P2 4-phosphatase and PIPKIIβ in p53-dependent stress responses.
PLC family
PI-PLC is a family of 13 isozymes classified into 6 isotypes (β, γ, δ, ε, ζ, η) that participate in PtdIns(4,5)P2 catabolism, producing Ins(1,4,5)P3 and DAG, mobilizing cellular calcium and activating PKC. All four PLCβ isozymes have been detected in the nucleus, especially in the nuclear matrix when membranes are depleted, with PLCβ1 the most abundant nuclear isoform [Citation93]. IGF1 selectively activates PLCβ1 in the nucleus without affecting its localization [Citation56]. PLCβ1 is also present in nuclear speckles [Citation55]. Indeed, overexpression of PLCβ1 protects cells from oxidative stress by facilitating the mRNA generation of c-Fos for cell survival [Citation94]. PLCδ1 is found in the nuclear matrix and partially colocalizes with PtdIns(4,5)P2 in DAPI-low areas [Citation95]. Upon cell cycle arrest by serum starvation or double thymidine blockade, PLCδ1 accumulates in the nucleus at the G1/S transition by a PtdIns(4,5)P2-dependent mechanism, as mutation of the PtdIns(4,5)P2-binding site reduced the nuclear localization of PLCδ1 [Citation95]. Nuclear-localized PLCδ4, when knocked down, is reported to cause cell cycle arrest, with an increased G1 phase and decreased S and G2/M phases [Citation96].
The nuclear PI-binding proteins
The nuclear PIs are signaling messengers that function in precise locations with specific PI-binding proteins. This is achieved by specific PI-binding domains/motifs in proteins that anchor to or constantly sample the environmental phospholipids. These PI-protein complexes are controlled both at temporal and spatial levels by various mechanisms, and they are actively involved in nuclear signaling and stress responses ( and ).
Table 3. Nuclear PI-binding proteins, their interacting PIs, associated PI enzymes, nuclear location, response to cell stress and functions
Aly
Aly, a nuclear export factor, is involved in the assembly and recognition of spliced messenger RNA with TREX [Citation97]. The function of Aly on mRNA export depends on nuclear speckle residency, which is directly impacted by binding to PtdIns(4,5)P2 and PtdIns(3,4,5)P3 through its N-terminal RG motif, as mutants lacking PtdIns(4,5)P2/PtdIns(3,4,5)P3-binding accumulate in the nucleoplasm [Citation98]. Conversion of Aly-bound PtdIns(4,5)P2 into PtdIns(3,4,5)P3 by IPMK, and phosphorylation on T219 by Akt1 are further required for specific mRNA motif recognition [Citation98,Citation99]. The Aly-PIs complex hasn’t been explored in the context of cell stress, but multiple stressors increase nuclear PtdIns(4,5)P2/PtdIns(3,4,5)P3 levels [Citation25,Citation28,Citation45,Citation47], with some specifically causing PtdIns(4,5)P2 accumulation in nuclear speckles [Citation42,Citation43], suggesting stress roles at play for Aly-regulated transcripts.
BASP1
The brain acid-soluble protein 1 (BASP1) is a transcriptional repressor regulating Wilms’ tumor 1 protein (WT1) target genes. The myristoylated BASP1 binds to nuclear PtdIns(4,5)P2 through its N-terminal polybasic region (PBR), which leads to the recruitment of PtdIns(4,5)P2 to the promoter region of WT1 target genes, where they recruit Histone Deacetylase 1 (HDAC1) and elicit transcriptional repression [Citation100]. As an oncogene, WT1 has been reported to enhance cisplatin-resistance of advanced non-small-cell lung cancer (NSCLC), suggesting a role of BASP1-PtdIns(4,5)P2 complex in genotoxic stress response [Citation101]. BASP1 also protects cells from cellular stress induced by abrin toxin, a type II ribosome-inactivating protein, by sequestering the abrin A chain to the nucleus to alleviate its inhibitory effect on protein synthesis [Citation102].
BRG1
The Brahma Related Gene 1 (BRG1) protein is an ATP-dependent helicase subunit of the BAF chromatin remodeling complex that controls gene expression [Citation103]. It has roles in DNA damage repair, oxidative stress, and metabolic stress [Citation104–Citation106]. BRG1 directly binds to PtdIns(4,5)P2, and this binding facilitates its association with nuclear β-actin, which in turn enhances the ATPase activity of BRG1 [Citation107]. The BRG1-PtdIns(4,5)P2 complex also stabilizes nuclear β-actin. Interestingly, four regions of BRG1 bind to β-actin, and PtdIns(4,5)P2 replaces two of them while leaving the others still available for binding [Citation108], suggesting that PtdIns(4,5)P2-binding exposes/uncaps β-actin so β-actin can interact with actin filaments or other proteins. BRG1 may utilize β-actin polymerization and interactions to assist in chromosomal remodeling, allowing for the repression or expression of target genes. As BRG1 regulates expression of stress response genes [Citation106], PtdIns(4,5)P2 may play a role in transcriptional regulation of these genes.
EBP1
ErbB3-binding protein (EBP1), a putative tumor suppressor, translocates to the nucleus and functions as a transcriptional corepressor. It directly binds to several PIs via two lysine-rich PBR domains at the N- and C-terminus of the protein [Citation46]. The C-terminal PBR mediates the nucleolar localization of EBP1, where it associates with PtdIns(3,4,5)P3 via both electrostatic and hydrophobic interactions and co-localizes with the PI3K catalytic subunit p110β [Citation46]. Interestingly, genotoxic stress triggers SUMOylation of EBP1, facilitating its nucleolar localization, protein stability, and transcriptional repressive activity, suggesting a potential role of the EBP1-PtdIns(3,4,5)P3 complex in stress response.
Fibrillarin
Fibrillarin is a small nucleolar ribonucleoprotein (snoRNP) involved in pre-ribosome assembly and rRNA modification [Citation109,Citation110]. It also functions in the innate immune response as it is downregulated upon bacterial infection, which causes enhanced cell survival, inflammation reduction, and bacteria clearance [Citation111]. Fibrillarin directly binds to PtdIns(4,5)P2, and together they form a complex that co-localizes with nascent rRNA dependent on rRNA transcription [Citation110,Citation112]. Inhibition of rRNA transcription during mitosis or via chemical inhibition (actinomycin D), disrupts the co-localization of fibrillarin with PtdIns(4,5)P2 and rRNA synthesis foci [Citation110,Citation112,Citation113]. Fibrillarin also loses its localization to nucleoli in response to diverse cell stressors such as heat shock, bacterial infection, or various drugs (actinomycin D, 5,6-Dichloro-1-β-D-ribofuranosylbenzimidazole (DRB), cycloheximide, and Roscovitine/Seliciclib) [Citation111,Citation113]. The exact role of fibrillarin-PtdIns(4,5)P2 binding remains unclear, but data does suggest that PtdIns(4,5)P2 binding induces a conformational change in fibrillarin [Citation102], which could stabilize it as in the case of p53 [Citation25], or aid in its localization as in the case of Aly [Citation98].
