ABSTRACT
Skeletal muscle development is a multistep biological process regulated by a variety of myogenic regulatory factors, including MyoG, MyoD, Myf5, and Myf6 (also known as MRF4), as well as members of the FoxO subfamily. Differentiation and regeneration during skeletal muscle myogenesis contribute to the physiological function of muscles. Super enhancers (SEs) and enhancer RNAs (eRNAs) are involved in the regulation of development and diseases. Few studies have identified the roles of SEs and eRNAs in muscle development and pathophysiology. To develop approaches to enhance skeletal muscle mass and function, a more comprehensive understanding of the key processes underlying muscular diseases is needed. In this review, we summarize the roles of SEs and eRNAs in muscle development and disease through affecting of DNA methylation, FoxO subfamily, RAS-MEK signaling, chromatin modifications and accessibility, MyoD and cis regulating target genes. The summary could inform strategies to increase muscle mass and treat muscle-related diseases.
1. Introduction
Skeletal muscle is important for metabolism [Citation1,Citation2] and movement [Citation3–5] and is the major contributor to body mass. In some pathological conditions, such as cancer cachexia [Citation6,Citation7], heart failure [Citation8,Citation9], Duchenne muscular dystrophy [Citation10,Citation11], burn injury [Citation12,Citation13], and rhabdomyosarcoma (RMS) [Citation14,Citation15], patients show muscle disorders. Skeletal muscle regeneration is a physiological process relying on the adult muscle stem cells (MuSCs) which are generally in a quiescent state. Following a muscle injury or disorders, MuSCs are activated, enter the cell cycle to proliferate, migrate to the impaired areas, enter the myogenic differentiation program differentiate, and eventually give rise to fusion forming newly myofibers [Citation16]. The regulation of forming new myofibers from MuSCs during skeletal muscle development relies on a series of specific transcription factors. Pax3 and its paralog, Pax7, are considered to be markers of MuSCs, which support an essential role in the normal function of satellite cells in adult skeletal muscle. Pax3 is necessary for maintaining the migration of the cells in the somite and their migration to the myogenic sites [Citation17]. Knockdown or deletion of Pax7 results in growth arrest [Citation18,Citation19] and significantly impaired regeneration as indicated by substantial deposition of fibrotic and decreased formation of myofibers [Citation20]. Myogenic regulatory factors (MRFs), including MyoG, MyoD, Myf5, and Myf6 (also known as MRF4) are well-known regulators of muscle development. MyoG plays a vital role in myogenesis and homeostasis [Citation21]. MyoD and Myf5 primarily participate in muscle specification and trigger the transformation of non-muscle cells into muscle cells, such as fibroblasts and chondroblasts [Citation22,Citation23]. MyoG and Myf6 function later in myogenesis, promoting the formation and maturation of myotubes [Citation24,Citation25]. Mice with mutations of the MyoG gene survive fetal development, but die immediately after birth due to failure to develop normal muscle mass [Citation26]. Four members of the FoxO subfamily, FoxO1, FoxO3a, FoxO4, and FoxO6, are involved in diseases, such as diabetes [Citation27], alveolar RMS [Citation28], senescence [Citation29], and cancer [Citation30,Citation31].
Next-generation sequencing technologies have facilitated comprehensive analysis of coding sequences and led to the discovery of numerous regulatory elements that do not act as templates for coding proteins [Citation32]. In recent years, several studies have indicated that non-coding RNAs (ncRNAs), such as microRNAs (miRNAs), long ncRNAs (lncRNAs), and circular RNAs (circRNAs), play vital roles in skeletal muscle development and disease, by acting as competitive endogenous RNA [Citation33,Citation34], regulating transcription factors, and participating in the epigenetic control of DNA methylation or chromatin activity [Citation35–37]. Two systematic reviews by our groups summarized the known functions and mechanisms of lncRNAs and circRNAs in skeletal muscle development, as well the roles of miRNAs and lncRNAs in regulating the skeletal muscle atrophy seen in cachexia [Citation38,Citation39].
Among non-coding regulatory elements, super enhancers (SEs) were discovered at a relatively late stage. Enhancers, which were first shown to enhance the transcription of β-globin in rabbits in 1981 [Citation40], are short regulatory elements in DNA that help activate the transcription of target genes remotely from gene locus. Whyte found that the master transcription factors of embryonic stem cells could bind special enhancers essential for maintaining the pluripotent state in 2013; these special enhancers were defined as SEs [Citation41]. Compared with typical enhancers, SEs, which occur in large numbers of enhancer clusters, exhibit remarkably increased occupancy of transcription factors and RNAP II, enhance hypersensitivity to DNase I, and promote higher levels of H3K4me1 and H3K27ac density, as well as cohesin, Brd4, mediators, and p300/CBP occupancy [Citation41–43]. High binding levels of the master transcription factors OCT4 and SOX2 are seen in enhancers near genes that determine embryonic stem cell identity [Citation41]. Super and typical enhancers can be identified and distinguished from each other by sequencing nascent RNA, similar to traditional ChIP-seq [Citation44]. In 2010, enhancer regions were found to actively transcribed [Citation45,Citation46]. The product of this transcription was termed enhancer RNA (eRNA). The production of eRNA requires three steps: recruitment of necessary transcription factors and coactivators; histone modifications at enhancer loci; and transcription, elongation, and processing of eRNA [Citation47]. An increasing number of studies have suggested various roles for SEs and eRNAs in regulating multiple aspects of cell function in cancer [Citation48,Citation49], metabolic [Citation50,Citation51], immune diseases [Citation52,Citation53], Huntington disease [Citation54] and Alzheimer [Citation55], as well as skeletal muscle development and RMS which is a soft tissue tumor linked with the skeletal muscle lineage generally occurring in childhood and adolescence [Citation56,Citation57].
