Abstract
Objective: The aim of this study was to investigate the possible effects of age-related intracranial changes on the potential outcome of diffuse axonal injuries and acute subdural hematoma under rotational head loading.
Methods: A simulation-based parametric study was conducted using an updated and validated finite element model of a rat head. The validation included a comparison of predicted brain cortex sliding with respect to the skull. Further, model material properties were modified to account for aging; predicted tissue strains were compared with experimental data in which groups of rats in 2 different lifecycle stages, young adult and mature adult, were subjected to rotational trauma. For the parameter study, 2 age-dependent factors—brain volume and region-specific brain material properties—were implemented into the model. The models young adult and old age were subjected to several injurious and subinjurious sagittal plane rotational acceleration levels.
Results: Sequential analysis of the simulated trauma progression indicates that an increase in acute subdural hematoma injury risk indicator occurs at an early stage of the trauma, whereas an increase in diffuse axonal injury risk indicators occurs at a later stage. Tissue stiffening from young adult to mature adult rats produced an increase in strain-based thresholds accompanied by a wider spread of strain distribution toward the rear part of the brain, consistent with rotational trauma experiments with young adult and mature adult rats. Young adult to old age brain tissue softening and brain atrophy resulted in an increase in diffuse axonal injuries and acute subdural hematoma injury risk indicators, respectively.
Conclusions: The findings presented in this study suggest that age-specific injury thresholds should be developed to enable the development of superior restraint systems for the elderly. The findings also motivate other further studies on age-dependency of head trauma.
Introduction
Traumatic brain injuries (TBIs) account for half of the 1.3 million annual deaths and 50 million traffic-related injuries worldwide (World Health Organization Citation2012). Acute subdural hematoma (ASDH) and diffuse axonal injuries (DAIs) are the 2 most common types of severe TBI (Sawauchi et al. 2007). Children, young adults, and the elderly are at higher risks than mature adults (Bener et al. Citation2010; Bruns and Hauser Citation2003). As the aging population live considerably longer (United Nations, Department of Economic and Social Affairs 2012), understanding and considering the effect of these changes on injury outcome will become crucial to developing effective prevention countermeasures.
Age-dependent injury risks may be related to changes in composition of brain tissue throughout development (Elkin et al. Citation2010; Thibault and Margulies Citation1998), maturity, and senescence (Rouser and Yamamoto Citation1968; Svennerholm et al. Citation1994). Such changes have been shown to correlate with brain tissue stiffness in very young brain tissue (Elkin et al. Citation2010; Thibault and Margulies Citation1998). Human brain tissue lipid contents (from 20 to 100 years old) change as a function of age. The lipid properties change rather uniformly until 30 to 50 years of age; then the change reverses (Rouser and Yamamoto Citation1968; Svennerholm et al. Citation1994). The data suggest that important changes may also occur after entering adulthood. Sack et al. (Citation2009) estimated brain tissue viscoelastic modulus from multifrequency magnetic elastography conducted on 18- to 88-year-oldvolunteers. The results suggest that stiffness peaks can also occur after reaching adulthood (at ages from 30 to 50 years), after which modulus would gradually decrease as a function of age until senescence. In addition to brain tissue property changes, differences in injury risk in the elderly may be affected by brain atrophy (Matsumae et al. Citation1996) or due to selective and differential brain shrinkage (Xu et al. Citation2000).
Axonal damage is produced when brain tissue is subjected to strain (Anderson et al. Citation2003; Bain and Meaney Citation2000; Galbraith et al. Citation1993). ASDH is produced when violent motions of the brain inside the skull tear the vasculature between the brain cortex and the dura mater (Meaney 1991). Therefore, brain tissue strain (or stress) and brain–skull relative motion measurements are suitable indicators of brain injury risk in studies using finite element (FE) models of the head and neck complex.
Cumulative strain damage measurement (CSDM) is a local brain tissue criterion that calculates the cumulative volume of brain tissue exposed to strains above a predefined critical level (Takhounts et al. Citation2003, 2008). Based on reconstructions of real-life human impacts and scaled animal experimental data with a human FE model, DAI risk curves were proposed on the basis of the calculated CSDM (Takhounts et al. Citation2013). Following the same methodology, the relative motion damage measurement (RMDM), which accounts for relative displacements between the surface of the brain cortex and the skull, was correlated to ASDH injuries (Takhounts et al. Citation2003). Yanaoka and Dokko (Citation2013) utilized these and other injury indicators to analyze the effect of age-dependent parameters on potential injury outcome by conducting a parametric study with a human head FE model. This simulation-only study suggested that 2 of the most influential age-related parameters were brain shrinkage and shear stiffness of the brain matter.