ING2
ING2 is a tumor suppressor that forms a chromatin-modifying complex for modulating methylation and acetylation of lysine residues [Citation31]. It contains a PHD finger domain that coordinates with zinc to bind PtdIns5P [Citation29]. Upon DNA damage, ING2 localizes to chromatin, mediates p53 acetylation, and represses a subset of specific target genes during the DNA damage response [Citation31,Citation92]. ING2 localization with chromatin, p53 acetylation, and ING targeted gene repression are all dependent on Ptdins5P binding [Citation29,Citation31]. Loss of ING2 binding to Ptdins5P reduces p53-mediated apoptosis in response to etoposide or H2O2 [Citation29]. This is consistent with studies that show ING2 upregulation and increased nuclear localization of Type I PtdIns(4,5)P2 4-phosphatase, the main PtdIns5P generating enzyme, upon DNA damage [Citation31,Citation92,Citation114]. The generation of PtdIns5P likely occurs at specific target sites on chromatin to recruit and activate ING2 [Citation29]. Taken together, these studies show that PtdIns5P plays a key role in localizing ING2 to chromatin and activating it in response to genotoxic and oxidative stress.
NPM1
NPM1 (also known as B23 or nucleophosmin) is a multifunctional chaperone implicated in ribosome biogenesis, chromatin remodeling, and DNA damage repair, and is frequently overexpressed in proliferating cells and interacts with both p53 and the retinoblastoma (Rb) tumor suppressor gene product [Citation115–Citation117]. NPM1 is predominantly located in nucleoli, but a smaller fraction also can be detected in the nucleoplasm [Citation115]. With both NLS and NES, NPM1 shuttles between the nucleus and cytoplasm and facilitates the nucleolar targeting of proteins [Citation115,Citation118]. Genotoxic stress and viral stress (viral K cyclin) induce the re-localization of NPM1 from nucleoli to the nucleoplasm, where it binds HDM2 and inhibits p53 degradation by disrupting HDM2-p53 complex formation [Citation117]. NPM1 was also identified in a complex with PtdIns(3,4,5)P3 that prevents cells from undergoing apoptosis [Citation119]. This effect was abrogated by hydrolyzing PtdIns(3,4,5)P3 with PTEN or SHIP [Citation119]. Interestingly, a mutant NPM1 lacking the p53-interacting domain fails to confer cellular resistance to stress-induced apoptosis [Citation116], suggesting that NPM1 protects cells from apoptosis through a mechanism involving p53, potentially in a PtdIns(3,4,5)P3-dependent manner. Recent studies show that NPM1 undergoes liquid-liquid phase separation (LLPS), a process important for defining the membrane-less compartmentalization in nucleoli [Citation120,Citation121]. In the future, it would be of interest to test the role of lipid messengers in LLPS, especially under stressed conditions.
PHF8
The histone lysine demethylase PHF8 (PHD finger protein 8) directly interacts with PtdIns(4,5)P2 through the C-terminal lysine/arginine-rich motif [Citation122]. This interaction represses demethylation of H3K9me2 at the rDNA promoter, which represses the transcription of rRNA genes [Citation122]. Genotoxic stress induced by UV irradiation and the radiomimetic DNA damage agent neocarzinostatin (NCS) increases protein levels of PHF8 via USP7 deubiquitinase-dependent stabilization, leading to the upregulation of a group of genes, including cyclin A2, which is critical for cell growth and proliferation in breast cancer [Citation123]. Interesting, the C-terminal region, where PtdIns(4,5)P2 binds, is also required for USP7 binding and transcriptional regulation of the DNA damage response [Citation123], suggesting a potential regulatory role of the PHF8-PtdIns(4,5)P2 complex in the cellular stress response.
SAP30 and SAP30L
The Sin3A-associated proteins SAP30 and SAP30L are linker molecules between various components of the Sin3A corepressor complex that regulates histone deacetylation and transcriptional activity. SAP30 and SAP30L not only facilitate the nucleolar targeting of Sin3A, but also directly associate with core histones and naked DNA [Citation124,Citation125]. SAP30 and SAP30L interact specifically with monophosphoinositides, most tightly to PtdIns5P (followed by PtdIns3P and PtdIns4P) through a PBR and supported by a hydrophobic region and a zinc-coordination structure [Citation124]. Interestingly, the same region also contributes to DNA binding. Mutation and deletion of this region blocked the chromatin association of SAP30L, and decreased the repressive activity of SAP30L. Oxidative stress, which induces nuclear PtdIns5P accumulation [Citation30], led to relocalization of SAP30L from the nuclear chromatin to the cytoplasm and attenuated its repressive activity [Citation124]. Potentially, the SAP30L-chromatin interaction is mediated by the PI-/DNA-binding region, and PI binding disrupts this, leading to decreased transcriptional repression by SAP30L.
SF-1
The nuclear receptor SF-1 is an orphan nuclear receptor that serves as an essential regulator of multiple hormone-induced genes in the vertebrate endocrine system. It binds PIs and other phospholipids in a large hydrophobic pocket [Citation119,Citation126]. This pocket sequesters the hydrophobic tails of PtdIns(4,5)P2/PtdIns(3,4,5)P3 with their head groups fully solvent-exposed, which may define a new interaction surface between SF-1 and coregulatory proteins [Citation127]. Indeed, PtdIns(3,4,5)P3 binding increases coactivator recruitment to SF-1 and increases SF-1 activity [Citation127]. Nuclear IPMK and PTEN switch SF-1-bound PIs between PtdIns(4,5)P2 and PtdIns(3,4,5)P3, regulating SF-1 transcriptional activity [Citation79]. UV irradiation-induces SF-1 accumulation at DNA damage sites, where it forms a complex with IPMK-catalyzed PtdIns(3,4,5)P3 and contributes to the ATR DNA damage repair machinery [Citation28]. Analysis of a patient heterozygous for the SF-1 gene indicated that reduced expression of SF-1 leads to adrenal failure [Citation128]. In SF-1 heterozygous mice, adrenal deficits were revealed upon stress induction, including food deprivation, and inflammation induced by injection of lipopolysaccharide [Citation128], suggesting potential roles of SF-1 in stress responses, which is possibly PI-regulated.
Syntenin-2
Syntenin-2 binds with high affinity to PtdIns(4,5)P2 through its two PDZ (Postsynaptic density protein/Disc large/Zona occludens) domains [Citation129]. These domains target syntenin-2 to nuclear speckles and nucleoli. Although the response of syntenin-2 to cell stress is unknown, it likely plays a role in stress signaling as depletion of syntenin-2 disrupts PtdIns(4,5)P2 localization at nuclear speckles [Citation129], where the PtdIns(4,5)P2 generation is induced by genotoxic and oxidative stress [Citation42,Citation43], suggesting that syntenin-2 may function as an organizer of nuclear PtdIns(4,5)P2. Moreover, depletion of syntenin-2 decreased cell division and survival [Citation129], indicating that the syntenin-2-PtdIns(4,5)P2 complex may play important roles in cell viability and proliferation.
