Abstract
Objective: The shape, size, bone density, and cortical thickness of the thoracic skeleton vary significantly with age and sex, which can affect the injury tolerance, especially in at-risk populations such as the elderly. Computational modeling has emerged as a powerful and versatile tool to assess injury risk. However, current computational models only represent certain ages and sexes in the population. The purpose of this study was to morph an existing finite element (FE) model of the thorax to depict thorax morphology for males and females of ages 30 and 70 years old (YO) and to investigate the effect on injury risk.
Methods: Age- and sex-specific FE models were developed using thin-plate spline interpolation. In order to execute the thin-plate spline interpolation, homologous landmarks on the reference, target, and FE model are required. An image segmentation and registration algorithm was used to collect homologous rib and sternum landmark data from males and females aged 0–100 years. The Generalized Procrustes Analysis was applied to the homologous landmark data to quantify age- and sex-specific isolated shape changes in the thorax. The Global Human Body Models Consortium (GHBMC) 50th percentile male occupant model was morphed to create age- and sex-specific thoracic shape change models (scaled to a 50th percentile male size). To evaluate the thoracic response, 2 loading cases (frontal hub impact and lateral impact) were simulated to assess the importance of geometric and material property changes with age and sex.
Results: Due to the geometric and material property changes with age and sex, there were observed differences in the response of the thorax in both the frontal and lateral impacts. Material property changes alone had little to no effect on the maximum thoracic force or the maximum percent compression. With age, the thorax becomes stiffer due to superior rotation of the ribs, which can result in increased bone strain that can increase the risk of fracture. For the 70-YO models, the simulations predicted a higher number of rib fractures in comparison to the 30-YO models. The male models experienced more superior rotation of the ribs in comparison to the female models, which resulted in a higher number of rib fractures for the males.
Conclusion: In this study, age- and sex-specific thoracic models were developed and the biomechanical response was studied using frontal and lateral impact simulations. The development of these age- and sex-specific FE models of the thorax will lead to an improved understanding of the complex relationship between thoracic geometry, age, sex, and injury risk.
Introduction
Motor vehicle crashes (MVCs) are a serious public health concern that resulted in more than 2,000,000 injured occupants and more than 33,000 fatalities in 2012 (National Center for Statistics and Analysis Citation2013). In MVCs, thoracic injury ranks second only to head injury in terms of the number of fatalities and serious injuries, the body region most often injured, and the overall economic cost (Cavanaugh Citation2002; Ruan et al. Citation2003).
Age- and sex-related morphologic changes in the thorax may affect the injury tolerance, especially in at-risk populations such as pediatrics and the elderly. With a growing elderly population of adults aged 65+ years, which is projected to increase to 20% of the population by 2040, it is critical to understand the biomechanics of the human thorax with age because the elderly possess an increased risk of mortality and morbidity due to increased fragility and frailty (Finelli et al. Citation1989; Hanna and Hershman Citation2009; Perdue et al. Citation1998; Shorr et al. Citation1989; Stitzel et al. Citation2008; Vincent and Velkoff Citation2010). In addition to age, there are structural and morphological differences in the thorax with sex (Shi et al. Citation2014; Weaver, Schoell, Nguyen, et al. Citation2014; Weaver, Schoell, and Stitzel Citation2014). Studies have shown that females are more susceptible to severe injuries in comparison to males and analysis of real-world frontal MVCs determined that females and the elderly are the 2 most vulnerable groups of the entire population (Hu et al. Citation2012; Wang and Yang Citation1998; Welsh and Lenard Citation2001). Variations in thoracic size, shape, bone density, and cortical thickness with age and sex will result in different injury tolerances to traumatic insults. In order to prevent and mitigate injuries, a better understanding of the biomechanics and injury mechanisms of the thorax is needed.
Computational modeling has emerged as a powerful and versatile tool to assess injury risk and improve the effectiveness of vehicle safety systems. Current finite element (FE) models are limited to certain ages and sexes in the population. The full spectrum of ages was not investigated in any previous studies, and females in particular are underrepresented (Deng et al. Citation1999; El-Jawahri 2010; Gayzik et al. Citation2006; Ito et al. Citation2009; Kent et al. Citation2005; Kimpara et al. Citation2005; Lizee et al. Citation1998; Mattrey et al. Citation2008; Plank Citation1998; Ruan et al. Citation2003; Song et al. Citation2009; Tamura et al. 2005; Vezin and Verriest 2005; Wang and Yang 1998). Though in theory, FE models can represent all ages and sexes, a limitation of FE modeling is the time-consuming process to develop subject-specific meshes. In order to accelerate the development of FE models, model morphing can be used to accurately and efficiently generate models of all ages and sexes. The purpose of this study is to develop and validate age- and sex-specific FE models of the thorax to accurately model thorax morphology and mechanics for males and females of ages 30 and 70 years old (YO). The biomechanical response of an individual of a given age and sex will be studied through simulations of various frontal and lateral impacts to assess the importance of geometric and material property changes with age and sex.
