ABSTRACT
The severe acute respiratory syndrome coronavirus 2 (SARS-CoV-2) causes COVID-19. Until now, diverse drugs have been used for the treatment of COVID-19. These drugs are associated with severe side effects, e.g. induction of erythrocyte death, named eryptosis. This massively affects the oxygen (O2) supply of the organism. Therefore, three elementary aspects should be considered simultaneously: (1) a potential drug should directly attack the virus, (2) eliminate virus-infected host cells and (3) preserve erythrocyte survival and functionality. It is known that PKC-α inhibition enhances the vitality of human erythrocytes, while it dose-dependently activates the apoptosis machinery in nucleated cells. Thus, the use of chelerythrine as a specific PKC-alpha and -beta (PKC-α/-β) inhibitor should be a promising approach to treat people infected with SARS-CoV-2.
Introduction
The error-prone nature of RNA polymerases, a pre-requisite for fast genomes evolution of RNA viruses
Evolutionary more highly evolved species with their corresponding large genome size require sophisticated energy- and time-consuming mechanisms to produce precise and viable genome copies. This needs DNA polymerases to operate with the highest degree of proofreading to keep error frequencies and mutation rates very low. However, there are exceptions, i.e. suppression or bypass of proofreading mechanisms in some phases of B-cell development to generate the greatest variability of immunoglobulin molecules [Citation1,Citation2] (for reviews see: [Citation3,Citation4]). This is an important aspect of the immune response to maintain the genetic health of mammalian populations.
RNA replicating viruses are naturally equipped with an error-prone RNA polymerase, allowing them a “turn-on-mutagenesis” status and thus, the highest mutation rates and variability, rapid adaptability and fast genomes evolution [Citation5]. Thus, the RNA viruses are always in attack mode in terms of variability and pathogenicity, and their host must first develop a suitable immune response. The single-stranded RNA virus SARS-CoV-2 with its ~30 kb genome and remarkable capability to transmit between humans, caused COVID-19 [Citation6], affected public life worldwide in an unprecedented way and has so far cost a million people their lives. The respiratory disease COVID-19 has a devastating effect on the health of elderly and people with preexisting conditions, e.g. immunocompromised patients. Currently, several substances are used for COVID-19 treatment, such as glucocorticoids [Citation7] and anti-malaria agents, e.g. chloroquine [Citation8]. The latter usually induce eryptosis. Chloroquine exacerbates virus-associated anemia by enhancing virus replication [Citation9]. Glucocorticoids limit the production of pro-inflammatory cytokines, but as immunosuppressants they also impair the protective function of the T cells, which are urgently needed to combat COVID-19. Furthermore, glucocorticoids inhibit the activity of macrophages and antibody-producing B cells. Thus, a sustained increase in plasma viral load is pre-programmed [Citation10]. In this context, glucocorticoids´ ability to limit the overproduction of inflammatory cytokines triggered by SARS-CoV-2 should not be overestimated. Hence, the use of substances for COVID-19 treatment that inhibit the protective effect of immune cells and impair the functionality of erythrocytes or even induce their death is counterproductive. The immediate consequence is a sustained increase in plasma viral load and sub-optimal oxygen (O2) supply of the organism, respectively.
Immuno-modulatory and anti-inflammatory function of human erythrocytes
Human erythrocytes play a vital role in immune and defense mechanisms. They attach to bacteria, transport them to liver and spleen for final elimination [Citation11,Citation12]. Human erythrocytes function as a sink for sphingosine-1-phosphate (S1P) [Citation13] and release on demand this molecule into the plasma through a finely regulated mechanism. S1P negatively regulates lymphocytes circulation [Citation14], maintains endothelial cell barrier integrity [Citation15] and exerts a protective effect on kidney (for reviews see: [Citation16,Citation17]). In addition to this, kidney vascular development is positively regulated by interplay between S1P, erythroblasts and vascular endothelium [Citation18]. Each single human erythrocyte contains approximately 270 millions hemoglobin molecules and thus 1.1 billion heme groups. Heme inhibitory binding to pro-inflammatory cytokine IL-36α (in a ratio of 2:1) negatively regulates IL-36 dependent IL-6 and IL-8 expression [Citation19]. Heme-regulated proteins are involved in several physiological processes, e.g. protein synthesis [Citation20]. Physical interaction of human erythrocytes with a sub-group of dendritic cells (slanDCs) prevents their maturation. The maturation inhibitory effect of erythrocytes ends when slanDCs leave the blood and reach their target tissue, where they mature and acquire an IL-12 and TNF-α producing capacity to fight off pathogens [Citation21]. Human erythrocytes with their unique chemokine receptor are capable to bind an array of inflammatory chemokines [Citation22] including IL-8 [Citation23], and thus avoiding the excessive inflammatory response. Interestingly, 20% of SARS-CoV-2 infected patients develop acute respiratory distress syndrome which is associated with abundance release of chemokines and pro-inflammatory cytokines (e.g. IL-8) culminating in multi-organ failure (for review see: [Citation24]). These data show that human erythrocytes have not only respiratory but also immuno-modulatory and anti-inflammatory functions. Thus, the application of such substances which improve the vitality of erythrocytes and simultaneously eliminate pathogens or pathogen-infected host cells is the best choice and of utmost importance for treating COVID-19.