TAF3
The highly conserved RNA polymerase II transcription factor (TFIID) comprises the TATA box-binding protein (TBP) and a set of TBP-associated factors (TAFs, including TAF3) that play key roles in promoter recognition. Through a targeted screen for PI interactors, TAF3 was found to strongly interact with PtdIns5P through its PHD finger [Citation70]. This interaction modulates the association of TAF3 with chromatin and regulates TAF3-dependent gene expression during myoblast differentiation. Etoposide-induced genotoxic stress, which largely increases nuclear PtdIns5P [Citation31], enhances the TAF3-PtdIns5P interaction and alters transcriptional output to promote cell differentiation [Citation70]. Notably, PIPKIIβ, the kinase that converts PtdIns5P to PtdIns(4,5)P2, also regulates specific gene expression dependent on the PI-binding site of TAF3 upon etoposide treatment [Citation70], suggesting that TAF3-mediated nuclear alterations contribute to its transcriptional regulation.
UHRF1
Ubiquitin-like with PHD and RING finger domain 1 (UHRF1) is a nuclear multi-domain protein, localized to chromatin that plays key roles in DNA methylation, histone H3 modification (H3K9me3), and gene regulation, including silencing tumor suppressors [Citation130–Citation132]. UHRF1 is allosterically regulated by PtdIns5P binding [Citation133]. Without PtdIns5P, the PBR in the C terminus of UHRF1 occupies an essential peptide-binding groove in its tandem tudor domain (TTD) to block its interaction with H3K9me. In this state, UHRF preferentially interacts with unmodified H3. In contrast, binding of PtdIns5P to the PBR of UHRF1 leads to a conformational change that releases the TTD for H3K3me binding, suggesting that PtdIns5P or other nuclear PIs may directly impact gene expression by regulating the chromatin binding activity of interactors. Upon psoralen/cisplatin-induced DNA damage, UHRF1 was enriched at the DNA lesions, serving as a lesion recognition factor and nuclease scaffold for DNA damage repair [Citation134]. Depletion of UHRF1 increases DNA damage sensitivity [Citation134]. Also, UHRF1 protects cancer cells against diethyl maleate (DEM)-induced oxidative stress by activating the oncogenic Keap1-Nrf2 pathway [Citation135]. Given that UHRF1 is over-expressed in many cancer cells [Citation135–Citation137], the UHRF1-PtdIns5P complex may contribute to the malignant behavior of cancer cells and mediate chemotherapy-resistance.
Star-PAP
Speckle targeted PIPKIα regulated-poly(A) polymerase (Star-PAP) [Citation43] (also called TUT1) [Citation138] is a noncanonical poly(A) polymerase localized in nuclear speckles [Citation43]. It controls the expression of about 40% of human genes and is activated by DNA damage and oxidative stress signals [Citation42,Citation43,Citation139]. Star-PAP processes mRNAs by directly binding to the 3ʹUTR upstream of the poly(A) signal, where it recruits the cleavage and polyadenylation specificity factor (CPSF) subunits [Citation140]. In nuclear speckles, especially in response to oxidative stress (tBHQ), Star-PAP complexes with PIPKIα and directly binds PtdIns(4,5)P2, which enhances the polyadenylation activity of Star-PAP to control the expression of selected genes [Citation43]. Oxidative stress also stimulates CKIα/ε-mediated Star-PAP phosphorylation, which is critical for Star-PAP activity and its stimulation by PtdIns(4,5)P2 [Citation141]. Moreover, etoposide-induced genotoxic stress stimulates the nuclear localization of PKCδ, which is integrated into the Star-PAP complex and directly interacts with PIPKIα [Citation42]. PIPKIα-produced PtdIns(4,5)P2 further activates PKCδ, which in turn promotes the activity of Star-PAP for 3ʹ end processing of BIK mRNA and induction of apoptosis [Citation42]. In contrast, activation of the Star-PAP pathway by oxidative stress activates a distinct subset of genes, suggesting specificity of Star-PAP in stress signaling. Other Star-PAP target genes are independent of PIPKIα and may be independent of PI signaling [Citation139].
p53
The tumor suppressor p53 is activated by a wide range of cellular stressors, protecting the genome from genotoxic stress, oxidative stress, hypoxia, and oncogene activation [Citation142–Citation144]. Most cellular stressors induce accumulation of wild-type p53 in the nucleus for the transcriptional regulation of gene networks that lead to cell cycle arrest, DNA damage repair, senescence, apoptosis, and/or metabolic reprogramming [Citation143]. Mutant p53 dramatically accumulates in the nucleus and stress further enhances this accumulation [Citation25]. Significantly, a subset of mutant p53 proteins acquire oncogenic activities [Citation145,Citation146]. We have demonstrated that p53 is a novel PI-binding protein, and the stability of mutant p53 and stress-induced wild-type p53 is regulated by PtdIns(4,5)P2 [Citation25]. Under genotoxic or oxidative stress, nuclear PIPKIα binds to p53, leading to the production and association of PtdIns(4,5)P2 with the C-terminal PBR of p53 [Citation25], and this sequence is known to regulate many p53 functions [Citation25,Citation143,Citation144,Citation146]. PtdIns(4,5)P2-binding recruits small heat shock proteins HSP27/HSPB1 and αB-Crystallin/HSPB5 to the p53 complex, which stabilizes p53 and displaces PIPKIα from the complex [Citation25]. Using sub-nuclear makers and proximity ligation assay (PLA), an approach to detect in situ interaction of two epitopes within 40 nm [Citation147–Citation150], the PI-p53 complex was identified in the nucleoplasm, nuclear speckles, and DNA damage sites, indicating multiple roles for this complex. Mutant p53, PIPKIα, and sHSPs have independently been implicated in cancer progression [Citation146,Citation151–Citation153]. The discovery of a nuclear complex containing all three proteins points to multiple drug targets to disrupt this complex therapeutically in cancer. In addition to PIPKIα [Citation25], multiple nuclear PI enzymes, including IPMK [Citation80], PTEN [Citation87], and SHIP1 [Citation36] are associated with p53, illuminating a central role for PI signaling in regulating p53 function.
The nuclear compartment for phosphoinositides
A fundamental issue in nuclear PI signaling is the question of how the PIs are compartmentalized in the nucleus [Citation8,Citation9,Citation20,Citation50,Citation127,Citation154]. Although there is evidence for vesicular compartments that contain PIs that major PI content appears to be separate from membranes, suggesting novel protein-lipid complexes that remain to be defined.
