Abstract
Objective: During dynamic injury scenarios, such as motor vehicle crashes, neck biomechanics contribute to head excursion and acceleration, influencing head injuries. One important tool in understanding head and neck dynamics is computational modeling. However, realistic and stable muscle activations for major muscles are required to realize meaningful kinematic responses. The objective was to determine cervical muscle activation states for 6-year-old, 10-year-old, and adult 50th percentile male computational head and neck models. Currently, pediatric models including muscle activations are unable to maintain the head in an equilibrium position, forcing models to begin from nonphysiologic conditions. Recent work has realized a stationary initial geometry and cervical muscle activations by first optimizing responses against gravity. Accordingly, our goal was to apply these methods to Duke University's head–neck model validated using living muscle response and pediatric cadaveric data.
Methods: Activation schemes maintaining an upright, stable head for 22 muscle pairs were found using LS-OPT. Two optimization problems were investigated: a relaxed state, which minimized muscle fatigue, and a tensed activation state, which maximized total muscle force. The model's biofidelity was evaluated by the kinematic response to gravitational and frontal impact loading conditions. Model sensitivity and uncertainty analyses were performed to assess important parameters for pediatric muscle response. Sensitivity analysis was conducted using multiple activation time histories. These included constant activations and an optimal muscle activation time history, which varied the activation level of flexor and extensor groups, and activation initiation and termination times.
Results: Relaxed muscle activations decreased with increasing age, maintaining upright posture primarily through extensor activation. Tensed musculature maintained upright posture through coactivation of flexors and extensors, producing up to 32 times the force of the relaxed state. Without muscle activation, the models fell into flexion due to gravitational loading. Relaxed musculature produced 28.6–35.8 N of force to the head, whereas tensed musculature produced 450–1023 N. Pediatric model stiffnesses were most sensitive to muscle physiological cross-sectional area.
Conclusions: Though muscular loads were not large enough to cause vertebral compressive failure, they would provide a prestressed state that could protect the vertebrae during tensile loading but might exacerbate risk during compressive loading. For example, in the 10-year-old, a load of 602 N was produced, though estimated compressive failure tolerance is only 2.8 kN. Including muscles and time-variant activation schemes is vital for producing biofidelic models because both vary by age. The pediatric activations developed represent physiologically appropriate sets of initial conditions and are based on validated adult cadaveric data.
Supplemental materials are available for this article. Go to the publisher's online edition of Traffic Injury Prevention to view the supplemental file.
Introduction
The leading cause of death among children ages one to 19 years in the United States is traumatic injury (Centers for Disease Control and Prevention [CDC] Citation2007). Pediatric injuries resulted in over 17,000 fatalities in 2007, which equates to 21 deaths per 100,000 children (CDC Citation2007). Motor vehicle crashes (MVCs) are the leading cause of injuries sustained by children, representing almost 40 percent of all fatal injuries in the United States (CDC Citation2007). The National Highway Traffic Safety Administration (NHTSA) reported that in 2007 there were an average of 5 children age 14 years and younger killed and 548 injured daily in MVCs in the United States (NHTSA Citation2007a).
The head and neck of children are regions of the body that are of particular interest during MVCs. The head, either alone or in conjunction with multiple-system injury, is the most frequently injured body region among children in MVCs. The incidence of children sustaining head injury is 8.3 cases per 1000 crashes, which is 7 times the incidence of the next highest body region (Durbin et al. Citation2001; Partners for Child Passenger Safety Citation2008). Injuries to the pediatric cervical spine, although not as common, are debilitating and often fatal. Of children who sustained cervical injury, the mortality rate was 27 percent and the overall incidence of neurological deficits was 66 percent (Platzer et al. Citation2007). Neck injuries are associated with head injury in 40 percent of cases (Eleraky et al. Citation2000). Notwithstanding the relative infrequency of these injuries, understanding the kinematics and kinetics of the pediatric neck is critical to fully investigate pediatric head injury. During dynamic injury scenarios, such as MVCs, neck biomechanics determine head excursion and acceleration, thus influencing head injuries. By understanding pediatric head and neck biological and mechanical properties, better safety measures can be developed to reduce pediatric injury.
The use of passenger restraint systems has been shown to significantly decrease the risk of injury and death to all motor vehicle occupants (Cummings et al. Citation2003; Houston et al. Citation1996; McGwin et al. Citation2003; NHTSA Citation2007b), including children (Elliott et al. Citation2006; Halman et al. Citation2002; Javouhey et al. Citation2006; NHTSA Citation2007a; Valent et al. Citation2002). Engineering advancements to motor vehicle restraint systems and passenger compartments provide a direct means to further reduce pediatric MVC injuries. Anthropomorphic test devices (ATDs) are the principal tool to test the safety effectiveness of engineering advancements and include a range of pediatric ages. Though beneficial, child ATDs were developed with limited human pediatric biomechanical data.
The accuracy of child ATD head and neck biofidelity and injury criteria is limited by the paucity of relevant pediatric research. Common approaches for assessing adult human biomechanics can be adapted for the study of pediatric biomechanics, including human volunteers, cadavers, animal surrogates, and computer models. These approaches each have inherent limitations and are further limited when applied to the pediatric population.
Volunteer testing is limited largely by the need to use loading scenarios that will not cause injury. To date, only a single volunteer study at the Children's Hospital of Philadelphia has been published in which pediatric volunteers were subjected to a loading scenario comparable to low-speed MVCs (Arbogast et al. Citation2009). Adult males, as well as pediatric males aged 6 to 14 years, were subjected to low-speed frontal impacts.
Though cadavers have been the chief surrogate for human biomechanical research, they are limited in that they lack the contribution of active musculature and that they are not sensitive to minor injury such as pain or loss of consciousness. In addition, the use of pediatric cadavers has been specifically limited by the rarity of pediatric cadavers. Only a handful of pediatric cadavers studies for the neck have been conducted (e.g., Dejeammes et al. Citation1984; Duncan Citation1874; Kallieris et al. Citation1976; Luck et al. Citation2008, 2013; Ouyang Citation2005; Wismans et al. Citation1979). These studies reported cervical stiffness and failure loads and are extremely valuable for understanding the pediatric neck. However, they lack the contribution of active musculature needed for use in designing pediatric ATDs.