The discovery of SEs and eRNAs paved the way for modern cellular genomics, although their roles in regulating skeletal muscle development and pathophysiology remain unclear. In this review, we summarize the SEs and eRNAs that regulate skeletal muscle development according to recent reports. A better understanding of the functional mechanisms of SEs and eRNAs will aid efforts to increase muscle mass and could reveal potential therapeutic targets for muscle-related diseases.
2. Roles of SEs in skeletal muscle development and disease
Although the roles of coding sequences in muscle development have been extensively investigated, the key processes underlying the non-coding sequences driving muscle development and disease require further clarification. Recent reports have shown that SEs play roles in skeletal muscle development () and disease () via different mechanisms.
Figure 1. Schematic showing the role of SEs in skeletal muscle development. the various SEs that regulate myoblast differentiation are in green font.
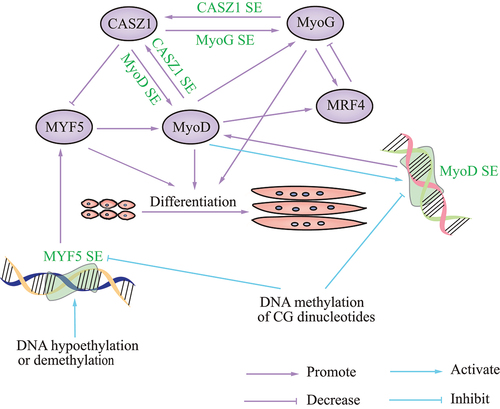
Figure 2. Schematic showing the role of SEs in skeletal muscle disease. the various SEs that regulate disease are in green font.
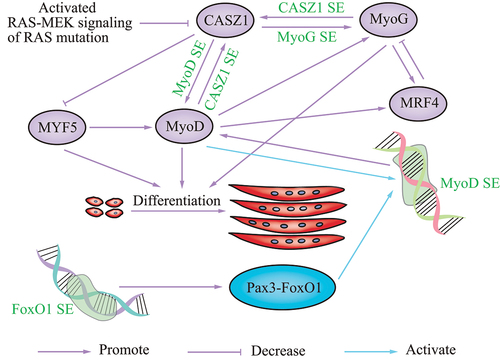
SE activation can be affected by DNA hypomethylation, demethylation, and methylation. The main epigenetic modifications are histone and DNA modifications, which play an important role in the development of skeletal muscle. Preferential gene expression in skeletal muscle was associated with overlapping foci of DNA hypomethylation, according to bioinformatic analysis of 44 muscle-associated genes; half of these genes have skeletal muscle-specific SEs [Citation58], consistent with a study revealing that tissue-specific regulatory genes of skeletal muscle are highly enriched in SEs [Citation59]. A 0.258-kb distal enhancer of MyoD is completely unmethylated at all the CG sites tested in myogenic cells and a subpopulation of somite cells [Citation60]. Recently, transfection assays confirmed the tissue specificity of the 0.3-kb core enhancer (CE) within the SE of MyoD in C2C12, and demonstrated its repression by methylation of three CG dinucleotides. DNA hypomethylation has been shown to increase enhancer tissue-specificity [Citation58], while methylation of three CG nucleotide pairs in the MyoD CE strongly repressed its activity [Citation58]. Myogenic stem cells display enhanced DNA methylation, while muscle differentiation was associated with a loss of DNA methylation in CG-poor regions. DNA methylation could prevent the deleterious activation of Myf5 [Citation61]. Activation of the Myf5 SE was driven by DNA demethylation exclusively in muscle-committed cells, resulting in gene expression. Furthermore, upstream stimulatory factor 1 (Usf1) binding to the Myf5 SE occurs upon DNA demethylation in myogenic stem cells [Citation61]. These results emphasize the function of DNA demethylation in lineage-specific activation of the muscle-identity gene Myf5 SE, in combination with the transcription factor Usf1, during cellular development [Citation61]. Studies of epigenetic modifications via SEs during skeletal muscle development are needed.
SEs exert effects in myogenesis or RMS via members of the FoxO subfamily. In a recent study, SEs were uncovered to gradually remodel during myogenic differentiation by ChIP-seq analysis of histone marks; SE hotspots are co-bound by master transcription factors and MyoD. In-depth dissection showed that MyoD/FoxO3 interaction drove hotspot formation and SE activation [Citation62]. In addition to FoxO3, FoxO1 also affects SE activity. In a cancer of muscle lineage, fusion-positive RMS, a translocated FoxO1 SE, drives the oncogene PAX3-FoxO1 in the late stage of myogenesis. Disruption of FoxO1 SE reduced PAX3-FoxO1 at the transcript and protein levels. PAX3 activates MyoD by binding and activating MyoD SEs [Citation63], and several FoxO1 cis-regulatory domains interact with PAX3. HiChIP of H3K27ac revealed connections among the PAX3 promoter, additional intra-domain enhancers, and FoxO1 SE [Citation63]. PAX3-FoxO1 can also reprogram the cis-regulatory landscape by eliciting SEs; PAX3-FoxO1 established auto-regulatory circuitry with transcription factors MyoG and MyoD via SEs. In cell lines and primary tumors, this myogenic SE loop is consistently seen [Citation64]. These studies suggest a vital role of SEs in myogenesis and RMS.