Conclusions
A flourishing body of literature from many groups provide unequivocal evidence that nuclear PIs serve as central signaling hubs for regulating many nuclear processes, especially in response to cellular stress (). However, it is also clear that we are only starting to scratch the surface in understanding the diverse biological roles of nuclear PIs and their effectors. Many crucial questions remain unanswered. For example, how do these phospholipids exist outside of membrane structures within the nucleoplasm? Do they play roles in LLPS for membrane-less compartmentalization? Where is their lipid origin? How are they held soluble by nuclear proteins? Do they link to nuclear proteins covalently or non-covalently? What advantage would phosphorylating a nuclear protein-bound PI have over simply phosphorylating one amino acid in the nuclear protein itself? How do the nuclear PIs and effectors sense cellular stress? Are there nuclear PI-scaffolds similar to the cytosolic ones [Citation155,Citation156]? Continued research efforts are needed to address these questions, to discover novel interactors, to decipher the nature of the PI-protein interaction, and to clarify the role of nuclear PIs. With many new tools in place to accelerate discovery in this field, many new insights regarding nuclear PIs and their associated proteins are likely to emerge in the next decade.
Figure 4. Schematic illustration of nuclear PI signaling in response to cellular stress.
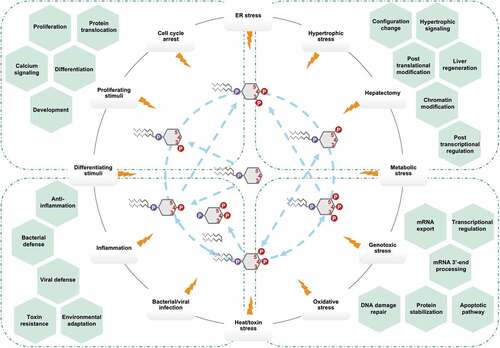
Acknowledgments
We wish to thank members of the Anderson and Cryns lab and apologize to the authors whose work was not cited due to space limitations. This work was supported in part by a National Institutes of Health grant GM114386 (R.A.A.), Department of Defense Breast Cancer Research Program grants W81XWH-17-1-0258 (R.A.A.) and W81XWH-17-1-0259 (V.L.C.), and a grant from the Breast Cancer Research Foundation (V.L.C.).
Disclosure statement
No potential conflict of interest was reported by the authors.
Additional information
Funding
References
- Hokin MR, Hokin LE. Enzyme secretion and the incorporation of P32 into phospholipides of pancreas slices. J Biol Chem. 1953;203:967–977.
- Di Paolo G, De Camilli P. Phosphoinositides in cell regulation and membrane dynamics. Nature. 2006;443:651–657.
- Rose HG, Frenster JH. Composition and metabolism of lipids within repressed and active chromatin of interphase lymphocytes. Biochim Biophys Acta. 1965;106:577–591.
- Manzoli FA, Maraldi NM, Cocco L, et al. Chromatin phospholipids in normal and chronic lymphocytic leukemia lymphocytes. Cancer Res. 1977;37:843–849.
- Smith CD, Wells WW. Phosphorylation of rat liver nuclear envelopes. II. Characterization of in vitro lipid phosphorylation. J Biol Chem. 1983;258:9368–9373.
- Cocco L, Gilmour RS, Ognibene A, et al. Synthesis of polyphosphoinositides in nuclei of friend cells. Evidence for polyphosphoinositide metabolism inside the nucleus which changes with cell differentiation. Biochem J. 1987;248:765–770.
- Boronenkov IV, Loijens JC, Umeda M, et al. Phosphoinositide signaling pathways in nuclei are associated with nuclear speckles containing pre-mRNA processing factors. Mol Biol Cell. 1998;9:3547–3560.
- Barlow CA, Laishram RS, Anderson RA. Nuclear phosphoinositides: a signaling enigma wrapped in a compartmental conundrum. Trends Cell Biol. 2010;20:25–35.
- Sztacho M, Sobol M, Balaban C, et al. Nuclear phosphoinositides and phase separation: important players in nuclear compartmentalization. Adv Biol Regul. 2019;71:111–117.
- Castano E, Yildirim S, Faberova V, et al. Nuclear phosphoinositides-versatile regulators of genome functions. Cells. 2019;8(7):649.
- Dickson EJ, Hille B. Understanding phosphoinositides: rare, dynamic, and essential membrane phospholipids. Biochem J. 2019;476:1–23.
- Shisheva A. Regulating Glut4 vesicle dynamics by phosphoinositide kinases and phosphoinositide phosphatases. Front Biosci. 2003;8:s945–s946.
- Rameh LE, Cantley LC. The role of phosphoinositide 3-kinase lipid products in cell function. J Biol Chem. 1999;274:8347–8350.
- Liu Y, Bankaitis VA. Phosphoinositide phosphatases in cell biology and disease. Prog Lipid Res. 2010;49:201–217.
- Sarkes D, Rameh LE. A novel HPLC-based approach makes possible the spatial characterization of cellular PtdIns5P and other phosphoinositides. Biochem J. 2010;428:375–384.
- Bryant JM, Blind RD. Signaling through non-membrane nuclear phosphoinositide binding proteins in human health and disease. J Lipid Res. 2019;60:299–311.
- Fu P, Ebenezer DL, Ha AW, et al. Nuclear lipid mediators: role of nuclear sphingolipids and sphingosine-1-phosphate signaling in epigenetic regulation of inflammation and gene expression. J Cell Biochem. 2018;119:6337–6353.
- Poli A, Billi AM, Mongiorgi S, et al. Nuclear phosphatidylinositol signaling: focus on phosphatidylinositol phosphate kinases and phospholipases C. J Cell Physiol. 2016;231:1645–1655.
- Hamann BL, Blind RD. Nuclear phosphoinositide regulation of chromatin. J Cell Physiol. 2018;233:107–123.
- Jacobsen RG, Mazloumi Gavgani F, Edson AJ, et al. Polyphosphoinositides in the nucleus: roadmap of their effectors and mechanisms of interaction. Adv Biol Regul. 2019;72:7–21.
- Shah ZH, Jones DR, Sommer L, et al. Nuclear phosphoinositides and their impact on nuclear functions. Febs J. 2013;280:6295–6310.
- Gillooly DJ, Morrow IC, Lindsay M, et al. Localization of phosphatidylinositol 3-phosphate in yeast and mammalian cells. Embo J. 2000;19:4577–4588.
- Kalasova I, Faberova V, Kalendova A, et al. Tools for visualization of phosphoinositides in the cell nucleus. Histochem Cell Biol. 2016;145:485–496.
- Sindic A, Aleksandrova A, Fields AP, et al. Presence and activation of nuclear phosphoinositide 3-kinase C2beta during compensatory liver growth. J Biol Chem. 2001;276:17754–17761.
- Choi S, Chen M, Cryns VL, et al. A nuclear phosphoinositide kinase complex regulates p53. Nat Cell Biol. 2019;21:462–475.
- Clarke JH, Letcher AJ, D’Santos CS, et al. Inositol lipids are regulated during cell cycle progression in the nuclei of murine erythroleukaemia cells. Biochem J. 2001;357:905–910.
- Zhang L, Malik S, Pang J, et al. Phospholipase Cepsilon hydrolyzes perinuclear phosphatidylinositol 4-phosphate to regulate cardiac hypertrophy. Cell. 2013;153:216–227.
- Wang YH, Hariharan A, Bastianello G, et al. DNA damage causes rapid accumulation of phosphoinositides for ATR signaling. Nat Commun. 2017;8:2118.