Methods
Model Development
The selection of 30- and 70-YO models was based on previous recommendations that defined a young adult group between 16 and 35 YO and an elderly group 66 YO and older (Zhou et al. Citation1996). Previous age-specific models used similar ages of 35 and 75 YO to define an adult and elderly model (El-Jawahri 2010; Ito et al. Citation2009).
Geometric Changes with Aging
Geometric changes with aging were implemented by changing the shape of the thorax. Development of age- and sex-specific FE meshes involves morphing an existing FE model of the thorax using radial basis function interpolation, specifically with a thin-plate spline as the basis function. The thin-plate spline is a smooth function that interpolates the connections between the nodes while minimizing the amount of change in landmark positions. The thin-plate spline procedure detailed by Bookstein et al. (Citation1999) involves the calculation of a bending energy matrix, L, and a partial warp score matrix, W, to determine the interpolation function and coefficients to map landmark coordinates on a reference structure to homologous landmark coordinates specified on a target structure. Subsequently, the calculated interpolation function and coefficients can be applied to other coordinates associated with the reference; that is, the nodal coordinates of the FE model (Bookstein et al. Citation1999). Thin-plate spline regularization was employed to relax interpolation requirements so that the resulting surface did not have to pass exactly through all of the control points. Regularization is controlled by the regularization parameter, λ, which was adjusted to achieve the highest element quality (Donato and Belongie Citation2002).
In order to execute the thin-plate spline interpolation, homologous landmarks on the reference, target, and FE model are required. The reference and FE model homologous landmarks were derived from the Global Human Body Models Consortium (GHBMC) v4.2 average male occupant model. The GHBMC is representative of a 50th percentile male (M50) and was based on medical images of a 26-YO individual (height, 174.9 cm; weight, 78.6 ± 0.77 kg; and body mass index [BMI], 25.7 ± 0.25; Gayzik et al. Citation2011; Vavalle et al. Citation2013). A simplified full-body model using the GHBMC M50 v4.2 was developed by combining the detailed thorax, detailed abdomen, rigid head, simplified neck, and rigid lower extremity. An image segmentation and registration algorithm was previously used to collect homologous rib and sternum landmark data from males and females aged 0–100 years (Weaver, Schoell, Nguyen, et al. Citation2014; Weaver, Schoell, and Stitzel Citation2014). The Generalized Procrustes Analysis was applied to the homologous landmark data to quantify age- and sex-specific size and shape changes, as well as isolated shape changes in the thorax. For the purposes of this study, the isolated shape change models representative of specific ages of males and females were used to define the target homologous landmarks. The isolated shape change models for both the males and females are scaled to a 50th percentile male size, which allows for the incorporation into the simplified full body GHBMC M50 v4.2. The combined size and shape models, not used in this study, are not scaled to a particular percentile but instead represent the percentile size of the subjects of a given age and sex from the sample population analyzed (Weaver, Schoell, and Stitzel et al. Citation2014).
Although homologous landmark data were only collected for the ribs and sternum, associated structures such as the costal cartilage, intercostal muscles, spine, and thoracic organs as well as the external body surface geometry are morphed along with the skeletal structures due to the connection of the elements. In order to reduce computational time of the morphing process, a uniform 5% subsample of the landmarks from the reference and target was selected, resulting in a reduction to 9,524 landmarks. The element quality of the reference and morphed FE models was analyzed by comparing the Jacobian (>0.3), warpage angle (<50°), and aspect ratio (<8). Though the morphing process is automated, manual mesh cleanup is required to ensure that element quality standards are met. An additional 54 reference and target homologous landmarks representative of external anthropometry of the GHBMC M50 model were incorporated as control points to maintain the 50th percentile size during the morphing process. To compare geometric differences across the models, the rib angles of each rib were measured from the superior-most posterior point of the rib to the superior-most anterior point of the rib relative to a straight vertical normal line.
Table 1 Material properties for the rib cortical bone
Material Property Changes with Aging
To evaluate the role of material property changes with age, a technique developed by Golman et al. (Citation2014) was implemented to age the ribs by adjusting the ultimate plastic strain. A statistically significant correlation between age and ultimate strain based on 2 previous studies was developed as given in EquationEq. (1)(1) (Kemper et al. Citation2005, 2007). Though sex may be a significant factor in the material properties of human rib cortex, current literature has found no statistically significant differences with sex in material properties obtained from coupon testing (Kemper et al. Citation2005; Lindahl and Lindgren Citation1967; Stitzel et al. Citation2003; Yeni et al. Citation1998). Therefore, sex was not controlled for and the simulations for the single factor material properties were only performed for the 2 ages and 2 loading cases.