SEs also play a role in muscle-related diseases by modulating RAS-MEK signaling. RMS is a common muscle-related disease arising from myoblasts, muscle stem cells, and activated satellite cells, as indicated by both in vivo and in vitro studies [Citation58,Citation61,Citation62,Citation65]. Ras-Raf-MEK-ERK-MAPK (RAS-MEK) is a signaling cascade that blocks myogenic differentiation [Citation61,Citation65]. In a recent report, a SE was found on the CASZ1 gene locus that occurs in human skeletal muscle myotubes but not myoblasts, which suggests that the increased expression of CASZ1 was driven by a SE during human skeletal myogenesis. Loss of CASZ1 in normal muscle cells led to a decrease in SE signaling at MyoD and MyoG [Citation56]. CASZ1 induces differentiation of skeletal muscle and PAX-FoxO1 fusion-negative embryonal RMS (ERMS) by forming a feedforward loop with MyoG and MyoD. Overexpression of CASZ1 decreased Myf5 mRNA levels, and increased MyoD and MyoG mRNA levels. Analysis of the ChIP-seq data suggested that, in C2C12 myotubes, both MyoD and MyoG bind the promoter and enhancer regions of CASZ1. Silencing of MyoD or MyoG, but not Myf5, in C2C12 cells decreased CASZ1 expression, indicating MyoD and MyoG directly regulate CASZ1 transcription [Citation56]. CASZ1 is inhibited by activated RAS-MEK signaling in ERMS with a RAS mutation [Citation56]. These data indicate that restoration of CASZ1 in ERMS establishes new SEs. Another report showed that MEK inhibition can induce MyoG via the RAS-MEK effector pathway. MyoG subsequently opens chromatin and establishes SEs in genes required for late myogenic differentiation in RAS-driven RMS [Citation65], suggesting that SEs function in RMS via RAS-MEK signaling.
3. eRNAs in skeletal muscle development
Enhancer-mediated regulation of various biological pathways and diseases has been extensively investigated [Citation66–68]. eRNAs, as products transcribed from enhancers or SEs, have only recently been investigated; nevertheless, they have attracted attention in terms of their functions in skeletal muscle development and disease ().
Figure 3. Schematic showing the role of eRNAs in skeletal muscle development. the various eRNAs that regulate the differentiation and regeneration of myoblasts are in green font.
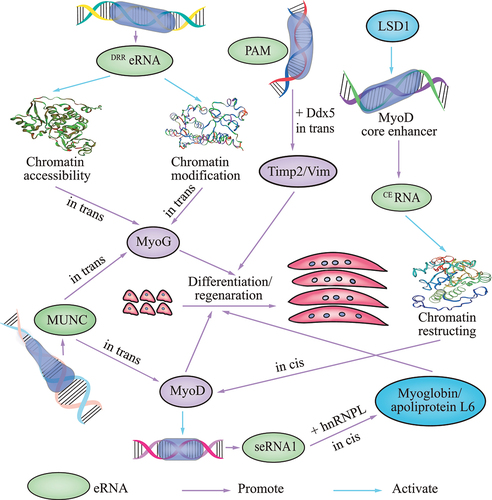
Emerging evidence supports regulatory roles of eRNAs in chromatin modifications and accessibility during muscle cell differentiation [Citation69,Citation70]. Two enhancers, namely the CE and the distal regulatory region (DRR), can regulate the expression of MyoD [Citation71–73]. The CE enhancer directs gene expression in somitic precursors during embryogenesis and the DRR enhancer does so in early differentiated myogenic cells [Citation74]. The DRReRNAs and CEeRNAs are transcribed from the enhancer regions of the master regulator MyoD. CEeRNA facilitates chromatin restructuring in MyoD, resulting in polymerase II (Pol II) recruitment and expression. Upon MyoD gene activation and during differentiation, DRReRNA promotes the chromatin modifications at MyoG necessary for its expression, resulting in a feedforward loop for the myogenic program [Citation70]. Thus, eRNAs direct gene activation by modulating chromatin remodeling at distinct regulatory genomic regions. DRReRNA is also recruited to the MyoG locus, where it colocalizes with MyoG nascent transcripts [Citation69]. DRReRNA associates with the cohesin complex; depletion of cohesin or DRReRNA can decrease chromatin accessibility, thereby blocking MyoG activation and preventing muscle cell differentiation [Citation69]. Thus, DRReRNA ensures spatially appropriate cohesin loading in trans, and regulates the expression of genes linked to muscle cell differentiation by decreasing chromatin accessibility. CEeRNA promotes chromatin restructuring in MyoD, resulting in Pol II recruitment and the expression, and regulation of transcription of the adjacent MyoD gene in cis [Citation70,Citation75].