Several studies on pediatric cervical spine have been conducted using animal surrogates as an alternative to pediatric cadavers (Aldman et al. Citation1974; Backaitis et al. Citation1975; Ching et al. Citation2001; Clarke et al. Citation2007; Hilker et al. Citation2002; Mertz et al. Citation1982; Nuckley et al. Citation2005, Citation2007; Pintar et al. Citation2000; Prasad and Daniel Citation1984; Schreck and Patrick Citation1975). Animal surrogate studies provide valuable insights into the biomechanical response of the cervical spine but are limited by interspecies differences between the animal surrogate and human pediatric cervical spines.
In view of the limitations of human volunteer, cadavers, and animal surrogate testing, computational modeling of the pediatric head and neck offers a valuable method to estimate biofidelity requirements and injury assessment reference values for the child ATDs. Experimentally validated models developed using accurate pediatric geometric and material properties can be used to simulate numerous loading conditions to investigate injury potential. Though numerous models of the adult 50th percentile male have been created, only a handful of pediatric models exist. Five models were developed by scaling adult models or adult properties and then validating them using scaled adult biofidelity corridors (de Lange et al. Citation2001; Dupuis et al. Citation2006; Liu and Yang Citation2002; Meyer et al. Citation2009; Mizuno et al. Citation2005). Another study, specifically examining the lower cervical spine (Kumaresan et al. Citation2000), indicated that modeling the pediatric cervical spine while incorporating anatomical developmental changes had a larger effect on the response of the model compared to a model developed purely on scaling. Therefore, studies of pediatric geometric and material properties were utilized in order to create pediatric computational models used in this study.
Computational models can be used to investigate and better understand head and neck kinematics and injury potential during traumatic loading scenarios such as MVCs. Computational models have advantages over other biomechanical testing methods in that they can study innumerable loading scenarios, investigate injury onset, examine structural component load sharing, and be tailored and developed to investigate specific biomechanical concerns.
However, computational head and neck models are unstable under gravitational loading unless cervical muscles are activated to maintain an upright, neutral posture; initial muscle activation levels are therefore required to maintain posture during impact simulations. Additionally, initial conditions due to gravitational stabilization may affect the kinetics of the model during dynamic loading conditions (Chancey et al. Citation2003). Many head and neck models reported in the literature did not include gravity in their simulations in order to avoid the challenges of determining a stabilizing muscle activation scheme. The authors justified this decision on the basis that the magnitude of gravity is small compared to the loading magnitude and therefore the gravitational effects are negligible (Brolin et al. Citation2005; Panzer Citation2006; van der Horst Citation2002). Still others failed to report whether gravity was included in their simulations (Deng and Goldsmith Citation1987; Golinski and Gentle Citation2005; Lee et al. Citation2004; Meyer et al. Citation2004; Stemper et al. Citation2004). A select few studies reported determining gravitational stabilization muscle activation schemes that were initiated before simulation loading (Brelin-Fornari et al. Citation2005; Chancey et al. Citation2003; Deng and Fu Citation2002; Östh et al. Citation2012). Chancey et al. (Citation2003) reported a method to find relaxed and tensed optimal cervical muscle activations, representing neck properties of both unaware and aware subjects.
The influence of neck muscles on dynamic muscle response for the pediatric population has not been studied to date. In order to investigate the effect of muscles for the pediatric population, the present study had 2 primary objectives. First, follow the methodology reported by Chancey et al. (Citation2003) to develop initial relaxed and tensed muscle activation states for 6- and 10-year-old pediatric computational head and neck models to be applied to dynamic loading conditions. Second, the effects of muscle activations were investigated by simulating low-speed frontal impacts using four activation levels, 2 constant and 2 dynamic, for the 6-year-old, 10-year-old, and adult computational head and neck models.
Methods
Model Development
The adult 50th percentile male and pediatric 6- and 10-year-old computational head and neck models () developed and used in the current study are hybrid multibody and finite element models. Previous models (Camacho Citation1998; Chancey Citation2005; Van Ee Citation2000) provided the foundation for the current models. The osteoligamentous cervical spine consists of a finite element head (modeled as a rigid body for this study), as well as 7 rigid body vertebrae (C1/C2, C3, C4, C5, C6, C7, T1) connected by 6 degree of freedom nonlinear viscoelastic beam intervertebral joints (occiput-C2, C2-C3, C3-C4, C4-C5, C5-C6, C6-C7, C7-T1). The atlas and axis were modeled as a single body because the upper cervical spine functionally acts as a single joint (Nightingale et al. Citation1998, Citation2002). Cervical musculature was modeled using sliding contact guided cables to simulate muscle wrapping. Twenty-two cervical muscles were separated into 81 muscle strands. Muscle models were rate sensitive and included passive and active properties. The models assumed an initial lordotic, neutral posture (Camacho Citation1998; Dibb Citation2011).
Fig. 1 Lateral view of the adult and pediatric head and neck models (color figure available online).
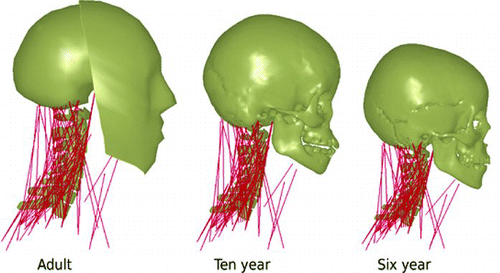
The pediatric head and neck models were developed using pediatric biomechanical properties from pediatric cadaveric and volunteer studies. Head inertial properties and anthropometric data were reported by Loyd et al. (Citation2010). Vertebral inertial properties developed from cadaveric cervical computed tomography scans as well as cervical muscle properties developed from clinical cervical magnetic resonance imaging scans were reported by Dibb (Citation2011). Pediatric intervertebral joint properties were incorporated from pediatric cadaveric osteoligamentous cervical spine mechanical tensile tests (Luck et al. Citation2008, 2013).
Further specifics of model development and design for both the adult 50th percentile male and pediatric 6- and 10-year-old models are detailed by Dibb (Citation2011). Modeling and analysis were performed using the general-purpose multiphysics simulations software LS-DYNA (Livermore Software Technology Corporation, Livermore, CA).
Determination of Initial Muscle Activations
To determine the significance of muscle activations in the developed adult and pediatric head and neck models, the model's kinematic response to gravity was evaluated with and without muscle activations. Gravity was simulated in LS-DYNA through a constant 9.81 m/s2 load in the superior to inferior direction. T1 was held fixed in both rotation and translation during the 100 ms simulations. All other parts were allowed free motion in the sagittal plane. The stability of the models was quantified using the maximal head center of gravity (CG) translational and rotational displacement.