Activation of protein kinase C-alpha (PKC-α) in respiratory diseases, its role in nuclear export of viral genome and its inhibition by chelerythrine
Respiratory diseases of viral and bacterial origin are associated with PKC-α activation [Citation25,Citation26]. Viral life cycle follows a certain pattern. The negative-strand influenza A RNA virus exclusively persues a nuclear replication and PKC-α promotes the export of ribonucleoproteins (RNPs) complexes from the nucleus of infected cells [Citation27]. Subsequently, RNPs are packaged into budding progeny virions at the cell membrane. The following remarkable review illustrates the principle of virus assembly and budding for an influenza A virus [Citation28]. It is to note that maturation of progeny virions and the intracellular sites at which they bud is determined by the accumulation of their glycoproteins. This principle was demonstrated four decades ago with coronavirus MHV-A59 [Citation29]. Latest studies suggest that positive-strand SARS-CoV-2 RNA virus also follows a nuclear replication strategy [Citation30]. The suppression of PKC-α mediated nuclear export of viral RNPs will be one of the potential targets for chelerythrine, thereby disrupting the formation of the emerging SARS-CoV-2 virions. The following publications describe more details about the pathogenic mechanisms of SARS-CoV-2 [Citation31,Citation32]. The bioactive molecule chelerythrine, a natural product of plant origin is a specific PKC-α/-β inhibitor [Citation33–36], which can exist in its charged monomeric iminium or neutral alkanolamine forms, enhances the vitality and biological functions of human erythrocytes and prevents stress-induced eryptosis [Citation37]. Several studies underpin the versatility of chelerythrine’s mode of action in terms of apoptosis induction [Citation38–40], in vivo tumor growth delay [Citation35], kidney [Citation41] and lung protection. The inhibitory effect of chelerythrine on Gram-positive bacteria [Citation42], and its ability to bind to DNA (five nucleotides per chelerythrine) [Citation43–47] and RNA [Citation48] (for reviews see: [Citation49,Citation50]) further underscores the potential of chelerythrine for the use against many diseases including COVID-19.
Inhibitory effect of chelerythrine on virus-mediated PKC-α/-β activation and RNA polymerase phosphorylation
Viral RNA sensing retinoic acid-inducible gene I (RIG-I) as an integral part of host pattern recognition receptors is able to detect both single and double-stranded RNAs in cytosol and initiate the primary line of defense by interferon (IFN)-mediated anti-viral response [Citation25,Citation51,Citation52]. The following work shows some aspects of the SARS-Cov-2 mode of action to undermine IFN-signaling pathways [Citation32]. Both conventional PKC-α and PKC-β are negative regulators of RIG-I. Chelerythrine could reverse PKC-α/-β-mediated RIG-I inhibition and thus initiate the IFN-mediated immune response and, if necessary, depending on the concentration used, eliminate the virus-infected (nucleated) host cells by apoptosis. Several enzymes engaged in DNA and RNA metabolism, e.g. DNA-dependent polymerases and RNA-dependent polymerases, are phospho-activated proteins. It exists a direct correlation between phosphorylation level of hepatitis C RNA polymerase and its RNA replication [Citation53]. In this concept, chelerythrine-mediated inhibition of a putative kinase X activity should decrease RNA polymerase phosphorylation of SARS-CoV-2, severely impair RNA replication and simultaneously enhance RIG-I-dependent INF-mediated anti-viral immune responses. The reciprocal relationship between kinases and phosphatases activities suggests that the use of the kinase inhibitor chelerythrine automatically promotes phosphatase(s) activities. In addition, the protective effect of chelerythrine on human erythrocytes, especially in case of respiratory diseases, is another central point to consider chelerythrine as a potential drug for COVID-19 treatment.