(1)
The ribs in the GHBMC M50 v4.2 are modeled as a piecewise linear plasticity material with a failure strain. Rib fracture is predicted by deleting elements exceeding the prescribed failure plastic strain in the cortical bone (Li et al. Citation2009). The material properties for the cortical bone of the ribs for the GHBMC M50 v4.2 model and the age-specific models are provided in . As expected, the ultimate plastic strain decreases with age, resulting in an increased risk of rib fracture for similar loading conditions. Abbreviated Injury Scale (AIS) 98 injury severity for each simulation was determined using the number of predicted rib fractures (Association for the Advancement of Automotive Medicine Citation2001). The effects of age-related changes in the thoracic soft tissue were not studied due to limited data in the literature (Kent et al. Citation2005). Therefore, the material properties of the surrounding soft tissue were constant across the models and representative of what is currently implemented in the GHBMC M50 v4.2 model.
Table 2 Simulation test matrix for age- and sex-specific thorax shape models incorporated into a simplified GHBMC full body model
Simulation Validation
All simulations were run using LS-Dyna (v6.1.1 rev78769, LSTC, Livermore, CA) on a Linux Red Hat computational cluster maintained at Wake Forest University (the DEAC cluster). The simplified full-body model GHBMC M50 v4.2 was developed for simulation purposes and allowed for faster run time in order to perform a greater number of simulations than with the full detailed model. The simplified GHBMC M50 v4.2 model contains 747,000 nodes, 1.6 million elements, and 378 parts and represents a weight of 74.3 kg. The simplified GHBMC M50 v4.2 model was validated against the following experimental testing conditions to assess numerical stability and overall responses of the model: frontal hub impact (Lebarbé and Petit 2012), lateral impact (Kemper et al. Citation2008), lateral shoulder impact (Koh et al. Citation2005), abdominal bar impact (Hardy et al. Citation2001), and oblique abdominal hub impact (Viano Citation1989). Overall responses of the simplified model were comparable to the experimental data as well as the GHBMC M50 v4.2. The simplified full-body model responses resulted in slight deviations from the GHBMC M50 v4.2 due to the simplifications of the head and neck.
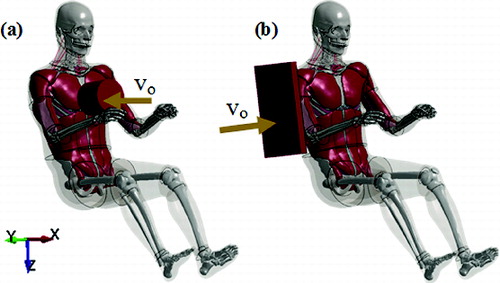
To evaluate the thoracic response, 2 loading cases (frontal hub impact and lateral impact) were simulated to assess the importance of geometric and material property changes with age and sex (). The frontal hub impact was conducted at 6.7 m/s with a 23.4 kg cylindrical impactor striking the sternum at the fourth rib interspace. The raw data were mass-scaled to 75 kg using the method described by Eppinger and were filtered at 300 Hz with an SAE class filter (Eppinger et al. Citation1978). The target corridors were developed for specific masses representative of a 50th percentile male. Because the mass of the morphed models was lower than the mass used to develop the target corridors, the predicted model response was mass scaled accordingly to allow for a consistent comparison. Deflection was normalized by chest depth and was calculated as a percent to compare models. Chest depth was calculated by averaging the coordinates of external nodes on the left and right front chest and calculating the distance between this averaged chest location and a node on T8. The lateral impact was conducted at 12.0 m/s with a 23.4 kg flat plate impactor striking the shoulder, arm, and ribs. The simulation corresponds to the 45° arm angle destructive testing conducted by Kemper et al. (2008). The raw data were filtered at 600 Hz with an SAE class filter and mass-scaled to 76 kg.
The GHBMC M50 v4.2 is preprogrammed with upper, middle, and lower chestbands that are each composed of 32 nodes (Hayes et al. Citation2014). Chestbands were placed around the circumference of the chest approximately at the level of the fourth, sixth, and eight ribs. Model chestband results were analyzed by using the postprocessor LS-PrePost (v4.1, LSTC, Livermore, CA), and MATLAB (R 10, The Mathworks, Natick, MA) for each simulation. LS-PrePost was used to visualize chestband responses and obtain Cartesian coordinates of each chestband's nodes over time. The coordinates of the chestband were sampled at a rate of 10,000 Hz.
A total of 20 simulations were performed (). The simulations included 2 ages and 2 sexes with 2 loading cases. Single factors studied included geometric and material property changes with age and sex. Multiple factors included the combination of geometric and material property changes with age and sex.