Muscle activation schemes that maintained an upright, stable posture were found through linear response surface optimization using LS-OPT (LSTC). Activation levels, 0 ≤ α ≤ 1, of each of the 22 muscle pairs () were varied using Latin hypercube point selection for 35 experiments per iteration. Two optimization problems were investigated, a relaxed and tensed activation state.
Fig. 2 Relaxed and tensed state muscle activation levels, 0 ≤ α ≤ 1, of the 6- and 10-year-old pediatric and adult models that maintain a neutral, upright head and neck posture provide initial conditions for dynamic loading. Relaxed activation levels decreased with increasing age.
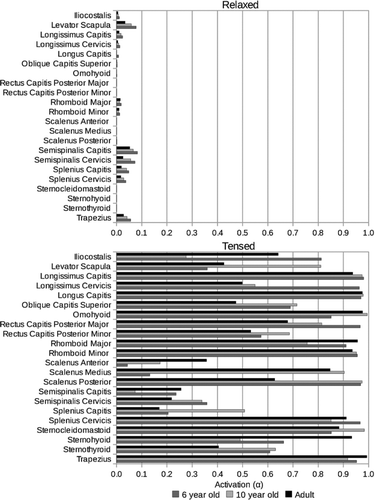
The relaxed activation state represented an unaware subject and optimization sought to minimize muscle fatigue. Muscle fatigue was defined as the sum of squares of the ratio of muscle force to maximal muscle force (Pedotti et al. Citation1978) resulting in the optimization solution of EquationEq. (1).
The tensed activation state represented an aware subject and optimization sought to maximize total muscle force. The tensed optimization function is expressed in EquationEq. (2):
Validation Against Low-Speed Frontal Impact
The only available pediatric volunteer results available for pediatric model validation are low-speed frontal impact tests conducted at Children's Hospital of Philadelphia (Arbogast et al. Citation2009). Twenty pediatric males ranging in age from 6 to 14 years and 10 adult males were belted onto a hydraulically controlled sled and subject to low-speed frontal impacts (). These impacts were comparable to those experienced on bumper car rides at amusement parks.
Fig. 3 Pediatric and adult mean volunteer low-speed frontal impact test with peak sled accelerations of 3.6 and 3.8 g used to validate the pediatric and adult head and neck computer models (Arbogast et al. Citation2009).
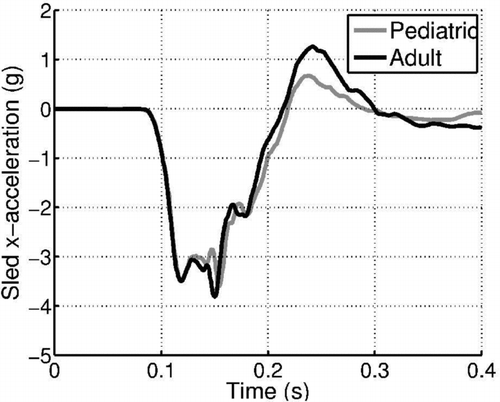
Fig. 4 Adult and pediatric head and neck models were subjected to low-speed frontal impact through imposed T1 displacements from volunteer experiments (Arbogast et al. Citation2009).
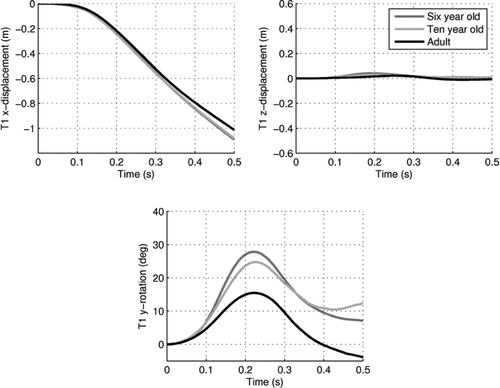
The adult and pediatric head and neck models developed in this study were subjected to the T1 linear acceleration and rotational displacement pulses from the volunteer tests. Average linear displacements from motion capture markers attached to the T1 of 6- to 8-year-old, 9- to 11-year-old, and adult volunteers (Arbogast et al. Citation2009) were prescribed to the T1 of the 6-year-old, 10-year-old, and adult models, respectively. T1 y-direction rotational displacement rotations were determined from motion capture markers attached to the skin above the T1 and T4 spinous processes. The visual markers were assumed to be rigidly attached to the vertebral spinous processes and that the rotation of the T1 to T4 marker linkage represented the rotation of the torso. The prescribed T1 displacements and rotations for the 3 models are presented in . Gravity was simulated as a constant body force in the superior to inferior direction.
Model responses were assessed by the translational displacements of the external auditory meatus (EAM) and nasion, relative to the T1 marker, and head rotational velocity. Volunteer response corridors were defined as the average response time history plus and minus one standard deviation (Arbogast et al. Citation2009).
The model response compared to volunteer corridors was quantified through a correlation analysis as a linear combination of a corridor method and a cross-correlation method (Gehre et al. Citation2009; Panzer et al. Citation2011; Xu et al. Citation2000). The corridor method quantified how well the model response fit within the volunteer corridors and resulted in a score of one if the model fit within the one standard deviation corridor, a value of zero if the model fell outside the 2 standard deviation corridor, and a linear transition between one and zero. The cross-correlation method quantified the magnitude, phase shift, and shape of the model response against the volunteer corridor and resulted in a score between zero and one with a linear transition between them. The mean cross-correlation score for magnitude, phase shift, and shape was then averaged with that of the corridor method to provide a correlation score for a specific response, such as head rotational velocity. An average correlation score for a simulation was the average of the 5 response correlations scores of nasion and EAM x-direction and z-direction displacement and head rotational velocity. The correlation score was qualified using the ISO-TR 9790 biofidelity rating scale (International Organization for Standardization Citation1999) ranging from zero to one representing poor to excellent biofidelity, respectively.