- Gozani O, Karuman P, Jones DR, et al. The PHD finger of the chromatin-associated protein ING2 functions as a nuclear phosphoinositide receptor. Cell. 2003;114:99–111.
- Jones DR, Bultsma Y, Keune WJ, et al. Nuclear PtdIns5P as a transducer of stress signaling: an in vivo role for PIP4Kbeta. Mol Cell. 2006;23:685–695.
- Bua DJ, Martin GM, Binda O, et al. Nuclear phosphatidylinositol-5-phosphate regulates ING2 stability at discrete chromatin targets in response to DNA damage. Sci Rep. 2013;3:2137.
- Bunce MW, Boronenkov IV, Anderson RA. Coordinated activation of the nuclear ubiquitin ligase Cul3-SPOP by the generation of phosphatidylinositol 5-phosphate. J Biol Chem. 2008;283:8678–8686.
- Poli A, Zaurito AE, Abdul-Hamid S, et al. Phosphatidylinositol 5 phosphate (PI5P): from behind the scenes to the front (nuclear) stage. Int J Mol Sci. 2019;20(9):2080.
- Van der Kaay J, Beck M, Gray A, et al. Distinct phosphatidylinositol 3-kinase lipid products accumulate upon oxidative and osmotic stress and lead to different cellular responses. J Biol Chem. 1999;274:35963–35968.
- Deleris P, Bacqueville D, Gayral S, et al. SHIP-2 and PTEN are expressed and active in vascular smooth muscle cell nuclei, but only SHIP-2 is associated with nuclear speckles. J Biol Chem. 2003;278:38884–38891.
- Ehm P, Nalaskowski MM, Wundenberg T, et al. The tumor suppressor SHIP1 colocalizes in nucleolar cavities with p53 and components of PML nuclear bodies. Nucleus. 2015;6:154–164.
- Yokogawa T, Nagata S, Nishio Y, et al. Evidence that 3 ‘-phosphorylated polyphosphoinositides are generated at the nuclear surface: use of immunostaining technique with monoclonal antibodies specific for PI 3,4-P-2. Febs Lett. 2000;473:222–226.
- Osborne SL, Thomas CL, Gschmeissner S, et al. Nuclear PtdIns(4,5)P2 assembles in a mitotically regulated particle involved in pre-mRNA splicing. J Cell Sci. 2001;114:2501–2511.
- Yoo SH, Huh YH, Huh SK, et al. Localization and projected role of phosphatidylinositol 4-kinases IIalpha and IIbeta in inositol 1,4,5-trisphosphate-sensitive nucleoplasmic Ca(2)(+) store vesicles. Nucleus. 2014;5:341–351.
- Watt SA, Kular G, Fleming IN, et al. Subcellular localization of phosphatidylinositol 4,5-bisphosphate using the pleckstrin homology domain of phospholipase C delta1. Biochem J. 2002;363:657–666.
- Sobol M, Krausova A, Yildirim S, et al. Nuclear phosphatidylinositol 4,5-bisphosphate islets contribute to efficient RNA polymerase II-dependent transcription. J Cell Sci. 2018;131:8.
- Li W, Laishram RS, Ji Z, et al. Star-PAP control of BIK expression and apoptosis is regulated by nuclear PIPKIalpha and PKCdelta signaling. Mol Cell. 2012;45:25–37.
- Mellman DL, Gonzales ML, Song C, et al. A PtdIns4,5P2-regulated nuclear poly(A) polymerase controls expression of select mRNAs. Nature. 2008;451:1013–1017.
- Mishkind M, Vermeer JE, Darwish E, et al. Heat stress activates phospholipase D and triggers PIP accumulation at the plasma membrane and nucleus. Plant J. 2009;60:10–21.
- Lindsay Y, McCoull D, Davidson L, et al. Localization of agonist-sensitive PtdIns(3,4,5)P3 reveals a nuclear pool that is insensitive to PTEN expression. J Cell Sci. 2006;119:5160–5168.
- Karlsson T, Altankhuyag A, Dobrovolska O, et al. A polybasic motif in ErbB3-binding protein 1 (EBP1) has key functions in nucleolar localization and polyphosphoinositide interaction. Biochem J. 2016;473:2033–2047.
- Tanaka K, Horiguchi K, Yoshida T, et al. Evidence that a phosphatidylinositol 3,4,5-trisphosphate-binding protein can function in nucleus. J Biol Chem. 1999;274:3919–3922.
- Kobayashi M, Muroyama A, Ohizumi Y. Phosphatidylinositol 4,5-bisphosphate enhances calcium release from sarcoplasmic reticulum of skeletal muscle. Biochem Biophys Res Commun. 1989;163:1487–1491.
- Prole DL, Taylor CW. Structure and function of IP3 receptors. Cold Spring Harb Perspect Biol. 2019;11(4).
- Romanauska A, Kohler A. The inner nuclear membrane is a metabolically active territory that generates nuclear lipid droplets. Cell. 2018;174:700-+.
- Payrastre B, Nievers M, Boonstra J, et al. A differential location of phosphoinositide kinases, diacylglycerol kinase, and phospholipase-C in the nuclear matrix. J Biol Chem. 1992;267:5078–5084.
- Zima AV, Bare DJ, Mignery GA, et al. IP3-dependent nuclear Ca2+ signalling in the mammalian heart. J Physiol. 2007;584:601–611.
- Humbert JP, Matter N, Artault JC, et al. Inositol 1,4,5-trisphosphate receptor is located to the inner nuclear membrane vindicating regulation of nuclear calcium signaling by inositol 1,4,5-trisphosphate. Discrete distribution of inositol phosphate receptors to inner and outer nuclear membranes (vol 271, pg 478, 1996). J Biol Chem. 1996;271:5287.
- Echevarria W, Leite MF, Guerra MT, et al. Regulation of calcium signals in the nucleus by a nucleoplasmic reticulum. Nat Cell Biol. 2003;5:440–446.
- Tabellini G, Bortul R, Santi S, et al. Diacylglycerol kinase-theta is localized in the speckle domains of the nucleus. Exp Cell Res. 2003;287:143–154.
- Neri LM, Bortul R, Borgatti P, et al. Proliferating or differentiating stimuli act on different lipid-dependent signaling pathways in nuclei of human leukemia cells. Mol Biol Cell. 2002;13:947–964.
- Zhang H, Shao Z, Alibin CP, et al. Liganded peroxisome proliferator-activated receptors (PPARs) preserve nuclear histone deacetylase 5 levels in endothelin-treated Sprague-Dawley rat cardiac myocytes. PLoS One. 2014;9:e115258.
- Arantes LAM, Aguiar CJ, Amaya MJ, et al. Nuclear inositol 1,4,5-trisphosphate is a necessary and conserved signal for the induction of both pathological and physiological cardiomyocyte hypertrophy. J Mol Cell Cardiol. 2012;53:475–486.
- Balla A, Balla T. Phosphatidylinositol 4-kinases: old enzymes with emerging functions. Trends Cell Biol. 2006;16:351–361.