Animal experimental data compensate to some degree for the lack of data from experiments with humans in a laboratory setting. To improve the understanding between head kinematics producing the injuries and the injury mechanisms and injury site, experiments are conducted and reconstructed with FE models of the specimens. This methodology has been conducted with different animal species and models, such as monkeys (Antona-Makoshi et al. Citation2013), sheep (Anderson et al. Citation2003), swine (Meaney et al. Citation1993), ferrets (Ueno et al. Citation1995), and rats (Antona-Makoshi et al. Citation2014; Lamy et al. Citation2009; Mao et al. Citation2006). However, none of these studies have considered the effect of age on injury outcome.
The life span of the most used laboratory rat strain is 24 months (Cameron et al. Citation1982), whereas life expectancy for humans ranges from 70 to 85 years in developed countries (United Nations, Department of Economic and Social Affairs 2012). Rats develop rapidly during infancy and become sexually mature at about 6 weeks of age, with reproductive senescence occurring at between 15 and 20 months of age (Sengupta Citation2013). Humans become sexually mature between 11 to 13 years of age and women reach menopause between 45 to 55 years. A rat's body and skull rapidly grow from birth to adulthood (3 months). Thereafter, the head stops growing, while the body, different from humans, continues to grow until death (Sengupta Citation2013). Brain shrinkage due to age, which also occurs in humans (Fox and Schott Citation2004), has been identified and quantified for rodents (Timaru-Kast et al. Citation2012), and rat brain tissue properties have been shown to be region dependent and to become stiffer from birth to young adulthood (Elkin et al. Citation2010; Elkin and Morrison Citation2013).
Head rotational experiments with rats have shown a threshold of 1 Mrad/s2 above which DAI is likely to occur (Davidsson et al. Citation2009; Davidsson and Risling 2011). A succeeding study revealed a higher injury threshold but not significant (P =.07), for mature adult rats than for young adult rats (of approximate equivalent human age of 40 and 20 years, respectively; Davidsson et al. 2015). In the young adult rats, DAI were mainly found in the corpus callosum, in regions just ventral of the corpus callosum and along its borders, and in the brainstem; in mature adult rats, DAI were also found in regions further toward the ventral side in the center and rear of the brain: the internal capsule, in the regions surrounding the anterior commissure, and in the preoptic nerve regions. The study found that both the risk of DAI and DAI distribution within the brain may be age dependent (Davidsson et al. 2015). For the brainstem, no clear difference in the number of injured axons between young adult and mature adult groups could be observed. Subdural hematoma was observed on the superior cortex in some animals when they were exposed to rotational accelerations above 1.0 Mrad/s2. summarizes these age-dependent changes in rats.
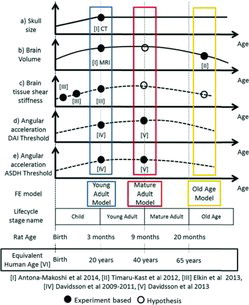
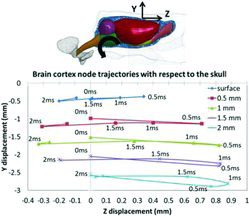
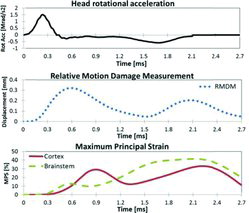
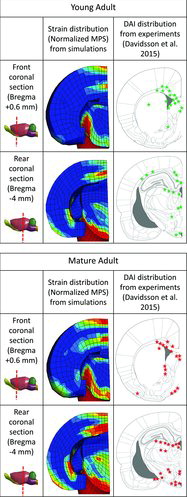
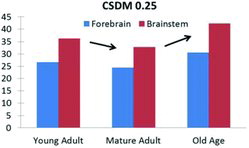
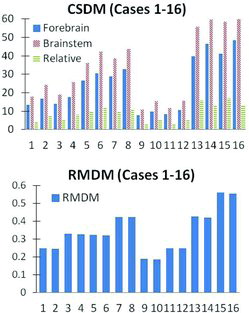
The aim of this study was to investigate the possible effects of age-dependent intracranial changes on the potential outcome of DAI and ASDH as a result of rotational trauma by conducting age-dependent parametric studies with a validated rat head/brain FE model.
Materials and Methods
In this study, an anatomically detailed head/brain FE model of a young adult rat developed from medical images was updated and assigned regional material properties (Elkin et al. Citation2010; Elkin and Morrison Citation2013). The local motion of the model's brain was validated against a series of experiments with rats designed to study relative motion between the brain and the skull (summarized in Appendix A [see online supplement] and described in detail in Antona-Makoshi et al. Citation2014).