Results
shows the morphing results for the 30- and 70-YO male and female. Overall, the ribs 1–12 rotated superiorly an average of 2.28° in males and 1.46° in females from 30 to 70 YO. The chest depth of the simplified GHBMC M50 v4.2 model was 240.7 mm. The chest depth of the 30- and 70-YO male models was 237.1 and 245.1 mm, respectively. The chest depth of the 30- and 70-YO female models was 236.7 and 241.2 mm, respectively. The mass of the 30- and 70-YO male models was 73.8 and 74.0 kg, respectively. The mass of the 30- and 70-YO female models was 73.3 kg for both ages. As a result, all of the models were within 1.4% of the baseline GHBMC M50 v4.2 simplified model mass. The regularization parameter used to relax the interpolation requirements resulted in slight deviations in the shape change models derived from the Weaver, Schoell, and Stitzel et al. (Citation2014) data. However, with no relaxation, the mesh quality substantially deteriorates.
Frontal Hub Impact
The model outputs of the frontal hub impact simulations when the geometric, material properties, and combined geometric and material properties are varied with age and sex are shown in Figure A1 (see online supplement). The force versus deflection in the frontal hub impact simulations can be compared to the Lebarbé corridors and experimental average in Figure A1 (Lebarbé and Petit 2012). The responses from the 30-YO and 70-YO male and female models were generally within the corridors. The percent difference of the 70-YO models relative to the 30-YO models for the maximum thoracic force (Fmax) and the maximum percent compression are summarized in by age and sex for geometric changes only, material property changes only, and combined geometric and material property changes. The 70-YO female geometric model experienced up to a 5.95% decrease in Fmax and the 70-YO male geometric model experienced up to 6.54% decrease in the maximum percent compression.
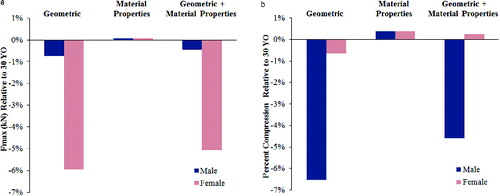
For Fmax, the models with geometric changes only and models with geometric and material property changes showed the most variation in the response. Material property changes alone had little to no effect on Fmax with an average value of 4.3 kN across all ages and sexes. The 30-YO models tended to experience slightly higher Fmax values in comparison to the 70-YO models. For the maximum percent compression, the models with geometric changes only and models with geometric and material property changes also showed the most variation in the response. The percent compression was slightly higher for the 30-YO models in comparison to the 70-YO models. A comparison of the strain history of the 30-YO and 70-YO male geometric and material property models is provided in . During the course of the simulation, the 70-YO model experienced higher strain values in comparison to the 30-YO model. A comparison of the chestband contours at the maximum deflection state is provided in Figure A2 (see online supplement) for each simulation case. Slight differences in the chestband contour response are shown for the models with geometric changes and models with geometric and material property changes.
Lateral Impact
The model outputs of the lateral impact simulations when the geometric, material properties, and combined geometric and material properties are varied with age and sex are shown in Figure A3 (see online supplement). The impactor force versus time can be seen compared to the experimental results in Figure A3. The first peak force represents the inertial and compressive response of the arm and shoulder. The second peak force represents the inertial and compressive response of the thorax. The percent difference of the 70-YO models relative to the 30-YO models for Fmax is summarized in by age and sex for geometric changes only, material property changes only, and combined geometric and material property changes. The 70-YO male geometric and material property model experienced an increase of 11.38% in Fmax relative to the 30-YO male geometric and material property model.
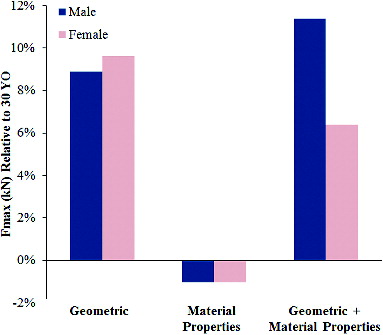
Fmax for the experimental data of the lateral impact tests was 11.6 ± 3.2 kN. The values predicted for the geometric changes only and combined geometric and material property changes models are comparable to the experimental data. The material property changes model had little to no effect on Fmax. The 70-YO models tended to have slightly higher Fmax values in comparison to the 30-YO models. In addition, the males tended to have slightly higher Fmax values in comparison to the females. A comparison of the strain history of the 30-YO and 70-YO male geometric and material property models is provided in Figure 4b. During the course of the simulation, the 70-YO model experienced higher strain values in comparison to the 30-YO model. A comparison of the chestband contours at the maximum deflection state is provided in Figure A4 (see online supplement) for each simulation case. Slight differences in the chestband contour response are shown for all 3 factors. The chestband contours of the 30-YO male model deviated from the other models due to slightly different loading of the arm during the lateral impact.
Fracture Prediction
A summary of the predicted rib fractures for the frontal hub impact and lateral impact tests is provided in . In the experimental data for the frontal hub impact tests, 8 subjects with a mean and standard deviation age of 55.5 ± 23.4 sustained 9.4 ± 7.2 rib fractures. The computational models underpredicted the number of rib fractures in comparison to the experimental data. The combined geometric and material property changes models resulted in the most rib fractures, with 4 fractures predicted for the 70-YO male and female models, which correlates to an AIS 2 injury. The 30-YO models predicted 0 to 2 fractures resulting in AIS 0 to AIS 2 injuries. The 70-YO models predicted 2 to 4 fractures resulting in AIS 2 injuries.