Human erythrocytes vs. SARS-CoV-2
Intact human erythrocytes promote proliferation of activated CD8+ cells [Citation54] whereas they inhibit CD4+ proliferation [Citation55]; they actively adsorb infectious HIV-1 virions [Citation56] for review see: [Citation57], hence reducing HIV infection of CD4+ T-cells. The interaction between virus and the receptive cell is a carbohydrate-dependent mechanism. Human erythrocyte gangliosides [Citation58] as well as sialoglycoprotein glycophorin A (GPA), act as receptors for numerous viruses [Citation59–63] resulting in reduction of both viral infectivity and pathogen load in the circulation [Citation60,Citation64]. In other words, the larger the erythrocyte-bound fraction of a virus, the greater its blood clearance and elimination rate. Each single human erythrocyte with 1 × 106 GPA molecules is capable to bind 1.5 × 105 virions with maximum uptake attaining within 1–2 hours [Citation65,Citation66]. According to the same principle, intact human erythrocytes can capture not only SARS-CoV-2 but also the active particles of this virus and deliver them to macrophages resident in the spleen and liver for final elimination. The elimination of erythrocytes-bound pathogens is a common mechanism. Complement receptor 1-dependent attachment of erythrocytes to bacteria and viruses leads also to phagocytosis and elimination of erythrocyte-bound bacteria [Citation67] (for review see: [Citation68]) and viruses [Citation69,Citation70]. Furthermore, the complexity and high efficiency of the circulatory clearance of bacteria and the involvement of innate (liver, our firewall) and adaptive (spleen) immunity requires the contribution of additional cells such as platelets [Citation71,Citation72] for preview see: [Citation73].
Inhibitory effect of chelerythrine on mTORC2-dependent, mTORC1-mediated protein synthesis
Host cell protein synthesis does not necessarily have to be accompanied by its proliferation [Citation74]. This principle can be used by a wide variety of intracellular parasites to proliferate with high velocity, e.g. malaria, or to be multiplied, e.g. viruses. Intense multiplication of SARS-CoV-2 within a single host cell requires the activation of both mammalian targets of rapamycin complexes 1 (mTORC1) and 2 (mTORC2) and the associated protein synthesis machinery. mTORC2 acts as an upstream kinase of serum and glucocorticoid-inducible kinase-1 (SGK-1) and protein kinase B (PKB also called Akt) which in turn activate mTORC1. It is to note that mTORC2 kinase activity is PKC-α-dependent [Citation75]. Phosphorylation-dependent Akt activation is regulated by the following kinases: (a) PKC-α/mTORC2 and (b) phosphoinositide 3-kinase (PI3K)/3ʹ-phosphoinositide-dependent kinase-1 (PDK1). Phosphorylation of BAD (a BH3-only pro-apoptotic protein) by Akt or protein kinase A (PKA) leads to BAD inactivation and promotion of cell survival (for review see: [Citation76]). By activating PKC-α-mTORC2 and PI3K-PDK1 signaling pathways, SARS-Cov-2 could induce the mTORC1-dependent protein synthesis and Akt-dependent pro-survival machinery, thus accelerating its multiplication. The majority of these processes could be inhibited by chelerythrine ().