The validated FE model of the young adult was utilized to simulate rotational trauma experiments with rats of an approximate equivalent human age of 20 years (Davidsson et al. Citation2009; Davidsson and Risling 2011). Thereafter, brain tissue properties corresponding to fully mature animals were assigned to the model referred to as mature adult, and experiments conducted with rats of an approximate equivalent human age of 40 years were simulated (Davidsson et al. 2015). The model's capacity to predict high-strain concentrations in brain regions commonly exhibiting axonal injuries following rotational trauma was assessed by comparing peak strain distribution patterns from the simulations with schemes of the axonal injuries detected in the experiments (Davidsson et al. Citation2009, 2015; Davidsson and Risling 2011). In addition, the model's capacity to capture a slightly higher injury threshold for mature adult specimens in comparison to that obtained with young adult specimens was assessed by comparing CSDM values.
Finally, a simulation-only parametric study was conducted to study the effect of aging past mature adult. Brain volume reduction and brain tissue softening hypothesized to be representative of old age rats of the equivalent human age of 80 years were introduced. This parametric study was conducted to identify age-dependent parameters that may influence the risk of brain tissue injuries that may need to be considered in future FE models of the head. The results may also be beneficial for guiding future experiments.
All simulations in this study were conducted in LS-DYNA FE code (mpp971s R7.0.0, LSTC Inc., Livermore, CA) and pre- and postprocessing were carried out in LS-PREPOST v4.1 (LSTC Inc.). All rotational trauma experimental data utilized in this study were previously published elsewhere (Antona-Makoshi et al. Citation2014; Davidsson et al. Citation2009, 2015; Davidsson and Risling 2011). Qualitative properties assigned to the young adult, mature adult and the old agebrain FE models developed in this study are highlighted in and described in detail in the following sections.
Development of the Young Adult Rat Brain FE Model
An FE model of a young adult rat head and brain developed from medical images (Antona-Makoshi et al. Citation2014) was enhanced through remeshing in this study. The enhanced model contains bony parts (skull and C2 vertebrae), modeled with shell rigid elements and brain parts (bulbus olfactorius, cortex, corpus callosum, hippocampus, thalamus, brainstem, cerebellum white matter, cerebellum grey matter) and the upper part of the spinal cord, modeled with deformable hexahedral solid elements. Brain regions were assigned region-specific Prony series linear viscoelastic material properties (Elkin et al. Citation2010; Elkin and Morrison Citation2013) summarized in . A pia mater membrane model surrounding the surface of the brain and the brainstem was built and isotropic elastic material properties with a Young's modulus of 30 MPa were assigned (Kimpara et al. Citation2006). A simplified model of the pia–arachnoid–complex and the cerebrospinal fluid (CSF) was built; the dura mater and the arachnoid mater were not modeled, and the space between the pia mater (brain surface) and the inner rigid skull bone was filled with tetrahedral solid elements simulating the CSF. Because these tetrahedral elements share nodes with the brain surface and the skull, contact was not defined, resulting in the brain–skull relative surface motion being controlled by the behavior of the CSF model. The CSF was defined as an isotropic, incompressible hyperelastic material model with a bulk modulus of 2.1 GPa (density of 1,000 kg/m3 and Poisson ratio of 0.499999; McElhaney et al. Citation1976). The ventricular space was filled with CSF elements with the same properties. The final model (shown in Figure C1, see online supplement) contains a total of 131,746 solid elements and 58,780 shell elements.
Table 1. Simulation age-dependent parametric study setup and results (in cases 4, 8, 12, and 16 the age-dependent changes are representative of an old age rat)
Validation of the Young Adult Brain FE Models
The simulated brain cortex motion with respect to the skull was validated against data from experiments with rats designed to study relative motion between the brain and the skull (summarized in Appendix A and described in detail in Antona-Makoshi et al. Citation2014). In these experiments, a pin was inserted into the brain and rigidly attached to the skull prior to application of head rotational trauma. The pin was removed from the brain immediately at the end of the experiment and the brain was then perfusion fixated, extracted, and sliced at 0.5, 1, 1.5, and 2 mm deep from the surface. The length of the scar in the brain cortex produced by the pin was measured and interpreted as an estimate of the relative motion between the brain and the skull. The average head rotational acceleration curve (acceleration peak of 1.67 Mrad/s2, duration of about 0.40 ms, total change in rotational velocity of 306 rad/s, and deceleration peak of about 0.5 Mrad/s2) obtained from 3 experiments was utilized to determine the acceleration to the skull of the FE model. A 2.5-ms duration rotational trauma was simulated under these conditions. To validate the local motion of the brain relative to the skull in the FE model, the trajectories followed by nodes at different depths from the brain cortex in the simulations were extracted and compared to the maximum length of the scars measured at corresponding brain cortex depth levels in the experiments.