In the experimental data for the lateral impact tests, there were 2 subjects (ages 47 and 61) who sustained 21 rib fractures on the impacted side and 1 to 2 rib fractures on the nonimpacted side. The models tended to underpredict the number of rib fractures in comparison to the experimental data. The 70-YO material property changes models and combined geometric and material property changes models resulted in the most rib fractures resulting in AIS 3 injuries. The 30-YO models predicted 5 to 9 fractures resulting in AIS 3 injuries. The 70-YO models predicted 8 to 19 fractures resulting in AIS 3 injuries. In addition, the 70-YO models matched the experimental data with the 2 rib fractures predicted on the nonimpacted side.
Discussion
In this study, age- and sex-specific FE models of the thorax were developed for a 30-YO and 70-YO male and female. To evaluate the thoracic response, 2 loading cases (frontal hub impact and lateral impact) were simulated to assess the importance of geometric and material property changes with age and sex. Geometric changes were implemented based on statistical data characterizing the shape changes of thorax morphology for males and females of ages 0–100. A radial basis function interpolation using a thin-plate spline basis function was used to morph an existing FE model to specific ages and sexes. Consistent with previous literature, the 70-YO models possess increased thoracic kyphosis, as shown in , due to the covariance of rib geometry and spine posture (Gayzik et al. Citation2008; Weaver, Schoell, and Stitzel et al. Citation2014). In addition, shows the increased roundedness as well as the upward rotation of ribs, which influences the biomechanical response of the thorax. Previous studies that have explored geometric changes only varied rib angles (El-Jawahri et al. Citation2010; Kent et al. Citation2005). The detailed homologous landmark data from the Weaver, Schoell, and Stitzel et al. study not only captures the rib angle changes but captures the full 3-dimensional changes in the rib cage. The shape models were utilized in order to compare full-body simulations across certain ages and sexes. However, the female shape models were scaled to a 50th percentile male size and therefore do not represent the response of an average female. The size and shape models, not used in this study, are not scaled to a particular percentile. Models derived from this data would be of the thoracic structure alone and therefore only local simulations could be performed. Though the shape models are limited in their ability to realistically represent real-world occupants, the size and shape models would not provide much useful information if only local simulations could be performed.
Material property changes were implemented using techniques derived from literature to age the ribs by adjusting the ultimate plastic strain. Overall, changes in geometry and material properties resulted in differing responses across age and sex. However, material property changes alone had little to no effect on the overall response of the thorax. These results agree with previous findings in the literature that the definition of the rib cortical bone ultimate strain has little effect on the output of the model (Forman et al. Citation2012). Though the results showed minor differences across ages and sex in terms of global parameters such as Fmax and percent compression, there were more noticeable differences on a local and tissue level in regards to the strain values and number of rib fractures across the ages and sexes.
For the frontal hub impact simulations, the 30-YO models tended to experience slightly higher Fmax values in comparison to the 70-YO models. Fmax is affected by mass recruitment as well as thoracic stiffness. The geometric changes with age contribute to the differences in thoracic stiffness. For ages 20–60, there is an increase in thoracic kyphosis and superior rotation of the ribs. For ages 60 and older, there is an increase in thoracic kyphosis, an inferior rotation of the upper ribs, and a superior rotation of the lower ribs (Weaver, Schoell, and Stitzel et al. Citation2014). With the superior rotation of the ribs that occurs from age 30 to 70, there is less joint rotation, which results in a stiffer thoracic response. There are slight differences in Fmax by sex, with the most apparent difference occurring at age 30. These differences are most likely the result of the slight geometric and structural variations due to sex. Similar trends in thoracic stiffness have been reported in the literature (Agnew et al. 2013; Maltese et al. Citation2008).
Additionally for the frontal hub impact simulations, the percent compression was slightly higher for the 30-YO models in comparison to the 70-YO models. Younger ages tend to have more compliant thoraxes resulting in more chest deflection. With age, the thorax becomes stiffer, which can result in increased bone strain that can increase the risk of fracture. The 70-YO models predicted more rib fractures than the 30-YO models. However, this did not have any effect on the amount of deflection during the simulation.
For the lateral impact simulations, the 70-YO models tended to have slightly higher Fmax values in comparison to the 30-YO models. Fmax is greater for older ages possibly due to the increased roundedness of the rib cage with age. The 70-YO models also predicted more rib fractures than the 30-YO models but once again this had no effect on Fmax during the simulation.