The effects of muscle activation during the low-speed frontal impacts were studied by using 4 activation time histories:
1. | Relaxed activation. The models were subjected to low-speed frontal impact with constant relaxed muscle state using the calculated 1 g gravitational activations. For this simulation, muscle activations were held constant throughout the 500 ms impact event. | ||||
2. | Tensed activation. The models were subjected to low-speed frontal impact with tensed muscle activation states using the calculated 1 g gravitational tensed state. For this simulation, muscle activations were held constant throughout the 500 ms impact event. | ||||
3. | Optimal activation. An optimal muscle activation time history was found for each age that minimized the error between the model and volunteer head rotational velocities using the least squares method and LS-OPT. Muscles were initially assigned relaxed state activations and the cervical muscles were grouped as either extensors or flexors, which were activated as groups. Three parameters were varied for each group during the impact event: activation level, initiation time, and termination time. | ||||
4. | Pediatric model using adult optimal activation. The pediatric models were assigned the adult optimum activation time history. |
Activation time histories were described by a coupled linear dynamic system (Winters and Stark Citation1985, Citation1988). The first component is neuromuscular excitation, which is the dynamics of the neurocontroller input signal and the motorneuronal system. The second dynamic system was activation state, which is the dynamics of the troponin binding with calcium, which initiates the contractile machinery. The neural excitation time constant in the current model was 30 ms (Winters and Stark Citation1988). The activation state time constant was 15 ms for activation and 40 ms for deactivation (Winters Citation1995).
The effects of prescribed T1 displacement boundary conditions were investigated through a sensitivity analysis of the adult model. T1 x-direction translational, z-direction translational, and y-direction rotational displacements were varied over their standard deviations. Latin hypercube sampling was used to select the values and 50 simulations were run per variable (Iman, Campbell, and Helton Citation1981; Iman, Helton, and Campbell Citation1981). With 3 variables, a total of 150 simulations were run. Relationships between model peak displacements and boundary conditions were quantified through Pearson correlation using a significance level of P < .05.
Results
Relaxed and tensed muscle activation states were found that maintained an upright, neutral, lordotic head and neck posture against the force of gravity (). Head CG displacement and rotation constraints were met for both the adult and pediatric models. Muscle activations did result in the compression of the cervical spine and the inferior displacement of the head CG. Though the relaxed muscle state only resulted in 2 mm or less of inferior CG displacement for each model, the tensed muscle state resulted in 4.2, 5.2, and 5.7 mm of inferior displacement for the 6-year-old, 10-year-old, and adult models.
Without muscle activation, the computational head and neck models were unstable and fell into flexion because of gravitational loading. The head CG displaced more than 5 mm anteriorly and 4 mm inferiorly from its initial position over the 100 ms simulation. The head also rotated into flexion 8.4, 8.0, and 5.7° for the 6-year-old, 10-year-old, and adult models.
Relaxed muscle activation levels decreased as age increased (, Appendix A, see online supplement). Activations were on average 2.2 and 1.7 times greater than adult activations for the 6- and 10-year-old models, respectively. No trends by age were found for the tensed muscle activations. The tensed muscle activation state maintained upright posture with the coactivation of flexor and extensor muscles for all age groups. The relaxed muscle activation state maintained upright posture primarily with the activation of extensor muscles.
The tensed state muscles that were attached to the head produced up to 32 times the muscle force compared with the relaxed state muscles. The tensed state muscles produced 450, 602, and 1023 N of force to the head whereas the relaxed state muscles produced 28.6, 29.3, and 35.8 N in the 6-year-old, 10-year-old, and adult models, respectively. The preloads in the tensed activation state as a percentage of the estimated compressive neck tolerance were 44.6, 41.8, and 39.2 for the 6-year-old, 10-year-old, and adult respectively (Luck et al. Citation2008; Nightingale et al. Citation1997). The ratios of 6- and 10-year-old pediatric to adult muscle forces were 0.44 and 0.59 for the tensed and 0.80 and 0.82 for the relaxed states. Muscle forces were primarily in the neck axial direction and their magnitude across the cervical spinal segments increased caudally ().
Fig. 5 Resultant muscle axial force across cervical spinal segments increased caudally and could exacerbate risk of injury during compressive loading.
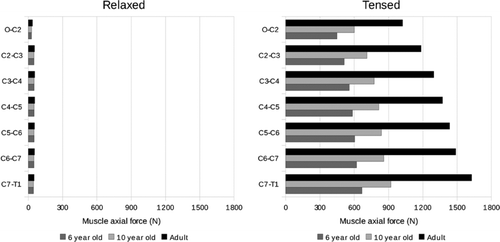
The adult and pediatric head and neck models reproduced the kinematic response of volunteers to low-speed frontal impact (–). The head rotated in flexion and translated anteriorly and inferiorly from neutral posture relative to T1. Peak displacements were reached by approximately 250 ms, after which the head rebounded, returning to its upright, initial position.
Fig. 6 The adult model response due to low-speed frontal impact compared to volunteer corridors. Optimized muscle activation resulted in the most biofidelic response (color figure available online).
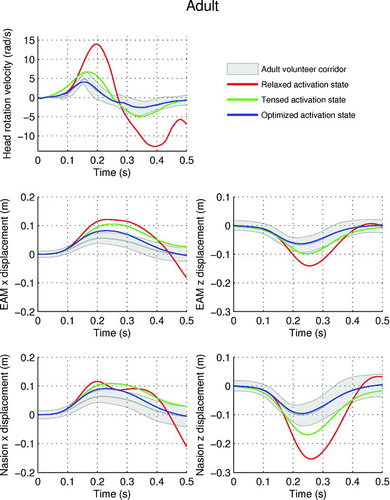
Fig. 7 Six-year-old model response due to low-speed frontal impact compared to volunteer corridors. Optimized activation states that lie between relaxed and tensed states provide the greatest correlation (color figure available online).
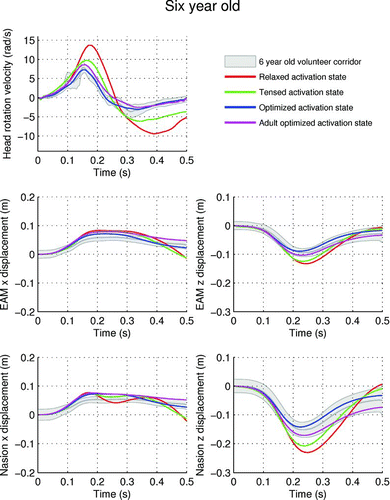
Fig. 8 Ten-year-old model response due to low-speed frontal impact compared to volunteer corridors. Optimized activation states that lie between relaxed and tensed states provide the greatest correlation (color figure available online).