MyoD plays a significant role in skeletal muscle development. eRNAs function in muscle development via regulation of MyoD. Lysine-specific demethylase 1 (LSD1) is a monoamine oxidase that can demethylate mono- or dimethylated lysine 4 and 9 of the histone H3 N-terminus (H3K4me1, H3K4me2 and H3K9me1, H3K9me2), thus promoting transcriptional repression or activation [Citation76–79]. LSD1 is recruited to the MyoD CE, where it facilitates transcription of an eRNA that controls the timing of MyoD expression during myoblast commitment [Citation75]. In myoblasts, depletion of lysine-specific LSD1 prevents recruitment of RNA Pol II and removal of H3K9 methylation on the CE, thereby precluding transcription of the non-coding eRNAs (CEeRNAs) required for MyoD expression; this has been shown to occur in muscle progenitor cells during embryogenesis [Citation75]. The effects of LSD1 inactivation can be efficiently reversed by the expression of the CEeRNA (−strand). MUNC, which is situated in the DRR enhancer of MyoD and formally classified as an eRNA (DRR eRNA), is required for optimal myogenic differentiation, and induces specific myogenic transcripts, such as MyoD and MyoG, in trans [Citation80]. Although MUNC is an eRNA of MyoD, it is also a trans-acting lncRNA whose sequence, structure, and co-operating factors include, but are not limited, to MyoD; as a lncRNA, MUNC regulates many myogenic genes [Citation80,Citation81]. Another seRNA PAM (Pax7 Associated Muscle lncRNA) which generated from a myoblast-specific SE also acts in trans, but without affecting MyoD. Timp2 is known to delay myogenic differentiation in C2C12 myoblasts [Citation82]. Vim is an intermediate filament protein, which is also considered as a reliable marker for regenerating muscle tissue [Citation83]. Studies indicate that seRNA PAM interacts with Ddx5 to tether PAM SE to its inter-chromosomal targets Timp2 and Vim to activate the gene expression, so as to promote satellite cell and C2C12 myoblast proliferation and regeneration [Citation84].
In addition to MyoD, eRNAs regulate other target genes in cis. The SE-generated eRNAs seRNA-1 and seRNA-2 facilitate myogenic differentiation in vitro and in vivo. The master transcription factor MyoD is crucial for activating eRNA production. Knockdown of MyoD decreased myotube seRNAs generated from SEs, supporting a role of MyoD in seRNA induction during differentiation [Citation57]. seRNA may bind and tether heterogeneous nuclear ribonucleoprotein L (hnRNPL) to the promoter of target myoglobin and apolipoprotein L6, to enhance transcription in cis by increasing the local concentration of Pol II or H3K36me3 [Citation57]. When evaluating expression dynamics in vivo during injury-induced muscle regeneration, myoglobin and apolipoprotein L6 were highly expressed in homeostatic muscles, although this was markedly reduced after muscle injury, and continually elevated during regeneration [Citation57], thereby supporting their role in promoting muscle regeneration. Disruption of the seRNA-1-hnRNPL interaction attenuates Pol II and H3K36me3 deposition at the myoglobin locus, thus reducing its transcription. Furthermore, analyses of hnRNPL binding transcriptome-wide have revealed that its association with eRNAs is a general phenomenon [Citation57]. The eRNA–hnRNPL interaction may contribute to target mRNA activation.
The epigenomic reader Brd4 is also involved in regulating the production of eRNAs during cell differentiation and development. Brd4 is a member of the BET family; evidence from tissue-specific Brd4 knockout mice and derived cells indicates that Brd4 controls cell identity gene induction and is essential for myogenesis [Citation85]. Brd4 colocalizes with lineage-determining transcription factors on active enhancers during differentiation. Deletion of Brd4 decreases eRNA production from Brd4+ adipogenic enhancers, cell identity gene induction, and cell differentiation [Citation85]. The small-molecule competitive BET inhibitor JQ1 inhibits Brd4 binding, leading to displacement of the mediator complex and Pol II from enhancers, which in turn suppresses eRNA production and associated gene expression [Citation86–88]. These discoveries suggest Brd4 is an enhancer epigenomic reader that mediates the relationship between eRNA and cell identity gene induction during differentiation.
4. Concluding remarks
Muscle development is a complex biological process strictly regulated by various pathways that also play a major role in related diseases. Dysregulation of these pathways is mediated by genetic and epigenetic alterations of protein coding genes and non-coding regulatory elements, which have been shown to function in myogenesis and related diseases.
Targeting disease-specific SEs may be a useful approach for identifying candidate biomarkers and therapeutic targets. Brd4, a member of the BET family, is essential for myogenesis. Brd4 inhibitors are promising candidate drugs for treating SE-related diseases, such as multiple myeloma and lymphoma [Citation89,Citation90]; the pharmacological efficacy and safety of these inhibitors are currently being evaluated in phase I clinical trials. Multiple myeloma cells were the first cells used to demonstrate how BET inhibitors can abolish SEs [Citation86]. Young and Bradner, as pioneering researchers of SEs [Citation86,Citation91,Citation92], collaborated with Syros Pharmaceuticals (SYRS) to research and develop SE-related targets for cancer treatment. We expect that further SE research will provide an in-depth understanding of molecular pathogenesis and progression, and aid the development of novel therapies to treat skeletal muscle disease.
A broad range of disease-associated mutations occur outside the coding genome; multiple genome-wide association studies have indicated that many of these mutations occur in enhancers or their transcribed RNAs [Citation93,Citation94]. eRNAs are useful for “mapping” anxiety disorders [Citation95], cardiac hypertrophy [Citation96], lymphoblast proliferation [Citation97], neurological disorders [Citation98], recurrent pregnancy loss [Citation99,Citation100], and cancer [Citation101–104]. The inhibition of hypoxia-inducible enhancer RNA (HERNA) 1 blocked stress-induced cardiac pathogenesis and significantly improved the overall survival of diseased mice, thereby demonstrating the therapeutic potential of eRNAs [Citation96]. Although therapeutic applications are still in their infancy, drugs affecting eRNAs directly or indirectly will likely be useful for treating cancer and muscle-related diseases in the future.