Assigning Brain PropertiesRepresentative of Mature Adult and Old Age Rats
For the development of the mature adult brain model and to support the parameter study on aging toward reaching old age, data on brain tissue property changes and brain shrinkage as a function of age was reviewed. Brain tissue viscoelastic modulus were estimated from multifrequency magnetic elastography conducted on 18- to 88-year-old male volunteers (Sack et al. Citation2009). The data indicate that the brain stiffness for a 40-year-old human is approximately 30% higher than for a 20-year-old human and about twice as stiff as for an 80-year-old human. Brain tissue data for infant (0.3 months), juvenile (0.6 months), and young adult (2.5 months) rats show gradual stiffening as function of age; mean modulus was measured to be 450, 775, and 1,050 Pa, respectively (Elkin et al. Citation2010; Elkin and Morrison Citation2013). Due to a lack of consistent tissue data from mature adult and old age rats, brain tissue shear modulus values used in the young adult brain FE model (Elkin et al. Citation2010; Elkin and Morrison, Citation2013) were scaled up by 30% for the mature adult brain FE model and scaled down by 50% for the old age brain FE model according to the estimations by Sack et al. (Citation2009). The brain tissue properties used in the young adult, mature adult, and old age FE models are listed in Table D1 (see online supplement).
Timaru-Kast et al. (Citation2012) found an 8% brain volume reduction when brain atrophy was measured through imaging in young (2 months) and old age mice (21 months). Based on these results, the brain volume (including all soft tissue regions and the ventricles) of the old age brain FE model was established by scaling the young adult brain model geometry with a factor of 0.97 in the x, y, and z directions with respect to the brain center of gravity. The skull remained unchanged and the increased space created between the brain surface and the skull was filled with a mesh assigned with CSF material properties.
Comparing Strain Distribution and CSDM Values from the Simulations with Experimental Data
An injurious level average rotational acceleration pulse with a peak of 1.5 Mrad/s2 and duration of 0.40 ms was defined from the experiments that produced DAI (Davidsson et al. Citation2009) and prescribed to the skull of the young adult and the mature adult models. Maximal principal strain (MPS) distribution images and CSDM values were extracted from the simulations and compared with schemes of the DAI distribution and the average DAI grade developed in the experiments (Davidsson et al. 2015).
Parametric Study of Aging and Reaching Old Age
A 4-parameter, 2-level full-factorial design parametric study, including a total of 16 simulations, was conducted to investigate the potential contribution of age-dependent parameters in the FE model on local brain injury risk predictors at different loading conditions. Two rotational acceleration loading parameters, magnitude (high and low) and duration (short and long), and 2 brain settings, brain volume reduction (normal and reduced) and brain tissue softening (stiff and soft), were implemented in the study. The loading parameters are explained below; brain settings are presented in the previous sections, and the complete parametric study design is presented in Table 1.
In the parametric study, the injurious level rotational acceleration pulse (with a peak of 1.5 Mrad/s2 and a duration of 0.40 ms) that was previously utilized to compare the young adult and mature adult models to the trauma experiments (Davidsson et al. Citation2009, 2015) was denoted high-short. A noninjurious level curve (with a peak of 0.85 Mrad/s2 and a duration of 0.40 ms), denoted low-short, was defined from the experiments that did not produce DAI (Davidsson et al. Citation2009). Finally, the high-short and the low-short curves were scaled in time so that the duration of the acceleration phase increased from 0.40 to 0.49 ms, resulting in 2 additional loading conditions, hereafter denoted as high-long and low-long. The scaling in time was adjusted so that the high-short and the low-long curves produced the same maximum head extension angle of 20°. The 4 curves utilized in the parametric study are shown in Figure E1 (see online supplement).