- Boura E, Nencka R. Phosphatidylinositol 4-kinases: function, structure, and inhibition. Exp Cell Res. 2015;337:136–145.
- Kakuk A, Friedlander E, Vereb G Jr., et al. Nucleolar localization of phosphatidylinositol 4-kinase PI4K230 in various mammalian cells. Cytometry A. 2006;69:1174–1183.
- de Graaf P, Klapisz EE, Schulz TK, et al. Nuclear localization of phosphatidylinositol 4-kinase beta. J Cell Sci. 2002;115:1769–1775.
- Szivak I, Lamb N, Heilmeyer LM. Subcellular localization and structural function of endogenous phosphorylated phosphatidylinositol 4-kinase (PI4K92). J Biol Chem. 2006;281:16740–16749.
- Heilmeyer LM Jr., Vereb G Jr., Vereb G, et al. Mammalian phosphatidylinositol 4-kinases. IUBMB Life. 2003;55:59–65.
- Schill NJ, Anderson RA. Two novel phosphatidylinositol-4-phosphate 5-kinase type Igamma splice variants expressed in human cells display distinctive cellular targeting. Biochem J. 2009;422:473–482.
- Chakrabarti R, Sanyal S, Ghosh A, et al. Phosphatidylinositol-4-phosphate 5-kinase 1alpha modulates ribosomal RNA gene Silencing through its interaction with histone H3 lysine 9 trimethylation and heterochromatin protein HP1-alpha. J Biol Chem. 2015;290:20893–20903.
- Ciruela A, Hinchliffe KA, Divecha N, et al. Nuclear targeting of the beta isoform of type II phosphatidylinositol phosphate kinase (phosphatidylinositol 5-phosphate 4-kinase) by its alpha-helix 7. Biochem J. 2000;346(Pt 3):587–591.
- Richardson JP, Wang MC, Clarke JH, et al. Genomic tagging of endogenous Type II beta phosphatidylinositol 5-phosphate 4-kinase in DT40 cells reveals a nuclear localisation. Cell Signal. 2007;19:1309–1314.
- Kouchi Z, Fujiwara Y, Yamaguchi H, et al. Phosphatidylinositol 5-phosphate 4-kinase type II beta is required for vitamin D receptor-dependent E-cadherin expression in SW480 cells. Biochem Biophys Res Commun. 2011;408:523–529.
- Stijf-Bultsma Y, Sommer L, Tauber M, et al. The basal transcription complex component TAF3 transduces changes in nuclear phosphoinositides into transcriptional output. Mol Cell. 2015;58:453–467.
- Jean S, Kiger AA. Classes of phosphoinositide 3-kinases at a glance. J Cell Sci. 2014;127:923–928.
- Kumar A, Redondo-Munoz J, Perez-Garcia V, et al. Nuclear but not cytosolic phosphoinositide 3-kinase beta has an essential function in cell survival. Mol Cell Biol. 2011;31:2122–2133.
- Kumar A, Fernandez-Capetillo O, Carrera AC. Nuclear phosphoinositide 3-kinase beta controls double-strand break DNA repair. Proc Natl Acad Sci U S A. 2010;107:7491–7496.
- Marques M, Kumar A, Poveda AM, et al. Specific function of phosphoinositide 3-kinase beta in the control of DNA replication. Proc Natl Acad Sci U S A. 2009;106:7525–7530.
- Didichenko SA, Thelen M. Phosphatidylinositol 3-kinase c2alpha contains a nuclear localization sequence and associates with nuclear speckles. J Biol Chem. 2001;276:48135–48142.
- Banfic H, Visnjic D, Mise N, et al. Epidermal growth factor stimulates translocation of the class II phosphoinositide 3-kinase PI3K-C2beta to the nucleus. Biochem J. 2009;422:53–60.
- Resnick AC, Snowman AM, Kang BN, et al. Inositol polyphosphate multikinase is a nuclear P13-kinase with transcriptional regulatory activity. Proc Natl Acad Sci U S A. 2005;102:12783–12788.
- Kim E, Beon J, Lee S, et al. IPMK: A versatile regulator of nuclear signaling events. Adv Biol Regul. 2016;61:25–32.
- Blind RD, Suzawa M, Ingraham HA. Direct modification and activation of a nuclear receptor-PIP(2) complex by the inositol lipid kinase IPMK. Sci Signal. 2012;5:ra44.
- Xu R, Sen N, Paul BD, et al. Inositol polyphosphate multikinase is a coactivator of p53-mediated transcription and cell death. Sci Signal. 2013;6:ra22.
- Lee YR, Chen M, Pandolfi PP. The functions and regulation of the PTEN tumour suppressor: new modes and prospects. Nat Rev Mol Cell Biol. 2018;19:547–562.
- Li P, Wang D, Li H, et al. Identification of nucleolus-localized PTEN and its function in regulating ribosome biogenesis. Mol Biol Rep. 2014;41:6383–6390.
- Shen WH, Balajee AS, Wang J, et al. Essential role for nuclear PTEN in maintaining chromosomal integrity. Cell. 2007;128:157–170.
- Choi BH, Chen Y, Dai W. Chromatin PTEN is involved in DNA damage response partly through regulating Rad52 sumoylation. Cell Cycle. 2013;12:3442–3447.
- Chang CJ, Mulholland DJ, Valamehr B, et al. PTEN nuclear localization is regulated by oxidative stress and mediates p53-dependent tumor suppression. Mol Cell Biol. 2008;28:3281–3289.
- Trotman LC, Pandolfi PP. PTEN and p53: who will get the upper hand?. Cancer Cell. 2003;3:97–99.
- Freeman DJ, Li AG, Wei G, et al. PTEN tumor suppressor regulates p53 protein levels and activity through phosphatase-dependent and -independent mechanisms. Cancer Cell. 2003;3:117–130.
- Mayo LD, Donner DB. The PTEN, Mdm2, p53 tumor suppressor-oncoprotein network. Trends Biochem Sci. 2002;27:462–467.
- Mayo LD, Dixon JE, Durden DL, et al. PTEN protects p53 from Mdm2 and sensitizes cancer cells to chemotherapy. J Biol Chem. 2002;277:5484–5489.
- Stambolic V, MacPherson D, Sas D, et al. Regulation of PTEN transcription by p53. Mol Cell. 2001;8:317–325.
- Elong Edimo W, Derua R, Janssens V, et al. Evidence of SHIP2 Ser132 phosphorylation, its nuclear localization and stability. Biochem J. 2011;439:391–401.
- Zou J, Marjanovic J, Kisseleva MV, et al. Type I phosphatidylinositol-4, 5-bisphosphate 4-phosphatase regulates stress-induced apoptosis. Proc Natl Acad Sci U S A. 2007;104:16834–16839.
- Cocco L, Rubbini S, Manzoli L, et al. Inositides in the nucleus: presence and characterisation of the isozymes of phospholipase beta family in NIH 3T3 cells. Biochim Biophys Acta. 1999;1438:295–299.