Figure 1. Proposed mechanism of SARS-CoV-2 action and its inhibition by chelerythrine. mTORC2-dependent, mTORC1-mediated protein synthesis as well as mTORC2/PI3K-PDK1-dependent Akt activation and the resulting promotion of cell survival is the basic prerequisite for the synthesis of viral proteins and replication of its genome by the host cell biosynthetic machinery . Therefore, the inhibition of various enzymes of the host cell involved in virus production is an adequate means to stop these processes. Chelerythrine as a specific inhibitor of the protein kinases C alpha and beta (PKC-α/-β) can play an elementary role to accomplish this task. Furthermore, chelerythrine could directly inhibit the upstream kinase of the RNA polymerase of SARS-CoV-2, thus causing its inactivation
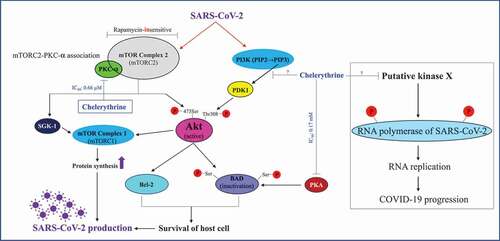
Conclusions
The remarkable properties of chelerythrine: (a) fast diffusion across the cell membrane [Citation77], (b) asymmetric distribution between cytosol and membrane particulate fractions [Citation78], (c) preferential inhibition of both conventional PKC-α and -β (d) causing a pro-apoptotic effect in nucleated cells and thus creation of a hostile environment for intracellular parasites including viruses, (e) creating a pro-survival effect in enucleated human erythrocytes and thus enhancing the anti-viral and -bacterial activities of these cells, (f) its curative effect against viral and bacterial infection-associated anemia [Citation79], and (g) its capability to interact with divers DNA and RNA confirmations, allows the legitimate hypothesis that chelerythrine is predestined for COVID-19 treatment.
Authors contributions
MG designed the project and wrote the manuscript. All figures were made by MG and MK. All authors read, discussed, improved and approved the final version of the manuscript.
Disclosure statement
The authors declare that no competing financial interests or otherwise exist.
Additional information
Funding
References
- Scheeren FA, Nagasawa M, Weijer K, et al. T cell-independent development and induction of somatic hypermutation in human IgM+ IgD+ CD27+ B cells. J Exp Med. 2008;205:2033–2042.
- Klein U, Goossens T, Fischer M, et al. Somatic hypermutation in normal and transformed human B cells. Immunol Rev. 1998;162:261–280.
- Li Z, Woo CJ, Iglesias-Ussel MD, et al. The generation of antibody diversity through somatic hypermutation and class switch recombination. Genes Dev. 2004;18:1–11.
- Lee GS, Brandt VL, Roth DB. B cell development leads off with a base hit: dU:dG mismatches in class switching and hypermutation. Mol Cell. 2004;16:505–508.
- Steinhauer DA, Holland JJ. Rapid evolution of RNA viruses. Annu Rev Microbiol. 1987;41:409–433.
- Kim D, Lee JY, Yang JS, et al. The architecture of SARS-CoV-2 transcriptome. Cell. 2020;181:914–21 e10.
- Solinas C, Perra L, Aiello M, et al. A critical evaluation of glucocorticoids in the management of severe COVID-19. Cytokine Growth Factor Rev. 2020;54:8–23.
- Ferner RE, Aronson JK. Chloroquine and hydroxychloroquine in covid-19. BMJ. 2020;369:m1432.
- Bonsch C, Kempf C, Mueller I, et al. Chloroquine and its derivatives exacerbate B19V-associated anemia by promoting viral replication. PLoS Negl Trop Dis. 2010;4:e669.
- Theoharides TC, Conti P. Dexamethasone for COVID-19? Not so fast. J Biol Regul Homeost Agents. 2020;34:1241-1243.
- Paccaud JP, Carpentier JL, Schifferli JA. Difference in the clustering of complement receptor type 1 (CR1) on polymorphonuclear leukocytes and erythrocytes: effect on immune adherence. Eur J Immunol. 1990;20:283–289.
- Craig ML, Bankovich AJ, Taylor RP. Visualization of the transfer reaction: tracking immune complexes from erythrocyte complement receptor 1 to macrophages. Clin Immunol. 2002;105:36–47.
- Hanel P, Andreani P, Graler MH. Erythrocytes store and release sphingosine 1-phosphate in blood. Faseb J. 2007;21:1202–1209.
- Matloubian M, Lo CG, Cinamon G, et al. Lymphocyte egress from thymus and peripheral lymphoid organs is dependent on S1P receptor 1. Nature. 2004;427:355–360.
- Garcia JG, Liu F, Verin AD, et al. Sphingosine 1-phosphate promotes endothelial cell barrier integrity by Edg-dependent cytoskeletal rearrangement. J Clin Invest. 2001;108:689–701.