Simulation Data Analysis
MPS distribution, CSDM (25%), and average RMDM were calculated as indicators of the risk of DAI and ASDH, respectively. MPS distribution was calculated by extracting peak MPS from the simulations and normalizing these values by the mean MPS obtained from all of the elements. Fringe plot images of the strain distribution at 2.4 ms were produced for 2 sections, front (Bregma +0.6 mm) and rear (Bregma−4.0 mm), and compared with the injury distribution schemes from the experiments (Davidsson et al. Citation2015). CSDM was calculated by tracking the elements that experienced MPS above a predefined critical level of 25% and then the cumulative volume fraction of these elements were calculated with respect to the volume fraction of the whole brain. The average RMDM was obtained by calculating the average relative displacement between 8 nodal pairs on the brain cortex surface (node A) and the inner skull (node B). The RMDM for each node was calculated according to Equation 1 (coordinate system use is displayed in Figure 2):
(1)
Results
Young Adult Rat Brain FE Model Validation
Simulated brain cortex node (on the surface, and 0.5, 1, 1.5, and 2 mm deep from the surface) trajectories with respect to the skull in the sagittal plane of the head are shown in Figure 2. During extension of the head, the brain lagged behind the skull, reaching a maximum relative displacement of up to 0.8 mm at approximately 0.5 ms. Thereafter, the brain caught up with the skull, reaching the approximate initial position at about 1.5 ms. At this stage, the skull was being subjected to flexion acceleration, which intensifies the brain motion rearwards with respect to the skull. Around 2 ms after the initiation of trauma, the brain cortex reached maximum rearward displacement with respect to the skull. The total maximum displacements of 1, 1.2, 1, and 0.9 mm (at 0.5, 1, 1.5, and 2 mm depth from the surface) obtained in the simulations agreed well with the experimental results; the total maximum displacements in the experiments were 1.2, 1.2, 1, and 0.6 mm (see Table A1, online supplement).
Brain Injury Indicators in Simulated Rotational Experiments with Young Adult Rats
Injury indicators (average RMDM and MPS from representative elements of the brain cortex and the brainstem) resulting from the simulated rotational trauma experiments with young adult rats and head rotational acceleration applied to the model are plotted against time in Figure 3. Peak RMDM was reached at 0.6 ms, shortly after the acceleration peak was reached. Peak MPS values were reached at about 2.4 ms, after the deceleration peak occurred.
Comparing Strain Distribution and CSDM Values from the Simulations with Experimental Data
Figure 4 shows MPS distribution fringe plot images of a frontand a rearsection of the brain obtained from the rotational trauma experiments simulated with young adult and mature adult tissue properties. The images were extracted at 2.4 ms, the approximate time at which peak strains occurred. Corresponding schemes of the DAI distribution (not severe) found in the animal experiments for the young adult and the mature adult (Davidsson et al. 2015) groups are also presented in Figure 4 for comparison. The simulation results showed high strain concentration in the lower part of the brainstem and in the border between the corpus callosum and cortex, which is consistent with the regions in which DAI was located in the experiments with young adult rats. For the mature adult model, high strains were concentrated in similar locations; however, slightly wider strain spread than was seen in the simulations with the young adult model was also found in regions located further ventral and rearwards.
The simulations with the young adult brain properties resulted in CSDM (25%) values in the forebrain and the brainstem of 27 and 36%, respectively. For the mature adult brain properties, lower CSDM values (24% and 33%) were obtained for the forebrain and the brainstem, respectively (Figure 5). These results show model sensitivity to material property changes and that the model correctly predicts the slightly decreased risk of DAI reported for mature adult rats compared to young adult rats (Davidsson et al. 2015).
Parametric Study
Table 1 lists the simulated case number (column 1), input rotational acceleration peak magnitude and duration (columns 2 and 3), and age-dependent brain volume and tissue properties settings (columns 4 and 5). The table also includes the brain tissue response (CSDM and RMDM in columns 6 and 7) from the simulations.
CSDM valuesfrom the 16 simulated cases are plotted in Figure 6 for the forebrain (solid bars), the brainstem (dashed bars), and the relative difference between the 2 regions (striped bars). CSDM values were sensitive to both magnitude (cases 1 to 4, in comparison to cases 13 to 16) and duration (cases 1 to 4, in comparison to cases 9 to 12) of the applied loading conditions, where they werelower for the subinjurious (cases 1 to 4 and 9 to 12) than for the injurious (cases 5 to 8 and 13 to 16) loading levels. For constant loading conditions, CSDM increased when only material properties were softened (case 1 in comparison to case 2) and remained constant or increased slightly when only brain volume was reduced (case 1 in comparison to case 3). Material softening (cases 5 and 6) increased the relative difference in CSDM between the forebrain and the brainstem. Volume reduction (cases 5 and 7) had almost no effect on this difference even though an increase in CSDM was recorded.
Average RMDM values at the cortex surface are presented in Figure 6. With constant brain volume and material properties, RMDM decreased when duration was shortened (case 1 in comparison to case 9) and increased when peak acceleration was increased (case 1 in comparison to case 13). Almost no difference could be observed when comparing simulations in which only material properties were modified (case 5 in comparison to case 6). Brain volume reduction produced an increase in RMDM values (case 5 in comparison to case 7).