- Lee YH, Kim SY, Kim JR, et al. Overexpression of phospholipase Cbeta-1 protects NIH3T3 cells from oxidative stress-induced cell death. Life Sci. 2000;67:827–837.
- Stallings JD, Tall EG, Pentyala S, et al. Nuclear translocation of phospholipase C-delta(1) is linked to the cell cycle and nuclear phosphatidylinositol 4,5-bisphosphate. J Biol Chem. 2005;280:22060–22069.
- Kunrath-Lima M, de Miranda MC, Ferreira ADF, et al. Phospholipase C delta 4 (PLCdelta4) is a nuclear protein involved in cell proliferation and senescence in mesenchymal stromal stem cells. Cell Signal. 2018;49:59–67.
- Chi B, Wang Q, Wu G, et al. Aly and THO are required for assembly of the human TREX complex and association of TREX components with the spliced mRNA. Nucleic Acids Res. 2013;41:1294–1306.
- Okada M, Jang SW, Ye K. Akt phosphorylation and nuclear phosphoinositide association mediate mRNA export and cell proliferation activities by ALY. Proc Natl Acad Sci U S A. 2008;105:8649–8654.
- Wickramasinghe VO, Savill JM, Chavali S, et al. Human inositol polyphosphate multikinase regulates transcript-selective nuclear mRNA export to preserve genome integrity. Mol Cell. 2013;51:737–750.
- Toska E, Campbell HA, Shandilya J, et al. Repression of transcription by WT1-BASP1 requires the myristoylation of BASP1 and the PIP2-dependent recruitment of histone deacetylase. Cell Rep. 2012;2:462–469.
- Wu C, Wang Y, Xia Y, et al. Wilms’ tumor 1 enhances Cisplatin-resistance of advanced NSCLC. Febs Lett. 2014;588:4566–4572.
- Gadadhar S, Bora N, Tiwari V, et al. Sequestration of the abrin A chain to the nucleus by BASP1 increases the resistance of cells to abrin toxicity. Biochem J. 2014;458:375–385.
- Kadoch C, Crabtree GR. Mammalian SWI/SNF chromatin remodeling complexes and cancer: mechanistic insights gained from human genomics. Sci Adv. 2015;1:e1500447.
- Khan P, Drobic B, Perez-Cadahia B, et al. Mitogen- and stress-activated protein kinases 1 and 2 are required for maximal trefoil factor 1 induction. PLoS One. 2013;8:e63189.
- Li F, Liang J, Tang D. Brahma-related gene 1 ameliorates the neuronal apoptosis and oxidative stress induced by oxygen-glucose deprivation/reoxygenation through activation of Nrf2/HO-1 signaling. Biomed Pharmacother. 2018;108:1216–1224.
- Porter EG, Dhiman A, Chowdhury B, et al. PBRM1 regulates stress response in epithelial cells. iScience. 2019;15:196–210.
- Zhao K, Wang W, Rando OJ, et al. Rapid and phosphoinositol-dependent binding of the SWI/SNF-like BAF complex to chromatin after T lymphocyte receptor signaling. Cell. 1998;95:625–636.
- Rando OJ, Zhao K, Janmey P, et al. Phosphatidylinositol-dependent actin filament binding by the SWI/SNF-like BAF chromatin remodeling complex. Proc Natl Acad Sci U S A. 2002;99:2824–2829.
- Shubina MY, Musinova YR, Sheval EV. Nucleolar methyltransferase fibrillarin: evolution of structure and functions. Biochemistry (Mosc). 2016;81:941–950.
- Yildirim S, Castano E, Sobol M, et al. Involvement of phosphatidylinositol 4,5-bisphosphate in RNA polymerase I transcription. J Cell Sci. 2013;126:2730–2739.
- Tiku V, Kew C, Mehrotra P, et al. Nucleolar fibrillarin is an evolutionarily conserved regulator of bacterial pathogen resistance. Nat Commun. 2018;9(1):3607.
- Sobol M, Yildirim S, Philimonenko VV, et al. UBF complexes with phosphatidylinositol 4,5-bisphosphate in nucleolar organizer regions regardless of ongoing RNA polymerase I activity. Nucleus. 2013;4:478–486.
- Kodiha M, Banski P, Stochaj U. Computer-based fluorescence quantification: a novel approach to study nucleolar biology. BMC Cell Biol. 2011;12:25.
- Nagashima M, Shiseki M, Miura K, et al. DNA damage-inducible gene p33ING2 negatively regulates cell proliferation through acetylation of p53. Proc Natl Acad Sci U S A. 2001;98:9671–9676.
- Lindstrom MS. NPM1/B23: A multifunctional chaperone in ribosome biogenesis and chromatin remodeling. Biochem Res Int. 2011;2011:195209.
- Li J, Zhang X, Sejas DP, et al. Negative regulation of p53 by nucleophosmin antagonizes stress-induced apoptosis in human normal and malignant hematopoietic cells. Leuk Res. 2005;29:1415–1423.
- Kurki S, Peltonen K, Latonen L, et al. Nucleolar protein NPM interacts with HDM2 and protects tumor suppressor protein p53 from HDM2-mediated degradation. Cancer Cell. 2004;5:465–475.
- Borer RA, Lehner CF, Eppenberger HM, et al. Major nucleolar proteins shuttle between nucleus and cytoplasm. Cell. 1989;56:379–390.
- Ahn JY, Liu X, Cheng D, et al. Nucleophosmin/B23, a nuclear PI(3,4,5)P(3) receptor, mediates the antiapoptotic actions of NGF by inhibiting CAD. Mol Cell. 2005;18:435–445.
- Feric M, Vaidya N, Harmon TS, et al. Coexisting liquid phases underlie nucleolar subcompartments. Cell. 2016;165:1686–1697.
- Mitrea DM, Cika JA, Stanley CB, et al. Self-interaction of NPM1 modulates multiple mechanisms of liquid-liquid phase separation. Nat Commun. 2018;9:842.
- Ulicna L, Kalendova A, Kalasova I, et al. PIP2 epigenetically represses rRNA genes transcription interacting with PHF8. Biochim Biophys Acta Mol Cell Biol Lipids. 2018;1863:266–275.
- Wang Q, Ma S, Song N, et al. Stabilization of histone demethylase PHF8 by USP7 promotes breast carcinogenesis. J Clin Invest. 2016;126:2205–2220.
- Viiri KM, Janis J, Siggers T, et al. DNA-binding and -bending activities of SAP30L and SAP30 are mediated by a zinc-dependent module and monophosphoinositides. Mol Cell Biol. 2009;29:342–356.
- Viiri KM, Korkeamaki H, Kukkonen MK, et al. SAP30L interacts with members of the Sin3A corepressor complex and targets Sin3A to the nucleolus. Nucleic Acids Res. 2006;34:3288–3298.
- Krylova IN, Sablin EP, Moore J, et al. Structural analyses reveal phosphatidyl inositols as ligands for the NR5 orphan receptors SF-1 and LRH-1. Cell. 2005;120:343–355.