- Jo SK, Bajwa A, Awad AS, et al. Sphingosine-1-phosphate receptors: biology and therapeutic potential in kidney disease. Kidney Int. 2008;73:1220–1230.
- Schwalm S, Pfeilschifter J, Huwiler A. Targeting the sphingosine kinase/sphingosine 1-phosphate pathway to treat chronic inflammatory kidney diseases. Basic Clin Pharmacol Toxicol. 2014;114:44–49.
- Hu Y, Li M, Gothert JR, et al. Hemovascular progenitors in the kidney require sphingosine-1-phosphate receptor 1 for vascular development. J Am Soc Nephrol. 2016;27:1984–1995.
- Wissbrock A, Goradia NB, Kumar A, et al. Structural insights into heme binding to IL-36alpha proinflammatory cytokine. Sci Rep. 2019;9:16893.
- Igarashi J, Murase M, Iizuka A, et al. Elucidation of the heme binding site of heme-regulated eukaryotic initiation factor 2alpha kinase and the role of the regulatory motif in heme sensing by spectroscopic and catalytic studies of mutant proteins. J Biol Chem. 2008;283:18782–18791.
- Schakel K, von Kietzell M, Hansel A, et al. Human 6-sulfo LacNAc-expressing dendritic cells are principal producers of early interleukin-12 and are controlled by erythrocytes. Immunity. 2006;24:767–777.
- Horuk R, Colby TJ, Darbonne WC, et al. The human erythrocyte inflammatory peptide (chemokine) receptor. Biochemical characterization, solubilization, and development of a binding assay for the soluble receptor. Biochemistry. 1993;32:5733–5738.
- de Winter RJ, Manten A, de Jong YP, et al. Interleukin 8 released after acute myocardial infarction is mainly bound to erythrocytes. Heart. 1997;78:598–602.
- Ragab D, Salah Eldin H, Taeimah M, et al. The COVID-19 cytokine storm; what we know so far. Front Immunol. 2020;11:1446.
- Maharaj NP, Wies E, Stoll A, et al. Conventional protein kinase C-alpha (PKC-alpha) and PKC-beta negatively regulate RIG-I antiviral signal transduction. J Virol. 2012;86:1358–1371.
- Chen F, Kumar S, Yu Y, et al. PKC-dependent phosphorylation of eNOS at T495 regulates eNOS coupling and endothelial barrier function in response to G+ -toxins. PLoS One. 2014;9:e99823.
- Marjuki H, Alam MI, Ehrhardt C, et al. Membrane accumulation of influenza A virus hemagglutinin triggers nuclear export of the viral genome via protein kinase Calpha-mediated activation of ERK signaling. J Biol Chem. 2006;281:16707–16715.
- Rossman JS, Lamb RA. Influenza virus assembly and budding. Virology. 2011;411:229–236.
- Tooze J, Tooze S, Warren G. Replication of coronavirus MHV-A59 in sac- cells: determination of the first site of budding of progeny virions. Eur J Cell Biol. 1984;33:281–293.
- Wu KE, Fazal FM, Parker KR, et al. RNA-GPS predicts SARS-CoV-2 RNA residency to host mitochondria and nucleolus. Cell Syst. 2020;11:102–8 e3.
- Harrison AG, Lin T, Wang P. Mechanisms of SARS-CoV-2 transmission and pathogenesis. Trends Immunol. 2020;41(12):1100–1115.
- Xia H, Cao Z, Xie X, et al. Evasion of type I interferon by SARS-CoV-2. Cell Rep. 2020;33:108234.
- Herbert JM, Augereau JM, Gleye J, et al. Chelerythrine is a potent and specific inhibitor of protein kinase C. Biochem Biophys Res Commun. 1990;172:993–999.
- Andrews DA, Yang L, Low PS. Phorbol ester stimulates a protein kinase C-mediated agatoxin-TK-sensitive calcium permeability pathway in human red blood cells. Blood. 2002;100:3392–3399.
- Chmura SJ, Dolan ME, Cha A, et al. In vitro and in vivo activity of protein kinase C inhibitor chelerythrine chloride induces tumor cell toxicity and growth delay in vivo. Clin Cancer Res. 2000;6:737–742.
- Shi B, Li S, Ju H, et al. Protein kinase C inhibitor chelerythrine attenuates partial unilateral ureteral obstruction induced kidney injury in neonatal rats. Life Sci. 2019;216:85–91.