Discussion
The motion of the brain cortex of the rat head FE model developed in this study has been compared to a series of experiments with rats designed to study relative motion between the cortex and the skull (Antona-Makoshi et al. Citation2014). In the experiments, a thin pin was inserted through the skull and 2 mm into the brain cortex. This pin produced scars in the brain tissue during trauma, which thereafter wereanalyzed at different depths. Experiments with 3 animals were carried out; on average the superficial cortex displaced 1.2 mm relative the skull and less at more central locations. The simplified pia–arachnoid–complex–CSF model implemented in the rat FE model was developed to allow certain degree of relative motion of the brain surface with respect to the skull. This, in combination with the implemented brain tissue material properties (Elkin et al. Citation2010; Elkin and Morrison Citation2013), allowed certain brain–skull relative motion and internal brain deformations with magnitudes reasonably close to those measured in the experiments (Antona-Makoshi et al. Citation2014; Figure 2).
A large number of local brain injury risk indicators have been proposed, compared, and ranked in the literature (Kleiven Citation2007; Takhounts et al. Citation2003, 2008). Our study aimed neither to evaluate criteria nor to establish quantified values applicable for human studies. Instead, we selected reasonably well-established indicators for the targeted injuries (DAI and ASDH) and evaluated how changes in age-dependent factors implemented in the brain model affected the injury risk indicators. Strain-based injury indicators (MPS and CSDM), which have been largely shown to correlate with DAI injury severity and locations through reconstructions of both animal experimental data scaled to humans (Takhounts et al. Citation2003, 2008) and human accidents (Kleiven Citation2007; Takhounts et al. Citation2013), were selected as reasonable indicators of DAI risk. RMDM, which accounts for relative motion between the brain cortex surface and the skull, may be a suitable ASDH risk indicator for modeling techniques that allow certain relative brain–skull motion through either tiebreak–type contacts (Takhounts et al. Citation2003) or sliding-type contacts (Kleiven 2007; Zhang et al. 2001) but not for models with tied-type contacts (Takhounts et al. Citation2008). Because the brain–skull interaction we implemented in the rat FE model allowed for some relative motion between the brain and the skull (Figure 2), RMDM was selected as an ASDH injury predictor suitable for the purpose of our study.
Simultaneous analysis of the input skull acceleration curves and the resulting MPS and RMDM (Figure 3) provided an opportunity to analyze the mechanics of the trauma progression. At the initial loading stage, when the head was subjected to extension, the brain lagged behind the skull due to inertia and viscoelasticity. This produced a relative motion between the upper surface of the brain cortex and the inner skull, triggering a raise in RMDM until reaching a peak at 0.6 ms, shortly after the rotational acceleration peak was reached. Thereafter, the skull was subjected to deceleration, while the brain cortex and the brainstem were still traveling rearwards. When the internal regions of the brain eventually caught up with the utmost brain regions and the skull, the generated momentum for the internal regions produced large internal strains (MPS peaks at 2.4 ms) in the forebrain and the brainstem as the skull was subjected to flexion acceleration.
Age-dependent tissue properties had an effect on CSDM. Figure 5 shows CSDM results from simulations with the same rotational loading conditions (high-short) and the same (normal) brain geometry for young adult (case 5 in the parametric study), mature adult, and old age tissue properties (case 6 in the parametric study). Mature adult material properties (scaled up by 30% from young adult) produced smaller CSDM values (Figure 5), with strains slightly redistributed toward the rear regions of the brain and the brainstem (Figure 4), which is in line with reported experimental findings (Davidsson et al. 2015). Consistently, old age brain tissue properties (scaled down 50% stiffer from young adult) produced higher CSDM values that would, assuming that the tissue critical strain level is not age dependent, suggest a potential increased risk of DAI for the old age.
Brain volume reduction in old age had an effect on RMDM. Brain volume reduction created an increased space between the brain and the skull, which was filled with additional CSF soft elements during simulations. This resulted in the brain being given increased freedom to move within the rotating skull cavity, which produced elevated peak RMDMs. Due to the lack of rotational trauma data for old age rats, it was not possible to confirm this finding with animal experiments. However, this finding is consistent with clinical data on humans (Chen and Levy Citation2000; Kameyama et al. Citation2008) and with the results of a previous age-dependent parametric study conducted with a human FE model (Yanaoka and Dokko Citation2013). Implemented brain volume reduction (atrophy) would suggest a potential increase in ASDH in old age.
The findings in this study, if translated to humans, could have implications in traffic injury prevention; brain kinematics analysis suggest that countermeasures focused on reducing peak acceleration would potentially generate a positive effect on ASDH injury reduction at an early stage of the trauma progression, and countermeasures focused on reducing the differences between acceleration and deceleration peaks would potentially be beneficial for DAI injury reduction occurring at later stages of the trauma. In addition, the observed contribution of brain tissue properties on DAI indicators, of brain volume changes on ASDH indicators, and the lack of interaction between both types of indicators, allow speculating that specific advanced restraint systems designed, for example, to reduce of ASDH risk in the elderly would not arouse any contrary effects in DAI risk in the elderly or any both types of injuries inadults.