For both impact simulations, the number of rib fractures predicted was analyzed. Overall, with increasing age there was an increase in the number of rib fractures. Due to the decrease in the ultimate plastic strain with age, the 70-YO models predicted more rib fractures than the 30-YO models. The material properties are the same for both males and females due to limited experimental data. As a result, the difference in rib fractures among males and females of the same age is solely due to the changes in geometry. The superior rotation of the ribs with age results in a more horizontally oriented rib. As a result, loading in the anterior–posterior direction results in increased deflection and an increased risk of rib fracture (Kent et al. Citation2005). The 70-YO male models experienced more superior rotation of the ribs as well as an expansion in the lateral rib cage width, which resulted in slight differences in the prediction of rib fractures in comparison to the female 70-YO model. For the 30-YO models, the males experienced slightly more superior rotation of the ribs in comparison to the females, which resulted in slightly more predicted rib fractures for the males.
However, it should be noted that prediction of rib fractures is very complex because various factors including age, bone mineral density, and preexisting conditions can result in different outcomes. In addition, rib fractures can be influenced by the properties of the surrounding soft tissue, which were not changed due to limited experimental data. For the frontal hub impact, the number of predicted fractures was much lower than the experimental results. However, this is most likely the result of age-related changes to the surrounding structures, which would result in more rib fractures in the older cadaver models for the experimental work. The age-related changes in the surrounding structures of the FE models were not controlled for and therefore fewer rib fractures were predicted. For the lateral hub impact, the prediction level was much closer to the experimental results because the properties of the surrounding structures have less of an effect on fracture prediction due to the lateral loading direction.
There are a few limitations associated with the study. In addition to age and sex, BMI affects rib geometry (Shi et al. Citation2014). BMI was not controlled for in the development of the statistical models used in this study and therefore was not accounted for in the development of the rib FE models. The goal of this study was focused on the changes in the rib cage and not the external body shape. However, these models can serve as a baseline for future work to include external body shape changes. Another limitation of the study involves the female shape models being scaled to a 50th percentile male size. This will be addressed in the future when the GHBMC releases female-specific models in which the age-specific rib cages of the females from this study can be incorporated to accurately represent an average female. Another limitation of the morphing technique is that it does not account for variability in cortical bone thickness. Future work includes incorporation of cortical thickness differences with age and sex based on medical imaging data. Incorporation of material property changes with age also serves as a limitation. Currently in the literature, there are limited data related to the material property changes with age and sex. Refinement of these models can be performed when more extensive age and sex data become available. Similarly, the validation of these models poses a limitation because most experimental work is limited primarily to older male cadavers. Additional comparison of these models can be performed if more age- and sex-specific experimental work is completed. The current study focused only on the responses of 30- and 70-YO males and females. Future work includes development and simulation of the models across the full age and sex spectrum to characterize the thoracic response for an entire population. Future work could also consider the effects of the residual variance from the regression equations for shape to characterize population variability, but this was beyond the scope of the current study.
Understanding the age- and sex-specific biomechanics of the thorax will lead to advancements in vehicle safety design, such as restraint systems that could be tailored to an occupant's age and sex. Assessment of injury risk and effectiveness of the performance of vehicle safety systems can be performed using computational models. Current FE models are limited to certain ages and sexes in the population. Development of age- and sex-specific FE models of the thorax will provide valuable tools for evaluating vehicle crashworthiness and understanding variations in thoracic injury patterns due to MVCs across populations. The goal of this research is to develop the age- and sex-specific FE models to predict, prevent, and mitigate thoracic injuries for the whole population with specific interest in at-risk populations, including pediatrics and the elderly.
Morphed age- and sex-specific FE models of the thorax were developed using thin-plate spline interpolation. The advantages of this approach include smooth interpolation between the reference and target geometry and a time-efficient method of developing a large number of FE models in comparison to the development of patient-specific models. The age- and sex-specific models developed in this study incorporate detailed changes in geometric and material property changes based on a large population sample. Geometric and material property changes with age and sex result in different responses of the thorax. The development of these age- and sex-specific FE models of the thorax will lead to an improved understanding of the complex relationship between thoracic geometry, age, sex, and injury risk. The improved understanding gained from these models will aid in changes in restraint design and use to not only reduce injuries but also save lives.
Funding
Funding was provided by the National Highway Traffic Safety Administration under Cooperative Agreement Number DTN22–09-H-00242. Views expressed are those of the authors and do not represent the views of NHTSA.
Supplemental Materials
Supplemental data for this article can be accessed on the publisher's website
Appendix
Download Zip (893.2 KB)References
- Agnew AM, Kang Y-S, Moorhouse K, Herriott R, Bolte JH. Age-related changes in stiffness in human ribs. Paper presented at: IRCOBI; September 2013; Gothenburg, Sweden.
- Association for the Advancement of Automotive Medicine. The Abbreviated Injury Scale (AIS) Update 98 Manual. Des Plaines, IL: AAAM; 2001.