- Ghashghaeinia M, Koralkova P, Giustarini D, et al. The specific PKC-alpha inhibitor chelerythrine blunts costunolide-induced eryptosis. Apoptosis. 2020;25:674–685.
- Jarvis WD, Turner AJ, Povirk LF, et al. Induction of apoptotic DNA fragmentation and cell death in HL-60 human promyelocytic leukemia cells by pharmacological inhibitors of protein kinase C. Cancer Res. 1994;54:1707–1714.
- Wan KF, Chan SL, Sukumaran SK, et al. Chelerythrine induces apoptosis through a Bax/Bak-independent mitochondrial mechanism. J Biol Chem. 2008;283:8423–8433.
- Allam M, Bertrand R, Zhang-Sun G, et al. Cholera toxin triggers apoptosis in human lung cancer cell lines. Cancer Res. 1997;57:2615–2618.
- Parlakpinar H, Tasdemir S, Polat A, et al. Protective effect of chelerythrine on gentamicin-induced nephrotoxicity. Cell Biochem Funct. 2006;24:41–48.
- He N, Wang P, Wang P, et al. Antibacterial mechanism of chelerythrine isolated from root of Toddalia asiatica (Linn) Lam. BMC Complement Altern Med. 2018;18:261.
- Li J, Li B, Wu Y, et al. Luminescence and binding properties of two isoquinoline alkaloids chelerythrine and sanguinarine with ctDNA. Spectrochim Acta A Mol Biomol Spectrosc. 2012;95:80–85.
- Bai LP, Hagihara M, Nakatani K, et al. Recognition of chelerythrine to human telomeric DNA and RNA G-quadruplexes. Sci Rep. 2014;4:6767.
- Bai LP, Zhao ZZ, Cai Z, et al. Site-specific binding of chelerythrine to single cytosine and thymine bulges in DNA hairpins. Nucleic Acids Symp Ser (Oxf). 2006;50:197–198.
- Terenzi A, Gattuso H, Spinello A, et al. Targeting G-quadruplexes with organic dyes: chelerythrine-DNA binding elucidated by combining molecular modeling and optical spectroscopy. Antioxidants (Basel). 2019;8:472.
- Jana J, Mondal S, Bhattacharjee P, et al. Chelerythrine down regulates expression of VEGFA, BCL2 and KRAS by arresting G-Quadruplex structures at their promoter regions. Sci Rep. 2017;7:40706.
- Basu P, Payghan PV, Ghoshal N, et al. Structural and thermodynamic analysis of the binding of tRNA(phe) by the putative anticancer alkaloid chelerythrine: spectroscopy, calorimetry and molecular docking studies. J Photochem Photobiol B. 2016;161:335–344.
- Agarwala P, Pandey S, Maiti S. The tale of RNA G-quadruplex. Org Biomol Chem. 2015;13:5570–5585.
- Hognon C, Miclot T, Garci AIC, et al. Role of RNA guanine quadruplexes in favoring the dimerization of SARS unique domain in coronaviruses. J Phys Chem Lett. 2020;11:5661–5667.
- Kato H, Takeuchi O, Mikamo-Satoh E, et al. Length-dependent recognition of double-stranded ribonucleic acids by retinoic acid-inducible gene-I and melanoma differentiation-associated gene 5. J Exp Med. 2008;205:1601–1610.
- Pichlmair A, Schulz O, Tan CP, et al. RIG-I-mediated antiviral responses to single-stranded RNA bearing 5ʹ-phosphates. Science. 2006;314:997–1001.
- Kim SJ, Kim JH, Kim YG, et al. Protein kinase C-related kinase 2 regulates hepatitis C virus RNA polymerase function by phosphorylation. J Biol Chem. 2004;279:50031–50041.
- Fonseca AM, Pereira CF, Porto G, et al. Red blood cells promote survival and cell cycle progression of human peripheral blood T cells independently of CD58/LFA-3 and heme compounds. Cell Immunol. 2003;224:17–28.
- Porto B, Fonseca AM, Godinho I, et al. Human red blood cells have an enhancing effect on the relative expansion of CD8+ T lymphocytes in vitro. Cell Prolif. 2001;34:359–367.
- Beck Z, Brown BK, Wieczorek L, et al. Human erythrocytes selectively bind and enrich infectious HIV-1 virions. PLoS One. 2009;4:e8297.