Skeletal muscle development is a complex progress regulated by various MRFs, which constitute the core of the transcriptional cascades that induce myogenesis and muscle phenotypes. Next-generation sequencing has led to the discovery of numerous non-coding regulatory elements, which play significant roles in skeletal muscle development and disease via different mechanisms. Research on preventing or treating muscle-related diseases, among other diseases, through targeting SEs and eRNAs is still in its infancy. Nevertheless, drugs targeting SEs and eRNAs may emerge as important options for treating muscle-related diseases.
Disclosure statement
No potential conflict of interest was reported by the author(s).
Data availability statement
Data sharing not applicable – no new data generated.
Additional information
Funding
References
- Koh JH, Pataky MW, Dasari S, et al. Enhancement of anaerobic glycolysis - a role of PGC-1alpha4 in resistance exercise. Nat Commun. 2022;13(1):2324.
- Agudelo LZ, Ferreira D, Dadvar S, et al. Skeletal muscle PGC-1alpha1 reroutes kynurenine metabolism to increase energy efficiency and fatigue-resistance. Nat Commun. 2019;10(1):2767.
- Murach KA, Dimet-Wiley AL, Wen Y, et al. Late-Life exercise mitigates skeletal muscle epigenetic aging. Aging Cell. 2022;21(1):e13527.
- Fiorenza M, Lemminger AK, Marker M, et al. High-Intensity exercise training enhances mitochondrial oxidative phosphorylation efficiency in a temperature-dependent manner in human skeletal muscle: implications for exercise performance. FASEB J. 2019;33(8):8976–8989.
- Devarshi PP, Pereyra AS, Ellis JM, et al. A single bout of cycling exercise induces nucleosome repositioning in the skeletal muscle of lean and overweight/obese individuals. Diabetes Obes Metab. 2022;24(1):21–33.
- Wyart E, Hsu MY, Sartori R, et al. Iron supplementation is sufficient to rescue skeletal muscle mass and function in cancer cachexia. EMBO Rep. 2022;23(4):e53746.
- Luan Y, Zhang Y, Yu SY, et al. Development of ovarian tumour causes significant loss of muscle and adipose tissue: a novel mouse model for cancer cachexia study. J Cachexia Sarcopenia Muscle. 2022;13(2):1289–1301.
- Konishi M, Akiyama E, Matsuzawa Y, et al. Prognostic impact of muscle and fat mass in patients with heart failure. J Cachexia Sarcopenia Muscle. 2021;12(3):568–576.
- Pandhi P, Streng KW, Anker SD, et al. The value of spot urinary creatinine as a marker of muscle wasting in patients with new-onset or worsening heart failure. J Cachexia Sarcopenia Muscle. 2021;12(3):555–567.
- Barnard AM, Lott DJ, Batra A, et al. Characterizing expiratory respiratory muscle degeneration in Duchenne muscular dystrophy using MRI. Chest. 2022;161(3):753–763.
- Sigoli E, Antao RA, Guerreiro MP, et al. Effects of low-intensity and long-term aerobic exercise on the psoas muscle of mdx mice: an experimental model of Duchenne muscular dystrophy. Int J Mol Sci. 2022;23(9):4483.
- Ozkal O, Topuz S, Karahan S, et al. Clinical predictors of pulmonary functions, respiratory/peripheral muscle strength and exercise capacity at discharge in adults with burn injury. Disabil Rehabil. 2021;43(20):2875–2881.
- Brightwell CR, Hanson ME, El AA, et al. Thermal injury initiates pervasive fibrogenesis in skeletal muscle. Am J Physiol Cell Physiol. 2020;319(2):C277–C287.
- Morita T, Hayashi K. Actin-Related protein 5 functions as a novel modulator of MyoD and MyoG in skeletal muscle and in rhabdomyosarcoma. Elife. 2022;11:e77746.
- Szabo K, Varga D, Vegh AG, et al. Syndecan-4 affects myogenesis via Rac1-mediated actin remodeling and exhibits copy-number amplification and increased expression in human rhabdomyosarcoma tumors. Cell Mol Life Sci. 2022;79(2):122.
- Hawke TJ, Garry DJ. Myogenic satellite cells: physiology to molecular biology. J Appl Physiol. 2001;91(2):534–551.
- Tremblay P, Dietrich S, Mericskay M, et al. A crucial role for Pax3 in the development of the hypaxial musculature and the long-range migration of muscle precursors. Dev Biol. 1998;203(1):49–61.
- Mckinnell IW, Ishibashi J, Le GF, et al. Pax7 activates myogenic genes by recruitment of a histone methyltransferase complex. Nat Cell Biol. 2008;10(1):77–84.
- Kawabe Y, Wang YX, Mckinnell IW, et al. Carm1 regulates Pax7 transcriptional activity through MLL1/2 recruitment during asymmetric satellite stem cell divisions. Cell Stem Cell. 2012;11(3):333–345.
- von Maltzahn J, Jones AE, Parks RJ, et al. Pax7 is critical for the normal function of satellite cells in adult skeletal muscle. Proc Natl Acad Sci. 2013;110(41):16474–16479.
- Ganassi M, Badodi S, Wanders K, et al. Myogenin is an essential regulator of adult myofibre growth and muscle stem cell homeostasis. Elife. 2020;9:e60445.
- Choi J, Costa ML, Mermelstein CS, et al. MyoD converts primary dermal fibroblasts, chondroblasts, smooth muscle, and retinal pigmented epithelial cells into striated mononucleated myoblasts and multinucleated myotubes. Proc Natl Acad Sci. 1990;87(20):7988–7992.
- Davis RL, Weintraub H, Lassar AB. Expression of a single transfected cDNA converts fibroblasts to myoblasts. Cell. 1987;51(6):987–1000.