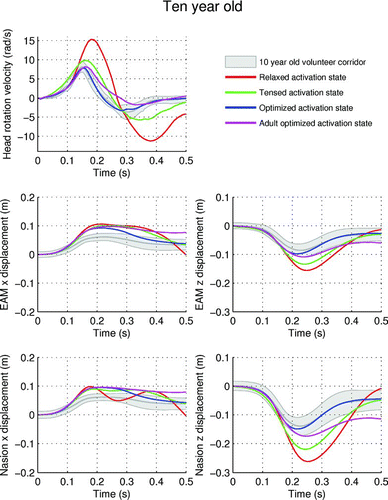
Correlation analyses between the model kinematics and that of the volunteers resulted in average scores greater than 0.6 for all muscle activation scenarios considered, which represents a fair correlation (). Time-dependent activation of the extensors and flexors (optimized) resulted in more biofidelic model responses than activations held constant (either relaxed or tensed) throughout the simulation. Simulations with optimized muscle activation histories had average correlation scores equal to or greater than 0.9, which represents an excellent correlation. Correlation scores were the lowest for the simulations with constant relaxed muscle activation states in which, on average, the head rotated 8.9 times faster and displaced 3.2 times farther than the volunteers. The head during simulations with constant tensed muscle activations also rotated faster and displaced farther than volunteers—2.9 and 2.4 times, respectively—but additionally had head rotations initiated 25 ms earlier than in the volunteer data.
Table 1 Adult and pediatric model correlation scores (average ± standard deviation) from varying muscle activation simulations evaluated against volunteer corridors
The pediatric models required greater dynamic extensor activations to match volunteer head rotational velocities than the adult model (). The optimized 6- and 10-year-old model extensor activations were almost twice those of the adult model. The peak optimized flexor activations were smaller than the extensor activations and ranged from 21 to 38 percent. Though adult optimized activations for both flexors and extensors were smaller, applying the adult activation histories to the pediatric models resulted in average correlation scores greater than 0.7, which represents good correlation ().
Peak head translational displacements and rotational velocities were most sensitive to the applied T1 x-direction displacement (). Peak head translational displacements and rotational velocities were also sensitive to the applied T1 y-direction rotational displacements. Only one of the measures, minimum head rotational velocity, was sensitive to the applied T1 z-direction displacement. Average Pearson correlation coefficients for all activation conditions were 0.81, 0.56, and 0.11 for the applied x translation, y rotation, and z translation, respectively, indicating that the model is most sensitive to x-translational and y-rotational displacements.
Table 2 Correlation coefficients of the adult model sensitivity analyses. T1 kinematic parameters were varied over their standard deviation and the influence on the kinematic output of the head were tabulated
To determine which parameters had the most significant effect, the average correlation score was fit with a general second degree polynomial model shown in EquationEq. (3) with an R 2 value of 0.70.
C is the correlation score and x and y are the T1 x-direction translation and y-direction rotational displacement, expressed as a percentage of standard deviation. The z-direction translation was not included in the correlation score fit because it was not statistically significant in the model.
Discussion
In this effort, gravitational loading was incorporated into a computational model of the head and neck—a limitation of many previous models. One challenge of including gravity is determining initial muscle activations that maintain a stable, upright, neutral posture prior to impact loading. Additionally, it is important to understand the range of possible activation strategies and the sensitivity of these activations to the parameters in the model. The goals of this study were to (1) determine initial relaxed and tensed muscle activation states by optimizing against gravity to maintain a stable initial posture for the 6-year-old, 10-year-old, and adult models and (2) establish how muscle activations affected head kinematics.
Muscle activation states were found that limited head motion due to gravity over 100 ms through optimization that minimized muscle fatigue or maximized muscle force. Motion was limited to less than 6 mm of displacement and half a degree of rotation or less. One limitation of this study was that the presented activation states may be nonunique and conditional to optimization goals and constraints. For example, the models were constrained to movement in the sagittal plane. However, experimental gravitational stabilization is 3-dimensional. Likewise, 3-dimensional data from Arbogast et al. (Citation2009) were projected onto a 2-dimensional plane. Additionally, there is a dearth of experimental activation level data in the literature with which the results can be validated. Furthermore, a linear relationship between muscle force and activation level was defined, but other activation relationships are possible. Other limitations, such as the application of volunteer initial neck angle to the model, may affect activation optimization. However, activation states were generally found to be robust to small disturbances (Dibb Citation2011; Dibb et al. Citation2013). Notwithstanding this limitation, the reported activation states represent physiologically appropriate sets of initial conditions, as evidenced by the model's corridor fits.
The relaxed muscle activation levels were greatest for the 6-year-old model and decreased with age, in contrast to muscle forces, which increased with age. The ratios of pediatric to adult relaxed muscle forces were 0.80 and 0.82, which were very similar to the pediatric-to-adult head mass ratios of 0.75 and 0.80 of the 6- and 10-year-old, respectively. This suggests that the relaxed activation state was dependent on head mass. An increase in head mass would create more flexion moment about the occipital condyles, which would need to be offset by an equal increase in moment about the joint due to contribution from the extensors. The distance from the condyles of both the gravitational load vector and the muscle forces is maintained because the same scale factor scales both distances. However, because head mass scales separately from muscle or neck length, activation states can be dependent on head mass and not muscular length.
For the tensed muscle activation, the total muscle force increased with age. The ratios of pediatric to adult tensed muscle forces were 0.43 and 0.57, which were well correlated with the pediatric to adult muscle physiological cross-sectional area (PCSA) ratios of 0.47 and 0.57 for the 6- and 10-year-old, respectively. As expected, this suggests that the main contributor to total muscle force is total muscle area; increasing the muscle area produces a proportional increase in total muscle force.
For the tensed activation state, compressive preloads reach 40–45 percent of the estimated compressive tolerance of the neck (Luck et al. Citation2008; Nightingale et al. Citation1997). The tensed muscle activation state produced muscle forces large enough to compress the spine up to 6 mm for the adult model. Though these muscle loads were not large enough that they would cause vertebral compressive failure, they would provide a prestressed state that could protect the vertebrae during tensile loading but might exacerbate risk during compressive loading. The pediatric models, like the adult, showed that this muscle-induced precompression was greater for the lower cervical spine than the upper cervical spine and increased caudally.
Fig. 9 Dynamic component of extensor and flexor muscle activation histories for adult, 10-year-old, and 6-year-old that resulted in the optimal head rotational velocity profile for low-speed frontal impact.