- Bookstein F, Schäfer K, Prossinger H, et al. Comparing frontal cranial profiles in archaic and modern Homo by morphometric analysis. Anat Rec. 1999;257(6):217–224.
- Cavanaugh JM. Biomechanics of thoracic trauma. In: Nahum AM, Melvin JW, eds. Accidental Injury: Biomechanics and Prevention. 2nd ed. New York, NY: Springer-Verlag; 2002:374–404.
- Deng Y-C, Kong W, Ho H. Development of a Finite Element Human Thorax Model for Impact Injury Studies. Warrendale, PA: SAE; 1999. SAE Technical Paper No. 1999–01–0715.
- Donato G, Belongie S. Approximate thin plate spline mappings. In: Proceedings of the 7th European Conference on Computer Vision (ECCV 2002), Part III, LNCS 2352; Heyden A, Sparr G, Nielsen M, Johansen P, editors. New York: Springer; 2002:21–31.
- El-Jawahri RE, Laituri TR, Ruan JS, Rouhana SW, Barbat SD. Development and validation of age-dependent FE human models of a mid-sized male thorax. Stapp Car Crash J. 2010;54:407–430.
- Eppinger RH, Augustyn K, Robbins DH. Development of a Promising Universal Thoracic Trauma Prediction Methodology. Warrendale, PA: SAE; 1978. SAE Technical Paper No. 780891.
- Finelli FC, Jonsson J, Champion HR, Morelli S, Fouty WJ. A case control study for major trauma in geriatric patients. J Trauma. 1989;29:541–548.
- Forman JL, Kent RW, Mroz K, Pipkorn B, Bostrom O, Segui-Gomez M. Predicting rib fracture risk with whole-body finite element models: development and preliminary evaluation of a probabilistic analytical framework. Annu Proc Assoc Adv Automot Med. 2012;56:109–124.
- Gayzik FS, Loftis KL, Slice DE, Stitzel JD. A finite element study of age-based size and shape variation of the human rib cage. Biomed Sci Instrum. 2006;42:19–24.
- Gayzik FS, Moreno D, Geer C, Wuertzer S, Martin R, Stitzel J. Development of a full body CAD dataset for computational modeling: a multi-modality approach. Ann Biomed Eng. 2011;39:2568–2583.
- Gayzik FS, Yu MM, Danelson KA, Slice DE, Stitzel JD. Quantification of age-related shape change of the human rib cage through geometric morphometrics. J Biomech. 2008;41:1545–1554.
- Golman AJ, Danelson KA, Miller LE, Stitzel JD. Injury prediction in a side impact crash using human body model simulation. Accid Anal Prev. 2014;64:1–8.
- Hanna R, Hershman L. Evaluation of Thoracic Injuries Among Older Motor Vehicle Occupants. Washington, DC: NHTSA; 2009. DOT HS 811 101.
- Hardy WN, Schneider LW, Rouhana SW. Abdominal impact response to rigid-bar, seatbelt, and airbag loading. Stapp Car Crash J. 2001;45:1–32.
- Hayes AR, Vavalle NA, Moreno DP, Stitzel JD, Gayzik FS. Validation of simulated chestband data in frontal and lateral loading using a human body finite element model. Traffic Inj Prev. 2014;15(2):181–186.
- Hu J, Rupp JD, Reed MP. Focusing on vulnerable populations in crashes: recent advances in finite element human models for injury biomechanics research. Journal of Automotive Safety and Energy. 2012;3:.
- Ito O, Dokko Y, Ohashi K. Development of Adult and Elderly FE Thorax Skeletal Models. Warrendale, PA: SAE; 2009. SAE Technical Paper No. 2009–01–0381.
- Kemper AR, McNally C, Kennedy EA, Manoogian SJ, Duma SM. The influence of arm position on thoracic response in side impacts. Stapp Car Crash J. 2008;52:379–420.
- Kemper AR, McNally C, Kennedy EA, et al. Material properties of human rib cortical bone from dynamic tension coupon testing. Stapp Car Crash J. 2005;49:199–230.
- Kemper AR, McNally C, Pullins CA, Freeman LJ, Duma SM, Rouhana SM. The biomechanics of human ribs: material and structural properties from dynamic tension and bending tests. Stapp Car Crash J. 2007;51:235–273.
- Kent R, Lee SH, Darvish K, et al. Structural and material changes in the aging thorax and their role in crash protection for older occupants. Stapp Car Crash J. 2005;49:231–249.
- Kimpara H, Lee JB, Yang KH, et al. Development of a three-dimensional finite element chest model for the 5(th) percentile female. Stapp Car Crash J. 2005;49:251–269.
- Koh S-W, Cavanaugh JM, Mason MJ, et al. Shoulder injury and response due to lateral glenohumeral joint impact: an analysis of combined data. Stapp Car Crash J. 2005;49:291–322.