- Megeney LA, Rudnicki MA. Determination versus differentiation and the MyoD family of transcription factors. Biochem Cell Biol. 1995;73(9–10):723–732.
- Rudnicki MA, Jaenisch R. The MyoD family of transcription factors and skeletal myogenesis. Bioessays. 1995;17(3):203–209.
- Hasty P, Bradley A, Morris JH, et al. Muscle deficiency and neonatal death in mice with a targeted mutation in the myogenin gene. Nature. 1993;364(6437):501–506.
- Sarem Z, Bumke-Vogt C, Mahmoud AM, et al. Glucagon decreases IGF-1 bioactivity in humans, independently of insulin, by modulating its binding proteins. J Clin Endocrinol Metab. 2017;102(9):3480–3490.
- Schoffski P, Wozniak A, Leahy MG, et al. The tyrosine kinase inhibitor crizotinib does not have clinically meaningful activity in heavily pre-treated patients with advanced alveolar rhabdomyosarcoma with FOXO rearrangement: European organisation foR research and treatment of cancer phase 2 trial 90101 ‘CREATE’. Eur J Cancer. 2018;94:156–167.
- Baar MP, Brandt R, Putavet DA, et al. Targeted apoptosis of senescent cells restores tissue homeostasis in response to chemotoxicity and aging. Cell. 2017;169(1):132–147.
- Robertson J, Coleman RE, Cheung KL, et al. Proliferation and AKT activity biomarker analyses after capivasertib (AZD5363) treatment of patients with ER(+) invasive breast cancer (STAKT). Clin Cancer Res. 2020;26(7):1574–1585.
- Le HH, Cinaroglu SS, Manalo EC, et al. Molecular modelling of the FOXO4-TP53 interaction to design senolytic peptides for the elimination of senescent cancer cells. EBioMedicine. 2021;73:103646.
- Consortium EP. An integrated encyclopedia of DNA elements in the human genome. Nature. 2012;489(7414):57–74.
- Zhang ZK, Li J, Guan D, et al. A newly identified lncRNA MAR1 acts as a miR-487b sponge to promote skeletal muscle differentiation and regeneration. J Cachexia Sarcopenia Muscle. 2018;9(3):613–626.
- Cesana M, Cacchiarelli D, Legnini I, et al. A long noncoding RNA controls muscle differentiation by functioning as a competing endogenous RNA. Cell. 2011;147(2):358–369.
- Chiang HR, Schoenfeld LW, Ruby JG, et al. Mammalian microRnas: experimental evaluation of novel and previously annotated genes. Genes Dev. 2010;24(10):992–1009.
- Khanduja JS, Calvo IA, Joh RI, et al. Nuclear noncoding RNAs and genome stability. Mol Cell. 2016;63(1):7–20.
- Wang L, Zhao Y, Bao X, et al. LncRNA dum interacts with Dnmts to regulate Dppa2 expression during myogenic differentiation and muscle regeneration. Cell Res. 2015;25(3):335–350.
- Chen R, Lei S, Jiang T, et al. Roles of lncRNAs and circRNAs in regulating skeletal muscle development. Acta Physiol. 2020;228(2):e13356.
- Chen R, Lei S, Jiang T, et al. Regulation of skeletal muscle atrophy in cachexia by microRNAs and long non-coding RNAs. Front Cell Dev Biol. 2020;8:577010.
- Banerji J, Rusconi S, Schaffner W. Expression of a beta-globin gene is enhanced by remote SV40 DNA sequences. Cell. 1981;27(2 Pt 1):299–308.
- Whyte WA, Orlando DA, Hnisz D, et al. Master transcription factors and mediator establish super-enhancers at key cell identity genes. Cell. 2013;153(2):307–319.
- Hnisz D, Abraham BJ, Lee TI, et al. Super-Enhancers in the control of cell identity and disease. Cell. 2013;155(4):934–947.
- Witte S, Bradley A, Enright AJ, et al. High-Density P300 enhancers control cell state transitions. BMC Genomics. 2015;16:903.
- Henriques T, Scruggs BS, Inouye MO, et al. Widespread transcriptional pausing and elongation control at enhancers. Genes Dev. 2018;32(1):26–41.
- De Santa F, Barozzi I, Mietton F, et al. A large fraction of extragenic RNA pol II transcription sites overlap enhancers. PLoS Biol. 2010;8(5):e1000384.
- Kim TK, Hemberg M, Gray JM, et al. Widespread transcription at neuronal activity-regulated enhancers. Nature. 2010;465(7295):182–187.
- Kaikkonen MU, Spann NJ, Heinz S, et al. Remodeling of the enhancer landscape during macrophage activation is coupled to enhancer transcription. Mol Cell. 2013;51(3):310–325.
- Suzuki HI, Young RA, Sharp PA. Super-Enhancer-Mediated RNA processing revealed by integrative MicroRNA network analysis. Cell. 2017;168(6):1000–1014.
- Oldridge DA, Wood AC, Weichert-Leahey N, et al. Genetic predisposition to neuroblastoma mediated by a LMO1 super-enhancer polymorphism. Nature. 2015;528(7582):418–421.
- Ounzain S, Pedrazzini T. Super-Enhancer lncs to cardiovascular development and disease. Biochim Biophys Acta. 2016;1863(7 Pt B):1953–1960.
- Pacheco MP, John E, Kaoma T, et al. Integrated metabolic modelling reveals cell-type specific epigenetic control points of the macrophage metabolic network. BMC Genomics. 2015;16:809.