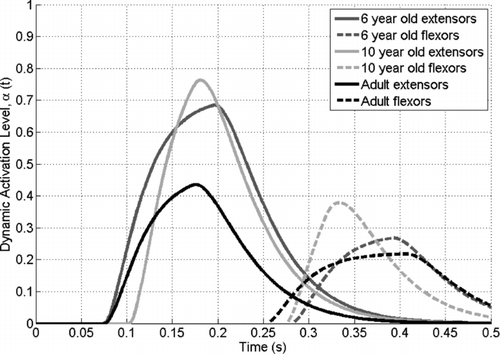
Active muscle response was essential to produce biofidelic response in the dynamic simulations. Initial activation of cervical extensors was needed to obtain head flexion and forward displacement that matched the volunteer data, but flexor activation was needed to control head rebound. Activations that were varied over the simulation time history resulted in greater model to volunteer correlation than simulations where activations were maintained at initial relaxed or tensed levels (, –). A limitation of the current study was the inability to compare optimal model activation time histories to volunteer activations. Though the volunteers were reported to have used their neck muscle during the impact experiments, the degree of muscle activation—recorded from surface electromyography—has not been quantified to date. Though the level of activation could not be validated, the importance of extensor and flexor muscles was demonstrated by applying the optimal adult activations to the pediatric models. The adult optimal extensor activation level was almost half the optimal pediatric muscle activation levels and yet when applied to the pediatric models it still resulted in greater correlation of the models to the volunteer corridors than the models with constant activation states. Pediatric activations are expected to be higher than adult activations due to head mass and muscle volume; however, it is expected that the varying input T1 rotational inputs () also lead to an increased activation level in the pediatric models compared to the adult model.
One of the limitations of the volunteer frontal impact experiments was the tracking of head and vertebrae displacements, which was accomplished through visual markers that were attached to the skin and not the actual bones. The head and neck models were exercised by applying the T1 and T4 vertebra marker displacements as boundary conditions as if those displacements were the actual volunteer vertebral displacements. A sensitivity analysis of the applied T1 boundary conditions showed that the T1 x-direction translation and y-direction rotations had a statistically significant effect on head rotational velocities and translational displacements.
Conclusions
Relaxed and tensed muscle activation states that maintained neutral, upright head and neck posture under gravitational loading were generated that provide muscle activation initial conditions for dynamic simulations, such as frontal impact. These activations were determined not only for the 50th percentile adult but also for 2 pediatric models, ages 6 and 10 years old. The activated muscles were similar across all ages for the relaxed state but less so for the tensed state. However, relaxed activations levels were found to decrease with increasing age.
Furthermore, it was found that muscle activations resulted in muscular preloads that could exacerbate risk during compressive loading. Muscular preloads for the tensed states reached 40–45 percent of estimated compressive neck tolerance, regardless of age. Importantly, these large preloads were only due to activation of the muscles in response to gravity and did not include any additional loads, such as those seen in impact.
Finally, the adult and pediatric head and neck models were validated against low-speed frontal impact volunteer corridors. Overall, the models demonstrated good to excellent biofidelity because the model responses agreed well with volunteer responses. Dynamic activation of neck extensors and flexors resulted in greater correlation (correlation scores > 0.89) between model and volunteer corridors than static activation levels. Volunteer activations were represented computationally by dynamic activations that lie between the relaxed and tensed constant activation states. Additionally, model sensitivity analysis of applied T1 boundary conditions revealed that T1 x-direction translation had the greatest effect on model head translational and rotational displacements. This suggests that accurate tracking of thoracic (T1) kinematics in volunteer studies is critical to accurate neck modeling. This study shows that including muscles and time-variant activation schemes is vital for producing biofidelic computational models.
Appendix
Download Zip (13.4 KB)Acknowledgment
This study was supported by the National Highway Traffic Safety Administration (NHTSA Cooperative Agreement No. DTNH22-08-H-00187).
© Alan T. Dibb, Courtney A. Cox, Roger W. Nightingale, Jason F. Luck, Hattie C. Cutcliffe, Barry S. Myers, Kristy B. Arbogast, Thomas Seacrist, and Cameron R. Bass
References
- Aldman , B , Andersson , A and Saxmark , O . Possible effects of airbag inflation on a standing child . Paper presented at: 18th Conference of the American Association for Automotive Medicine; Toronto, Canada, September 12–14 ,
- Arbogast , K , Balasubramanian , S Seacrist , T . 2009 . Comparison of kinematic responses of the head and spine for children and adults in low-speed frontal sled tests . Stapp Car Crash J. , 53 : 329 – 372 .
- Backaitis , S H , Medlin , J W Jr Radovich , V G . Performance evaluation of child dummies and baboons in child restraint systems in a systematized crash environment . Paper presented at: 19th Stapp Car Crash Conference; San Diego, CA, November 17–19 ,
- Brelin-Fornari , J , Shah , P and El-Sayed , M . 2005 . Physically correlated muscle activation for a human head and neck computational model . Comput Method Biomech. , 8 ( 3 ) : 191 – 199 .
- Brolin , K , Halldin , P and Leijonhufvud , I . 2005 . The effect of muscle activation on neck response . Traffic Inj Prev. , 6 ( 1 ) : 67 – 76 .
- Camacho , DLA. 1998 . Dynamic Response of the Head and Cervical Spine to Near-Vertex Head Impact: An Experimental and Computational Study , Durham , NC : Duke University . [Ph.D. thesis]
- Centers for Disease Control and Prevention . 2007 . WISQARS (Web-Based Injury Statistics Query and Reporting System) , Centers for Disease Control and Prevention .
- Chancey , VC. 2005 . Strength of the Human Neck: Understanding the Contributions of the Ligamentous and Muscular Spine in Tension and Bending , Durham , NC : Duke University . [Ph.D. thesis]
- Chancey , V C , Nightingale , R W , Van Ee , C A , Knaub , K E and Myers , B S . 2003 . Improved estimation of human neck tensile tolerance: reducing the range of reported tolerance using anthropometrically correct muscles and optimized physiologic initial conditions . Stapp Car Crash J. , 47 : 135 – 153 .
- Ching , R P , Nuckley , D J , Hertsted , S M , Eck , M P , Mann , F A and Sun , E A . 2001 . Tensile mechanics of the developing cervical spine . Stapp Car Crash J. , 45 : 329 – 336 .
- Clarke , E C , Appleyard , R C and Bilston , L E . 2007 . Immature sheep spines are more flexible than mature spines: an in vitro biomechanical study . Spine. , 32 : 2970 – 2979 .