The most significant limitations of this study apply to most state-of-the-art animal and human brain FE models. In general, lack of mechanical properties and improved models of brain tissue for simulation of events involving high-speed impacts; producing large tissue strains in the brain tissue. In particular, this study lacks tissue properties to define mature adult and old age rat specimens, as well as rotational trauma experimental with old age specimens.
The influence of age-related parameters on intracranial response during rotational head trauma has been examined with a validated rat head/brain FE model. Sequential analysis of the trauma progression indicates that the raise of ASDH injury risk occurs at an early stage of the trauma, and the increase in DAI risk occurs at a later stage. Tissue stiffening from young adult to mature adult rats produced an increase in strain-based thresholds accompanied by a wider spread of strain distribution toward the rear part of the brain, consistent with rotational trauma experiments with young adult and mature adult rats. Young adult to old age brain tissue softening and brain atrophy resulted in an increase in DAI and ASDH injury risk indicators, respectively. These findings, if applicable to humans, suggest that injury type and age-specific reduction strategies in the development of superior restraint systems may be possible, motivating further studies on age-dependency of head trauma.
Funding
Funding for this research was provided by the Japan Society for the Promotion of Science (JSPS) Summer Program, the Japan Automobile Research Institute (JARI), and Chalmers University Areas of Advance within Transport, Sweden.
Appendix
Download PDF (388.5 KB)References
- Anderson RW, Brown CJ, Blumbergs PC, McLean AJ, Jones NR. Impact mechanics and axonal injury in a sheep model. J Neurotrauma.2003;20:961–974.
- Antona-Makoshi J, Davidsson J, Ejima S, Ono K, Brolin K, Anata K. Correlation of global head and brain tissue injury criteria to experimental concussion derived from monkey head trauma experiments. Paper presented at: International Research Council of Biomechanics (IRCOBI) Conference; 2013; Gothenburg, Sweden.
- Antona-Makoshi J, Davidsson J, Risling M, Ejima S, Ono K. Validation of local brain kinematics of a novel rat brain finite element model under rotational acceleration. Int J Automot Eng. 2014;5:31–37.
- Bain AC, Meaney DF. Tissue-level thresholds for axonal damage in an experimental model of central nervous system white matter injury. J Biomech Eng.2000;122:615–622.
- Bener A, Omar AOK, Ahmad AE, Al-Mulla FH, Rahman YSA. The pattern of traumatic brain injuries: a country undergoing rapid development. Brain Inj. 2010;24(2):74–80.
- Bruns J, Hauser WA. The epidemiology of traumatic brain injury: a review. Epilepsia. 2003;44(10):2–10.
- Cameron TP, Lattuada CP, Kornreich MR, Tarone RE. Longevity and reproductive comparisons for male ACI and Sprague-Dawley rat aging colonies. Lab Anim Sci. 1982;32:495–499.
- Chen JC, Levy ML. Causes, epidemiology, and risk factors of chronic subdural hematoma. Neurosurg Clin N Am. 2000;11:399–406.
- Davidsson J, Angeria M, Risling M. Injury threshold for sagittal plane rotational induced diffuse axonal injuries. Paper presented at: International Research Council of Biomechanics (IRCOBI) Conference; 2009; York, England.
- Davidsson J, Angeria M, Risling M. Effect of age on amount and distribution of diffuse axonal injury after rotational trauma. Frontiers of Neurology; 2015 (submitted).
- Davidsson J, Risling M. A new model to produce sagittal plane rotational induced diffuse axonal injuries. Frontiers of Neurotrauma. 2011;2(41):1–11.
- Elkin BS, Ilankovan A, Morrison B. Age-dependent regional mechanical properties of the rat hippocampus and cortex. J Biomech Eng. 2010;132:011010.
- Elkin BS, Morrison B. Viscoelastic properties of the P17 and adult rat brain from indentation in the coronal plane. J Biomech Eng. 2013;135:114507.
- Fox NC, Schott JM. Imaging cerebral atrophy: normal ageing to Alzheimer's disease. The Lancet.2004;363:392–394.
- Galbraith JA, Thibault LE, Matteson DR. Mechanical and electrical responses of the squid giant axon to simple elongation. J Biomech Eng. 1993;115:13–22.
- Kameyama M, Karibe H, Onuma T, Tominaga T. Epidemiological study of head injury in miyagineurotrauma data bank: age, cause of injury, pathophysiology and outcome [in Japanese]. Neurotraumatology. 2008;31:46–56.