- Herranz D, Ambesi-Impiombato A, Palomero T, et al. A NOTCH1-driven MYC enhancer promotes T cell development, transformation and acute lymphoblastic leukemia. Nat Med. 2014;20(10):1130–1137.
- Benner C, Isoda T, Murre C. New roles for DNA cytosine modification, eRNA, anchors, and superanchors in developing B cell progenitors. Proc Natl Acad Sci. 2015;112(41):12776–12781.
- Achour M, Le Gras S, Keime C, et al. Neuronal identity genes regulated by super-enhancers are preferentially down-regulated in the striatum of Huntington’s disease mice. Hum Mol Genet. 2015;24(12):3481–3496.
- Chapuis J, Hansmannel F, Gistelinck M, et al. Increased expression of BIN1 mediates Alzheimer genetic risk by modulating tau pathology. Mol Psychiatry. 2013;18(11):1225–1234.
- Liu Z, Zhang X, Lei H, et al. CASZ1 induces skeletal muscle and rhabdomyosarcoma differentiation through a feed-forward loop with MYOD and MYOG. Nat Commun. 2020;11(1):911.
- Zhao Y, Zhou J, He L, et al. MyoD induced enhancer RNA interacts with hnRNPL to activate target gene transcription during myogenic differentiation. Nat Commun. 2019;10(1):5787.
- Ehrlich KC, Paterson HL, Lacey M, et al. DNA hypomethylation in intragenic and intergenic enhancer chromatin of muscle-specific genes usually correlates with their expression. Yale J Biol Med. 2016;89(4):441–455.
- Scott LJ, Erdos MR, Huyghe JR, et al. The genetic regulatory signature of type 2 diabetes in human skeletal muscle. Nat Commun. 2016;7(1):11764.
- Brunk BP, Goldhamer DJ, Emerson CJ. Regulated demethylation of the myoD distal enhancer during skeletal myogenesis. Dev Biol. 1996;177(2):490–503.
- Carrio E, Diez-Villanueva A, Lois S, et al. Deconstruction of DNA methylation patterns during myogenesis reveals specific epigenetic events in the establishment of the skeletal muscle lineage. Stem Cells. 2015;33(6):2025–2036.
- Peng XL, So KK, He L, et al. MyoD- and FoxO3-mediated hotspot interaction orchestrates super-enhancer activity during myogenic differentiation. Nucleic Acids Res. 2017;45(15):8785–8805.
- Gryder BE, Wachtel M, Chang K, et al. Miswired enhancer logic drives a cancer of the muscle lineage. iScience. 2020;23(5):101103.
- Gryder BE, Yohe ME, Chou H, et al. PAX3–FOXO1 establishes myogenic super enhancers and confers BET bromodomain vulnerability. Cancer Discov. 2017;7(8):884–899.
- Yohe ME, Gryder BE, Shern JF, et al. MEK inhibition induces MYOG and remodels super-enhancers in RAS-driven rhabdomyosarcoma. Sci Transl Med. 2018;10(448):eaan4470.
- De Repentigny Y, Marshall P, Worton RG, et al. The mouse dystrophin muscle enhancer-1 imparts skeletal muscle, but not cardiac muscle, expression onto the dystrophin Purkinje promoter in transgenic mice. Hum Mol Genet. 2004;13(22):2853–2862.
- Kwon B, Fansler MM, Patel ND, et al. Enhancers regulate 3’ end processing activity to control expression of alternative 3‘UTR isoforms. Nat Commun. 2022;13(1):2709.
- de Almeida BP, Reiter F, Pagani M, et al. DeepSTARR predicts enhancer activity from DNA sequence and enables the de novo design of synthetic enhancers. Nat Genet. 2022;54(5):613–624.
- Tsai P, Dell Orso S, Rodriguez J, et al. A muscle-specific enhancer RNA mediates cohesin recruitment and regulates transcription in trans. Mol Cell. 2018;71(1):129–141.
- Mousavi K, Zare H, Dell’Orso S, et al. eRnas promote transcription by establishing chromatin accessibility at defined genomic loci. Mol Cell. 2013;51(5):606–617.
- Asakura A, Lyons GE, Tapscott SJ. The regulation of MyoD gene expression: conserved elements mediate expression in embryonic axial muscle. Dev Biol. 1995;171(2):386–398.
- Chen JC, Goldhamer DJ. The core enhancer is essential for proper timing of MyoD activation in limb buds and branchial arches. Dev Biol. 2004;265(2):502–512.
- Goldhamer DJ, Faerman A, Shani M, et al. Regulatory elements that control the lineage-specific expression of myoD. Science. 1992;256(5056):538–542.
- Pownall ME, Gustafsson MK, Emerson CJ. Myogenic regulatory factors and the specification of muscle progenitors in vertebrate embryos. Annu Rev Cell Dev Biol. 2002;18(1):747–783.
- Scionti I, Hayashi S, Mouradian S, et al. LSD1 controls timely MyoD expression via MyoD core enhancer transcription. Cell Rep. 2017;18(8):1996–2006.
- Metzger E, Wissmann M, Yin N, et al. LSD1 demethylates repressive histone marks to promote androgen-receptor-dependent transcription. Nature. 2005;437(7057):436–439.
- Mulligan P, Yang F, Di Stefano L, et al. A SIRT1-LSD1 corepressor complex regulates Notch target gene expression and development. Mol Cell. 2011;42(5):689–699.
- Shi Y, Lan F, Matson C, et al. Histone demethylation mediated by the nuclear amine oxidase homolog LSD1. Cell. 2004;119(7):941–953.