- Cummings , P , Wells , J D and Rivara , F P . 2003 . Estimating seat belt effectiveness using matched-pair cohort methods . Accid Anal Prev. , 35 : 143 – 149 .
- Dejeammes , M , Tarri , C , Thomas , C and Kallieris , D . Exploration of biomechanical data towards a better evaluation of tolerance for children involved in automotive accidents . Paper presented at: 28th Stapp Car Crash Conference; Chicago, IL, November 6–7 ,
- de Lange , R , van der Made , R , Feustel , J R , Subbian , T and van Hoof , J . Development and evaluation of MADYMO child occupant dummy models . Paper presented at: 4th North American MADYMO User's Meeting; Detroit, MI, October ,
- Deng , Y C and Fu , J . Simulation and identification of the neck muscle activities during head and neck flexion whiplash . Paper presented at: SAE World Congress; March 11–15 , Detroit, MI
- Deng , Y C and Goldsmith , W . 1987 . Response of a human head/neck/upper-torso replica to dynamic loading—II. Analytical/numerical model . J Biomech. , 20 : 487 – 497 .
- Dibb , AT. 2011 . Pediatric Head and Neck Dynamic Response: A Computational Study , Durham , NC : Duke University . [Ph.D. thesis]
- Dibb , A T , Cutcliffe , H C , Luck , J F , Cox , C A , Myers , B S , Bass , C R , Arbogast , K B , Seacrist , T and Nightingale , R W . Seoul , , South Korea Pediatric head and neck dynamics in frontal impact: Analysis of important mechanical factors and proposed neck performance corridors for six and ten year old ATDs Proceedings of the 23rd International Technical Conference on the Enhanced Safety of Vehicles, May 27–30
- Duncan , J M . 1874 . On the tensile strength of the fresh adult fetus . Br Med J. , 2 : 763 – 764 .
- Dupuis , R , Meyer , F , Deck , C and Willinger , R . 2006 . Three-year-old child neck finite element modelization . Eur J Orthop Surg Traumatol. , 16 ( 3 ) : 193 – 202 .
- Durbin , D R , Bhatia , E Holmes , J H . 2001 . Partners for Child Passenger Safety: a unique child-specific crash surveillance system . Accid Anal Prev. , 33 : 407 – 412 .
- Eleraky , M , Theodore , N , Adams , M , Rekate , H and Sonntag , V . 2000 . Pediatric cervical spine injuries: report of 102 cases and review of the literature . J Neurosurg Spine. , 92 : 12 – 17 .
- Elliott , M R , Kallan , M J , Durbin , D R and Winston , F K . 2006 . Effectiveness of child safety seats vs seat belts in reducing risk for death in children in passenger vehicle crashes . Arch Pediatr Adolesc Med. , 160 : 617 – 621 .
- Gehre , C , Gades , H and Wernicke , P . Objective rating of signals using test and simulation responses . Paper presented at: Enhanced Safety of Vehicles; Stuttgart, Germany, June 15–18 ,
- Golinski , W Z and Gentle , R . 2005 . The influence of seat back rake on ligament loadings in rear-end impact . Proc Inst Mech Eng D J Automobile Eng. , 219 ( 2 ) : 197 – 205 .
- Halman , S I , Chipman , M , Parkin , P C and Wright , J G . 2002 . Are seat belt restraints as effective in school age children as in adults? A prospective crash study . Br Med J. , 324 : 1123 – 1125 .
- Hilker , C E , Yoganandan , N and Pintar , F A . 2002 . Experimental determination of adult and pediatric neck scale factors . Stapp Car Crash J. , 46 : 417 – 429 .
- Houston , D J , Richardson , LEJR and Neeley , G W . 1996 . Mandatory seat belt laws in the states: a study of fatal and severe occupant injuries . Eval Rev. , 20 ( 2 ) : 146 – 159 .
- Iman , R , Campbell , J and Helton , J . 1981 . An approach to sensitivity analysis of computer models. Part I—introduction, input, variable selection and preliminary variable assessment . J Qual Technol. , 13 ( 4 ) : 174 – 183 .
- Iman , R , Helton , J and Campbell , J . 1981 . An approach to sensitivity analysis of computer models: Part II—ranking of input variables, response surface validation, distribution effect and technique synopsis . J Qual Technol. , 13 ( 4 ) : 232 – 240 .
- International Organization for Standardization . 1999 . Road Vehicles— Anthropomorphic Side Impact Dummy—Lateral Impact Response Requirements to Assess the Biofidelity of the Dummy , International Organization for Standardization . Technical Report 9790
- Javouhey , E , Guerin , A C , Gadegbeku , B , Chiron , M and Floret , D . 2006 . Are restrained children under 15 years of age in cars as effectively protected as adults? . Arch Dis Child. , 91 : 304 – 308 .
- Kallieris , D , Barz , J , Schmidt , G , Heess , G and Mattern , R . Comparison between child cadavers and child dummy by using child restraint systems in simulated collisions . Paper presented at: 20th Stapp Car Crash Conference; Dearborn, MI, October 18–20 ,
- Kumaresan , S , Yoganandan , N , Pintar , F A , Maiman , D J and Kuppa , S . 2000 . Biomechanical study of pediatric human cervical spine: a finite element approach . J Biomech Eng. , 122 : 60 – 71 .
- Lee , I H , Choi , H Y , Lee , J H and Han , D C . 2004 . Development of finite element human neck model for vehicle safety simulation . Int J Automot Technol. , 5 ( 1 ) : 33 – 46 .
- Liu , X J and Yang , J K . 2002 . Development of child pedestrian mathematical models and evaluation with accident reconstruction . Traffic Inj Prev. , 3 ( 4 ) : 321 – 329 .
- Loyd , A M , Nightingale , R W Lee , C . 2010 . Pediatric head contours and inertial properties for ATD design . Stapp Car Crash J. , 54 : 167 – 196 .
- Luck , J F , Nightingale , R W Loyd , A M . 2008 . Tensile mechanical properties of the perinatal and pediatric PMHS osteoligamentous cervical spine . Stapp Car Crash J. , 52 : 107 – 134 .
- Luck , J F , Nightingale , R W Song , Y . 2013 . Tensile failure properties of the perinatal, neonatal and pediatric cadaveric cervical spine . Spine. , 38 ( 1 ) : E1 – 12 .
- McGwin , G Jr , Metzger , J , Alonso , J E and Rue , L W III . 2003 . The association between occupant restraint systems and risk of injury in frontal motor vehicle collisions . J Trauma. , 54 ( 6 ) : 1182 – 1187 .