- Kimpara H, Nakahira Y, Iwamoto M, et al. Investigation of anteroposterior head–neck responses during severe frontal impact using a brain–spinal cord complex FE model. Stapp Car Crash J. 2006;50:27–51.
- Kleiven S. Predictors for traumatic brain injuries evaluated through accident reconstructions. Stapp Car Crash J. 2007;51:81–114.
- Lamy M, Baumgarter D, Willinger R, et al. Finite element analysis of traumatic brain injuries mechanisms in the rat. Paper presented at: International Research Council of Biomechanics (IRCOBI) Conference; 2009; York, England.
- Mao H, Zhang L, Yang KH, King AI. Application of a finite element model of the brain to study traumatic brain injury mechanisms in the rat. Stapp Car Crash J. 2006;50:583–600.
- Matsumae M, Kikinis R, Morocz IA, et al. Age-related changes in intracranial compartment volumes in normal adults assessed by magnetic resonance imaging. J Neurosurg. 1996;84:982–991.
- McElhaney JH, Roberts VL, Hilyard JF. Handbook of Human Tolerance.Tokyo, Japan: Japan Automobile Research Institute Inc; 1976.
- Meaney DF. Biomechanics of acute subdural hematoma in the subhuman primate and man [PhD dissertation]. University of Pennsylvania, Philadelphia, PA; 1991.
- Meaney DF, Smith D, Ross DT, Gennarelli TA. Diffuse axonal injury in the miniature pig: biomechanical development and injury threshold. ASME Crashworthiness and Occupant Protection in Transportation Systems. 1993;25:169–175.
- Rouser G, Yamamoto A. Curvilinear regression course of human brain lipid composition changes with age. Lipids. 1968;3:284–287.
- Sack I, Beierbach B, Wuerfel J, et al. The impact of aging and gender on brain viscoelasticity. Neuroimage. 2009;46:652–657.
- Sawauchi S, Murakami S, Ogawa T, Abe T. Mechanism of injury in acute subdural hematoma and diffuse brain injury: analysis of 587 cases in the Japan Neurotrauma Data Bank [in Japanese]. No ShinkeiGeka. 2007;35:665–671.
- Sengupta P. The laboratory rat: relating its age with human's. Int J Prev Med. 2013;4:624–630.
- Svennerholm L, Boström K, Jungbjer B, Olsson L. Membrane lipids of adult human brain: lipid composition of frontal and temporal lobe in subjects of age 20 to 100 years. J Neurochem. 1994;63(5):1802–1811.
- Takhounts EG, Craig MJ, Moorhouse K, McFadden J, Hasija V. Development of brain injury criteria (BrIC). Stapp Car Crash J. 2013;57:243–266.
- Takhounts EG, Eppinger RH, Campbell JQ, Tannous RE, Power ED, Shook LS. On the development of the SIMonfinite element head model. Stapp Car Crash J. 2003;47:107–133.
- Takhounts EG, Ridella SA, Hasija V, et al. Investigation of traumatic brain injuries using the next generation of simulated injury monitor (SIMon) finite element head model. Stapp Car Crash J. 2008;52:1–31.
- Thibault KL, Margulies SS. Age-dependent material properties of the porcine cerebrum: effect on pediatric inertial head injury criteria. J Biomech. 1998;31:1119–1126.
- Timaru-Kast R, Luh C, Gotthardt P, et al. Influence of age on brain edema formation, secondary brain damage and inflammatory response after brain trauma in mice. PLoS ONE. 2012;7.
- Ueno K, Melvin JW, Li L, Lighthall JW. Development of tissue level brain injury criteria by finite element analysis. J Neurotrauma. 1995;12:695–706.
- United Nations, Department of Economic and Social Affairs. World Population Prospects: The 2012 Revision. 2012. Available at: http://esa.un.org/wpp. Accessed February 19, 2015.
- World Health Organization. Global Plan for the Decade of Action for Road Safety 2011–2020. 2012. Available at: http://www.who.int/roadsafety/decade_of_action/plan/en. Accessed February 19, 2015.
- Xu J, Kobayashi S, Yamaguchi S, Iijima KI, Okada K, Yamashita K. Gender effects on age-related changes in brain structure. Am J Neuroradiol.2000;21:112–118.
- Yanaoka T, Dokko Y. A parametric study of age-related factors affecting intracranial responses under impact loading using a human head/brain FE model.Paper presented at: International Research Council of Biomechanics (IRCOBI) Conference;2013; Gothenburg, Sweden.
- Zhang L, Yang KH, Dwarampudi R, et al. Recent advances in brain injury research: a new human head model development and validation. Stapp Car Crash J. 2001;45:369–394.