- Morera D, MacKenzie SA. Is there a direct role for erythrocytes in the immune response? Vet Res. 2011;42:89.
- Umeda M, Nojima S, Inoue K. Activity of human erythrocyte gangliosides as a receptor to HVJ. Virology. 1984;133:172–182.
- Paul RW, Lee PW. Glycophorin is the reovirus receptor on human erythrocytes. Virology. 1987;159:94–101.
- Enegren BJ, Burness AT. Chemical structure of attachment sites for viruses on human erythrocytes. Nature. 1977;268:536–537.
- Eaton BT, Crameri GS. The site of bluetongue virus attachment to glycophorins from a number of animal erythrocytes. J Gen Virol. 1989;70(Pt 12):3347–3353.
- Nishimura H, Sugawara K, Kitame F, et al. Attachment of influenza C virus to human erythrocytes. J Gen Virol. 1988;69(Pt 10):2545–2553.
- Ruvoen-Clouet N, Blanchard D, Andre-Fontaine G, et al. Partial characterization of the human erythrocyte receptor for rabbit haemorrhagic disease virus. Res Virol. 1995;146:33–41.
- Costafreda MI, Ribes E, Franch A, et al. A single mutation in the glycophorin A binding site of hepatitis A virus enhances virus clearance from the blood and results in a lower fitness variant. J Virol. 2012;86:7887–7895.
- Angel MA, Burness AT. The attachment of encephalomyocarditis virus to erythrocytes from several animal species. Virology. 1977;83:428–432.
- Allaway GP, Burness AT. Site of attachment of encephalomyocarditis virus on human erythrocytes. J Virol. 1986;59:768–770.
- Li J, Wang JP, Ghiran I, et al. Complement receptor 1 expression on mouse erythrocytes mediates clearance of Streptococcus pneumoniae by immune adherence. Infect Immun. 2010;78:3129–3135.
- Thielen AJF, Zeerleder S, Wouters D. Consequences of dysregulated complement regulators on red blood cells. Blood Rev. 2018;32:280–288.
- Carlisle RC, Di Y, Cerny AM, et al. Human erythrocytes bind and inactivate type 5 adenovirus by presenting Coxsackie virus-adenovirus receptor and complement receptor 1. Blood. 2009;113:1909–1918.
- Miyaike J, Iwasaki Y, Takahashi A, et al. Regulation of circulating immune complexes by complement receptor type 1 on erythrocytes in chronic viral liver diseases. Gut. 2002;51:591–596.
- Wong CH, Jenne CN, Petri B, et al. Nucleation of platelets with blood-borne pathogens on Kupffer cells precedes other innate immunity and contributes to bacterial clearance. Nat Immunol. 2013;14:785–792.
- Broadley SP, Plaumann A, Coletti R, et al. Dual-track clearance of circulating bacteria balances rapid restoration of blood sterility with induction of adaptive immunity. Cell Host Microbe. 2016;20:36–48.
- Llorente C, Schnabl B. Fast-track clearance of bacteria from the liver. Cell Host Microbe. 2016;20:1–2.
- Medina-Jover F, Gendrau-Sanclemente N, Vinals F. SGK1 is a signalling hub that controls protein synthesis and proliferation in endothelial cells. FEBS Lett. 2020;594:3200–3215.
- Partovian C, Ju R, Zhuang ZW, et al. Syndecan-4 regulates subcellular localization of mTOR Complex2 and Akt activation in a PKCalpha-dependent manner in endothelial cells. Mol Cell. 2008;32:140–149.
- Downward J. How BAD phosphorylation is good for survival. Nat Cell Biol. 1999;1:E33–5.
- Slaninova I, Slanina J, Taborska E. Quaternary benzo[c]phenanthridine alkaloids–novel cell permeant and red fluorescing DNA probes. Cytometry A. 2007;71:700–708.
- Dorney KM, Sizemore IE, Alqahtani T, et al. Surface-enhanced Raman spectroscopy (SERS) tracking of chelerythrine, a Na(+)/K(+) pump inhibitor, into cytosol and plasma membrane fractions of human lens epithelial cell cultures. Cell Physiol Biochem. 2013;32:146–156.
- Cheng T. Remedy for anemia and arthritis. Patent U, ed. 1986.