- Mertz , H J , Weber , D A , Nyquist , G W , Lenox , J B and Driscoll , G D . Responses of animals exposed to deployment of various passenger inflatable restraint system concepts for a variety of collision severities and animal positions . Paper presented at: ESV Conference; Kyoto, Japan, November 1–4 ,
- Meyer , F , Bourdet , N , Deck , C , Willinger , R and Raul , J . 2004 . Human neck finite element model development and validation against original experimental data . Stapp Car Crash J. , 48 : 177 – 206 .
- Meyer , F , Roth , S and Willinger , R . 2009 . Child neck FE model development and validation . Int J Hum Fact Model Simul. , 1 ( 2 ) : 244 – 257 .
- Mizuno , K , Iwata , K , Deguchi , T , Ikami , T and Kubota , M . 2005 . Development of a three-year-old child FE model . Traffic Inj Prev. , 6 : 361 – 371 .
- National Highway Traffic Safety Administration . 2007a . Traffic Safety Facts: Children , Department of Transportation, National Highway Traffic Safety Administration .
- National Highway Traffic Safety Administration . 2007b . Traffic Safety Facts: Occupant Protection , Department of Transportation, National Highway Traffic Safety Administration .
- Nightingale , R W , McElhaney , J H , Camacho , D L , Kleinberger , M , Winkelstein , B A and Myers , B S . The dynamic responses of the cervical spine: buckling, end conditions, and tolerance in compressive impacts . Paper presented at: 41st Stapp Car Crash Conference; Orlando, FL, November 13–14 ,
- Nightingale , R W , Winkelstein , B A , Knaub , K E , Richardson , W J , Luck , J F and Myers , B S . 2002 . Comparative strengths and structural properties of the upper and lower cervical spine in flexion and extension . J Biomech. , 35 : 725 – 732 .
- Nightingale , R W , Winkelstein , B A , Van Ee , C A and Myers , B S . Injury mechanisms in the pediatric cervical spine during out-of-position airbag deployments . Paper presented at: 42nd Annual Meeting of the Association for the Advancement of Automotive Medicine; Charlottesnile, VA, October 5–6 ,
- Nuckley , D J , Hertsted , S M , Eck , M P and Ching , R P . 2005 . Effect of displacement rate on the tensile mechanics of pediatric cervical functional spinal units . J Biomech. , 38 : 2266 – 2275 .
- Nuckley , D J , Van Nausdle , J A , Eck , M P and Ching , R P . 2007 . Neural space and biomechanical integrity of the developing cervical spine in compression . Spine. , 32 ( 6 ) : E181 – 187 .
- Östh , J J , Brolin , K , Carlsson , S , Wismans , J and Davidsson , J . 2012 . The occupant response to autonomous braking: a modeling approach that accounts for active musculature . Traffic Inj Prev. , 13 : 265 – 277 .
- Ouyang , J . 2005 . Biomechanical assessment of the pediatric cervical spine under bending and tensile loading . Spine. , 30 ( 24 ) : E716 – 723 .
- Panzer , M. 2006 . Numerical Modelling of the Human Cervical Spine in Frontal Impact , University of Waterloo, Waterloo, Ontario, Canada . [master's thesis]
- Panzer , M B , Fice , J B and Cronin , D S . 2011 . Cervical spine response in frontal crash . Med Eng Phys. , 33 : 1147 – 1159 .
- Partners for Child Passenger Safety . 2008 . Fact and Trend Report , The Children's Hospital of Philadelphia .
- Pedotti , A , Krishnan , V and Stark , L . 1978 . Optimization of muscle-force sequencing in human locomotion . Math Biosci. , 38 : 57 – 76 .
- Pintar , F A , Mayer , R G , Yoganandan , N and Sun , E . Child neck strength characteristics using an animal model . Paper presented at: 44th Stapp Car Crash Conference; Atlanta, GA, November 6–8 ,
- Platzer , P , Jaindl , M Thalhammer , G . 2007 . Cervical spine injuries in pediatric patients . J Trauma. , 62 ( 2 ) : 389–396
- Prasad , P and Daniel , R P . A biomechanical analysis of head, neck, and torso injuries to child surrogates due to sudden torso acceleration . Paper presented at: 28th Stapp Car Crash Conference; Chicago, IL, November 6–7 ,
- Schreck , R M and Patrick , L M . Frontal crash evaluation tests of a five-point harness child restraint . Paper presented at: 19th Stapp Car Crash Conference; San Diego, CA, November 17–19 ,
- Stemper , B D , Yoganandan , N and Pintar , F A . 2004 . Validation of a head–neck computer model for whiplash simulation . Med Biol Eng Comput. , 42 : 333 – 338 .
- Valent , F , McGwin , G Jr , Hardin , W , Johnston , C and Rue , L W III . 2002 . Restraint use and injury patterns among children involved in motor vehicle collisions . J Trauma. , 52 ( 4 ) : 745 – 751 .
- van der Horst , M . 2002 . Human Head Neck Response in Frontal, Lateral and Rear End Impact Loading: Modelling and Validation , Einhoven , , The Netherlands : Technische Universiteit Eindhoven . [Ph.D. thesis]
- Van Ee , C A . 2000 . Tensile Properties of the Human Muscular and Ligamentous Cervical Spine , Durham , NC : Duke University . [Ph.D. thesis]
- Winters , J M . 1995 . How detailed should muscle models be to understand multi-joint movement coordination? . HumMov Sci. , 14 : 401 – 442 .
- Winters , J M and Stark , L . 1985 . Analysis of fundamental human movement patterns through the use of in-depth antagonistic muscle models . IEEE Trans Biomed Eng. , 32 : 826 – 839 .
- Winters , J M and Stark , L . 1988 . Estimated mechanical properties of synergistic muscles involved in movements of a variety of human joints . J Biomech. , 21 : 1027 – 1041 .
- Wismans , J , Maltha , J , Melvin , J W and Stalnaker , R L . Child restraint evaluation by experimental and mathematical simulation . Paper presented at: 23rd Stapp Car Crash Conference; San Diego, CA, October 17–19 ,
- Xu , L , Agaram , V Rouhana , S . Repeatability evaluation of the pre-prototype NHTSA advanced dummy compared to the Hybrid III . Paper presented at: SAE World Congress; Detroit, MI, March 6–9 ,