- Yang M, Gocke CB, Luo X, et al. Structural basis for CoREST-dependent demethylation of nucleosomes by the human LSD1 histone demethylase. Mol Cell. 2006;23(3):377–387.
- Cichewicz MA, Kiran M, Przanowska RK, et al. MUNC, an enhancer RNA upstream from the MYOD gene, induces a subgroup of myogenic transcripts in trans independently of MyoD. Mol Cell Biol. 2018;38(20): e00655-17.
- Mueller AC, Cichewicz MA, Dey BK, et al. MUNC, a long noncoding RNA that facilitates the function of MyoD in skeletal myogenesis. Mol Cell Biol. 2015;35(3):498–513.
- Lluri G, Jaworski DM. Regulation of TIMP-2, MT1-MMP, and MMP-2 expression during C2C12 differentiation. Muscle Nerve. 2005;32(4):492–499.
- Bornemann A, Schmalbruch H. Desmin and vimentin in regenerating muscles. Muscle Nerve. 1992;15(1):14–20.
- So K, Huang Y, Zhang S, et al. seRNA PAM controls skeletal muscle satellite cell proliferation and aging through trans regulation of Timp2 expression synergistically with Ddx5. Aging Cell. 2022;21(8):e13673. DOI:10.1111/acel.13673
- Lee J, Park Y, Park S, et al. Brd4 binds to active enhancers to control cell identity gene induction in adipogenesis and myogenesis. Nat Commun. 2017;8(1):2217.
- Loven J, Hoke HA, Lin CY, et al. Selective inhibition of tumor oncogenes by disruption of super-enhancers. Cell. 2013;153(2):320–334.
- Bhagwat AS, Roe JS, Mok B, et al. BET bromodomain inhibition releases the mediator complex from select cis-regulatory elements. Cell Rep. 2016;15(3):519–530.
- Kanno T, Kanno Y, Leroy G, et al. BRD4 assists elongation of both coding and enhancer RNAs by interacting with acetylated histones. Nat Struct Mol Biol. 2014;21(12):1047–1057.
- Sengupta D, Kannan A, Kern M, et al. Disruption of BRD4 at H3K27Ac-enriched enhancer region correlates with decreased c-Myc expression in Merkel cell carcinoma. Epigenetics. 2015;10(6):460–466.
- Shin HY. Targeting super-enhancers for disease treatment and diagnosis. Mol Cells. 2018;41(6):506–514.
- Chipumuro E, Marco E, Christensen CL, et al. CDK7 inhibition suppresses super-enhancer-linked oncogenic transcription in MYCN-driven cancer. Cell. 2014;159(5):1126–1139.
- Chapuy B, Mckeown MR, Lin CY, et al. Discovery and characterization of super-enhancer-associated dependencies in diffuse large B cell lymphoma. Cancer Cell. 2013;24(6):777–790.
- Farh KK, Marson A, Zhu J, et al. Genetic and epigenetic fine mapping of causal autoimmune disease variants. Nature. 2015;518(7539):337–343.
- Aune TM, Crooke PR, Patrick AE, et al. Expression of long non-coding RNAs in autoimmunity and linkage to enhancer function and autoimmune disease risk genetic variants. J Autoimmun. 2017;81:99–109.
- Kyzar EJ, Zhang H, Pandey SC. Adolescent alcohol exposure epigenetically suppresses amygdala Arc enhancer RNA expression to confer adult anxiety susceptibility. Biol Psychiatry. 2019;85(11):904–914.
- Mirtschink P, Bischof C, Pham MD, et al. Inhibition of the hypoxia-inducible factor 1alpha-induced cardiospecific HERNA1 enhance-templated RNA protects from heart disease. Circulation. 2019;139(24):2778–2792.
- Liang J, Zhou H, Gerdt C, et al. Epstein-Barr virus super-enhancer eRnas are essential for MYC oncogene expression and lymphoblast proliferation. Proc Natl Acad Sci, USA. 2016;113(49):14121–14126.
- Le Gras S, Keime C, Anthony A, et al. Altered enhancer transcription underlies Huntington’s disease striatal transcriptional signature. Sci Rep. 2017;7(1):42875. DOI:10.1038/srep42875
- Huang Z, Du G, Huang X, et al. The enhancer RNA lnc-SLC4A1-1 epigenetically regulates unexplained recurrent pregnancy loss (URPL) by activating CXCL8 and NF-kB pathway. EBioMedicine. 2018;38:162–170.
- Huang Z, Yu H, Du G, et al. Enhancer RNA lnc-CES1-1 inhibits decidual cell migration by interacting with RNA-binding protein FUS and activating PPARgamma in URPL. Mol Ther Nucleic Acids. 2021;24:104–112.
- Tan Y, Jiang C, Jia Q, et al. A novel oncogenic seRNA promotes nasopharyngeal carcinoma metastasis. Cell Death Dis. 2022;13(4):401.
- Fang K, Huang W, Sun YM, et al. Cis-Acting lnc-eRNA SEELA directly binds histone H4 to promote histone recognition and leukemia progression. Genome Biol. 2020;21(1):269.
- Mo J, Zhang L, Li H, et al. The enhancer RNA ADCY10P1 is associated with the progression of ovarian cancer. J Ovarian Res. 2022;15(1):61.
- Deforzh E, Uhlmann EJ, Das E, et al. Promoter and enhancer RNAs regulate chromatin reorganization and activation of miR-10b/hoxd locus, and neoplastic transformation in glioma. Mol Cell. 2022;82(10):1894–1908.