- Lebarbé M, Petit P. New biofidelity targets for the thorax of a 50th percentile adult male in frontal impact. Paper presented at: IRCOBI; September 2012; Dublin, Ireland.
- Li Z, Kindig MW, Kerrigan JR, et al. Rib fractures under anterior–posterior dynamic loads: experimental and finite-element study. J Biomech. 2010;43:228–234.
- Lindahl O, Lindgren AG. Cortical bone in man 1. Variation of the amount and density with age and sex. Acta Orthop. 1967;38(1–4):133–140.
- Lizee E, Robin S, Song E, et al. Development of a 3D Finite Element Model of the Human Body. Warrendale, PA: SAE; 1998. SAE Technical Paper No. 983152.
- Maltese MR, Castner T, Niles D, et al. Methods for determining pediatric thoracic force–deflection characteristics from cardiopulmonary resuscitation. Stapp Car Crash J. 2008;52:83–105.
- Mattrey RF, Fournier A, Corbeil J, et al. Development and validation of subject-specific finite element models for blunt trauma study. J Biomech Eng. 2008;130(2):021022.
- National Center for Statistics and Analysis. Traffic Safety Facts: 2012 Motor Vehicle Crashes: Overview. Washington, DC: NHTSA; 2013. DOT HS 811 856.
- Perdue PW, Watts DD, Kaufmann CR, Trask AL. Differences in mortality between elderly and younger adult trauma patients: geriatric status increases risk of delayed death. J Trauma. 1998;45:805–810.
- Plank GR. Analytical Investigation of Driver Thoracic Response to Out of Position Airbag Deployment. Warrendale, PA: SAE;1998. SAE Technical Paper No. 983165.
- Ruan J, El-Jawahri R, Chai L, Barbat S, Prasad P. Prediction and analysis of human thoracic impact responses and injuries in cadaver impacts using a full human body finite element model. Stapp Car Crash J. 2003;47:299–321.
- Shi X, Cao L, Reed MP, Rupp JD, Hoff CN, Hu J. A statistical human rib cage geometry model accounting for variations by age, sex, stature and body mass index. J Biomech. 2014;47:2277–2285.
- Shorr RM, Rodriguez A, Indeck MC, Crittenden MD, Hartunian S, Cowley RA. Blunt chest trauma in the elderly. J Trauma. 1989;29:234–237.
- Song E, Trosseille X, Baudrit P. Evaluation of thoracic deflection as an injury criterion for side impact using a finite elements thorax model. Stapp Car Crash J. 2009;53:155–191.
- Stitzel JD, Cormier JM, Barretta JT, et al. Defining regional variation in the material properties of human rib cortical bone and its effect on fracture prediction. Stapp Car Crash J. 2003;47:243–265.
- Stitzel JD, Kilgo PD, Danelson KA, Geer CP, Pranikoff T, Meredith JW. Age thresholds for increased mortality of three predominant crash induced head injuries. Annu Proc Assoc Adv Automot Med. 2008;52:235–244.
- Tamura A, Watanabe I, Miki K. Elderly human thoracic FE model development and validation. Paper presented at: 19th ESV Conference; 2005; Washington, DC.
- Vavalle NA, Moreno DP, Rhyne AC, Stitzel JD, Gayzik FS. Lateral impact validation of a geometrically accurate full body finite element model for blunt injury prediction. Ann Biomed Eng. 2013;41:497–512.
- Vezin P, Verriest JP. Development of a set of numerical human models for safety. Paper presented at: 19th ESV Conference; 2005; Washington, DC.
- Viano DC. Biomechanical Responses and Injuries in Blunt Lateral Impact. Warrendale, PA: SAE; 1989. SAE Technical Paper No. 892432.
- Vincent GK, Velkoff VA. The Next Four Decades: The Older Population in the United States: 2010 to 2050. Washington, DC: US Department of Commerce, Economics and Statistics Administration, US Census Bureau; 2010.
- Wang K-C, Yang K. The development of a finite element human thorax model. Paper presented at: Advances in Bioengineering, the 1998 ASME International Mechanical Engineering Congress and Explosions; 1998; New York, NY.
- Weaver AA, Schoell SL, Nguyen CM, Lynch SK, Stitzel JD. Morphometric analysis of variation in the sternum with sex and age. J Morphol. 2014;275:1284–1299.
- Weaver AA, Schoell SL, Stitzel JD. Morphometric analysis of variation in the ribs with age and sex. J Anat. 2014;225:246–261.
- Welsh R, Lenard J. Male and female car drivers—difference in collision and injury risks. Annu Proc Assoc Adv Automot Med. 2001;45:73–91.
- Yeni Y, Brown C, Norman T. Influence of bone composition and apparent density on fracture toughness of the human femur and tibia. Bone. 1998;22:79–84.
- Zhou Q, Rouhana SW, Melvin JW. Age effects on thoracic injury tolerance. Stapp Car Crash J. 1996;40:137–148.