- Blind RD, Sablin EP, Kuchenbecker KM, et al. The signaling phospholipid PIP3 creates a new interaction surface on the nuclear receptor SF-1. Proc Natl Acad Sci U S A. 2014;111:15054–15059.
- Bland ML, Jamieson CA, Akana SF, et al. Haploinsufficiency of steroidogenic factor-1 in mice disrupts adrenal development leading to an impaired stress response. Proc Natl Acad Sci U S A. 2000;97:14488–14493.
- Mortier E, Wuytens G, Leenaerts I, et al. Nuclear speckles and nucleoli targeting by PIP2-PDZ domain interactions. Embo J. 2005;24:2556–2565.
- Liu XL, Gao QQ, Li PS, et al. UHRF1 targets DNMT1 for DNA methylation through cooperative binding of hemi-methylated DNA and methylated H3K9. Nat Commun. 2013;4:1563.
- Bronner C, Fuhrmann G, Chedin FL, et al. UHRF1 links the histone code and DNA methylation to ensure faithful epigenetic memory inheritance. Genet Epigenet. 2010;2009:29–36.
- Wang F, Yang YZ, Shi CZ, et al. UHRF1 promotes cell growth and metastasis through repression of p16(ink4a) in colorectal cancer. Ann Surg Oncol. 2012;19:2753–2762.
- Gelato KA, Tauber M, Ong MS, et al. Accessibility of different histone H3-binding domains of UHRF1 is allosterically regulated by phosphatidylinositol 5-phosphate. Mol Cell. 2014;54:905–919.
- Tian YY, Paramasivam M, Ghosal G, et al. UHRF1 contributes to DNA damage repair as a lesion recognition factor and nuclease scaffold. Cell Rep. 2015;10:1957–1966.
- Abu-Alainin W, Gana T, Liloglou T, et al. UHRF1 regulation of the Keap1-Nrf2 pathway in pancreatic cancer contributes to oncogenesis. J Pathol. 2016;238:423–433.
- Bronner C, Achour M, Arima Y, et al. The UHRF family: oncogenes that are drugable targets for cancer therapy in the near future? Pharmacol Ther. 2007;115:419–434.
- Unoki M, Brunet J, Mousli M. Drug discovery targeting epigenetic codes: the great potential of UHRF1, which links DNA methylation and histone modifications, as a drug target in cancers and toxoplasmosis. Biochem Pharmacol. 2009;78:1279–1288.
- Trippe R, Guschina E, Hossbach M, et al. Identification, cloning, and functional analysis of the human U6 snRNA-specific terminal uridylyl transferase. RNA. 2006;12:1494–1504.
- Li W, Li W, Laishram RS, et al. Distinct regulation of alternative polyadenylation and gene expression by nuclear poly(A) polymerases. Nucleic Acids Res. 2017;45:8930–8942.
- Laishram RS, Anderson RA. The poly A polymerase star-PAP controls 3ʹ-end cleavage by promoting CPSF interaction and specificity toward the pre-mRNA. Embo J. 2010;29:4132–4145.
- Laishram RS, Barlow CA, Anderson RA. CKI isoforms α and ε regulate star-PAP target messages by controlling star-PAP poly(A) polymerase activity and phosphoinositide stimulation. Nucleic Acids Res. 2011;39:7961–7973.
- Dai C, Gu W. p53 post-translational modification: deregulated in tumorigenesis. Trends Mol Med. 2010;16:528–536.
- Bieging KT, Mello SS, Attardi LD. Unravelling mechanisms of p53-mediated tumour suppression. Nat Rev Cancer. 2014;14:359–370.
- Kruiswijk F, Labuschagne CF, Vousden KH. p53 in survival, death and metabolic health: a lifeguard with a licence to kill. Nat Rev Mol Cell Biol. 2015;16:393–405.
- Freed-Pastor WA, Prives C. Mutant p53: one name, many proteins. Genes Dev. 2012;26:1268–1286.
- Muller PA, Vousden KH. Mutant p53 in cancer: new functions and therapeutic opportunities. Cancer Cell. 2014;25:304–317.
- Weibrecht I, Leuchowius KJ, Clausson CM, et al. Proximity ligation assays: a recent addition to the proteomics toolbox. Expert Rev Proteomics. 2010;7:401–409.
- Sehat B, Tofigh A, Lin Y, et al. SUMOylation mediates the nuclear translocation and signaling of the IGF-1 receptor. Sci Signal. 2010;3:ra10.
- Chen M, Qiu T, Wu J, et al. Extracellular anti-angiogenic proteins augment an endosomal protein trafficking pathway to reach mitochondria and execute apoptosis in HUVECs. Cell Death Differ. 2018;25:1905–1920.
- Chen M, Zhang Y, Yu VC, et al. Isthmin targets cell-surface GRP78 and triggers apoptosis via induction of mitochondrial dysfunction. Cell Death Differ. 2014;21:797–810.
- Semenas J, Hedblom A, Miftakhova RR, et al. The role of PI3K/AKT-related PIP5K1alpha and the discovery of its selective inhibitor for treatment of advanced prostate cancer. Proc Natl Acad Sci U S A. 2014;111:E3689–E3698.
- Arrigo AP, Gibert B. HspB1, HspB5 and HspB4 in human cancers: potent oncogenic role of some of their client proteins. Cancers (Basel). 2014;6:333–365.
- Malin D, Petrovic V, Strekalova E, et al. alphaB-crystallin: portrait of a malignant chaperone as a cancer therapeutic target. Pharmacol Ther. 2016;160:1–10.
- Fiume R, Faenza I, Sheth B, et al. Nuclear phosphoinositides: their regulation and roles in nuclear functions. Int J Mol Sci. 2019;20:2991.
- Choi S, Hedman AC, Sayedyahossein S, et al. Agonist-stimulated phosphatidylinositol-3,4,5-trisphosphate generation by scaffolded phosphoinositide kinases. Nat Cell Biol. 2016;18:1324–1335.
- Chen M, Choi S, Jung O, et al. The specificity of EGF-stimulated IQGAP1 scaffold towards the PI3K-Akt pathway is defined by the IQ3 motif. Sci Rep. 2019;9:9126.
- Kakuk A, Friedlander E, Vereb G Jr., et al. Nuclear and nucleolar localization signals and their targeting function in phosphatidylinositol 4-kinase PI4K230. Exp Cell Res. 2008;314:2376–2388.
- Visnjic D, Crljen V, Curic J, et al. The activation of nuclear phosphoinositide 3-kinase C2beta in all-trans-retinoic acid-differentiated HL-60 cells. Febs Lett. 2002;529:268–274.
- Oh SM, Liu Z, Okada M, et al. Ebp1 sumoylation, regulated by TLS/FUS E3 ligase, is required for its anti-proliferative activity. Oncogene. 2010;29:1017–1030.
- Geeraerts A, Hsiu-Fang F, Zimmermann P, et al. The characterization of the nuclear dynamics of syntenin-2, a PIP2 binding PDZ protein. Cytometry A. 2013;83:866–875.