Abstract
Objective
In 2020, 17% of all crash fatalities were individuals aged 65 years or older. Crash data also revealed that for older occupants, thoracic related injuries are among the leading causes of fatality. Historically, the majority of near-side impact postmortem human subjects (PMHS) studies used a generic load wall to capture external loads that were applied to PMHS. While these data were helpful in documenting biofidelity, they did not represent a realistic response an occupant would undergo in a near-side crash. The objective of this research was to test small, elderly female PMHS in a repeatable, realistic near-side impact crash scenario to investigate current injury criteria as they relate to this vulnerable population.
Method
Ten small, elderly PMHS were subjected to a realistic near-side impact loading condition. The PMHS were targeted to be elderly females age 60+, approximately 5th percentile in height and weight, with osteopenic areal bone mineral density. Each subject was seated on a mass-production seat, equipped with a side airbag and standard three-point restraint with a pretensioner. Other boundary conditions included an intruding driver’s side door. PMHS instrumentation included strain gages on ribs 3-10 bilaterally to identify fracture timing. Two chestbands were used to measure chest deflection, one at the level of the axilla and one at the level of the xiphoid process.
Results
Injuries observed included rib fractures, particularly on the struck side, and in multiple cases a flail chest was observed. Eight of ten subjects resulted in AIS3+ thoracic injuries, despite previously tested ATDs predicting less than a 10% chance of AIS3+ injury. Subjects crossed the threshold for AIS3 injury in the range of only 1% − 9% chest compression. Additionally, mechanisms of injury varied, as some injuries were incurred by door interactions while others came during airbag interactions.
Conclusions
This research points to two areas of concern that likely require further analysis: (1) the appropriateness of potentially oversimplified PMHS testing to establish injury thresholds and define injury criteria for complicated crash scenarios; (2) the importance of identifying the precise timing of injuries to better understand the effect of current passive restraint systems.
Introduction
In 2020, 17% of all crash fatalities were individuals aged 65 years or older. While there was a 10% decrease in the number of total fatalities from the previous year’s data of older occupants, the trend of a higher fatality rate for older occupants over those under the age of 65 remained. Over the past decade the number of people over the age of 65 in the United States has increased by 35%, while the total population has only increased by 6% (NHTSA, Citation2022). The expansion of this vulnerable group combined with the higher rate of fatality means that focusing on this demographic’s safety could significantly decrease the number of future fatalities.
Database reviews of the National Automotive Sampling System Crashworthiness Data System (NASS-CDS) revealed that older occupants in nearside crashes have a higher rate of injury, with the thorax being particularly vulnerable. Sunnevång et al. (Citation2010) found that for near-side crashes between 1994-2008, the thorax was one of the most vulnerable regions for severe injuries for all age groups. Additionally, older occupants had a 4-times higher rate of thoracic injury than younger occupants at near-side impact speeds between 40 and 80 km/h. A recent study was completed using data from the Korean In-Depth Accident Study database to review injuries to over 3,000 patients that presented to Level I Trauma Centers between 2011 and 2017 (Kong et al. Citation2018). The authors focused on non-catastrophic crashes based on collision deformation in which the occupants had been injured while seated in the front row. The study found that the rate of severe injury among occupants older than 55 was almost 1.6 times higher than that of occupants under the age of 55. The authors also found that the thorax was the most often severely injured body region of the older occupant group compared to the head, neck, and the extremities being the most often injured regions of the younger occupant group (Kong et al. Citation2018). When looking at restraint use, the National Highway Traffic Safety Administration’s (NHTSA) 2020 data reveal that 68% of older occupant fatalities were belted, while only 45% of occupant fatalities under 65 years were belted. The 2020 data also revealed that when compared to drivers under the age of 65, older drivers are more frequently killed in left-side impacts (16% vs 10%) and right-side impacts (9% vs 7%) (NHTSA, Citation2022). These past findings necessitate further investigation into near-side crashes, with a special focus on older occupants.
The majority of past near-side impact experimental studies used a generic load wall to capture external loads applied to postmortem human subjects (PMHS) (Morgan et al. Citation1986, Maltese et al. Citation2002, Kuppa et al. Citation2003, Yoganandan et al. Citation2012). Sled testing using such load walls often included both rigid and padded conditions. Cavanaugh et al. (Citation1993) additionally used different pelvic offsets and wall geometries while Yoganandan et al. (Citation2012) created a modular load wall which allowed for oblique and pure lateral side impact tests. While these data helped document both kinematic response and thoracic injury due to a simplified, generic side impact loading scenario, they do not represent a realistic environment an occupant would undergo in a near-side crash due to the lack of PMHS interaction with a standard seat, seatbelt restraint, airbag, or even an intruding car door.
Historically, thoracic biofidelity response corridors and injury risk curves for small females in near-side impacts have been derived for 5th percentile female anthropomorphic test devices (ATD) by scaling mid-size male PMHS data sets (Mertz et al. Citation2003). However, these scaled data may not be representative of small females. For example, Kimpara et al. (Citation2003) conducted a retrospective analysis of 83 frontal and lateral blunt cadaveric impacts and documented differences in chest stiffness between male and female PMHS. Chest stiffness and other variables are often not accounted for, or assumed to be the same between different demographics; thus, scaled mid-size male data may not be representative of the 5th percentile female. To date, very few impactor or sled test series using 5th percentile, elderly females in a near-side configuration have been conducted (Yoganandan and Pintar Citation2005, Wood et al. Citation2014, Baudrit et al. Citation2014). In addition, these test series also typically included 50th percentile males and either studied kinematic response only or created biofidelity response corridors and injury risk curves using scaling techniques between different subsets of occupants.
Given the increased occurrence of thoracic injuries in small, older females in near-side car crashes and the lack of realistic boundary condition testing completed with 5th percentile PMHS to date, more elderly female-specific data are required to determine if current scaling-based injury risk curves are appropriate to help safety engineers reduce these critical thoracic injuries. The objective of this research was to test small elderly female PMHS in a repeatable, realistic near-side impact crash scenario to evaluate currently accepted injury criteria as they relate to this vulnerable population.
Methods
Subject selection
Ten PMHS, obtained through The Ohio State University's Body Donation Program in accordance with all National Highway Traffic Safety Administration and University guidelines on the ethical use of anatomical donors, were utilized in this study. Included female subjects were approximately 5th percentile for height (136–167 cm) and weight (42–56 kg). Elderly PMHS were targeted, defined here as being at least 60 years old and osteopenic. Subjects were identified as osteopenic based on the clinical standard of lumbar t-score for areal bone mineral density (aBMD) from dual-energy x-ray absorptiometry (DXA) between –1 and –2.5 (Kanis et al. Citation1994), calculated as a comparison to a young reference population. These subjects were purposefully identified to represent a more vulnerable group than small size and/or advanced age alone. However, an attempt was also made to ensure the elderly PMHS were representative by targeting those with lumbar z-scores within 1 standard deviation of the mean of an age-matched reference population. Subject information and relevant anthropometric measurements are summarized in . PMHS were excluded from the study if any pre-existing injuries or pathological changes that would influence outcomes were identified from whole-body computed tomography (CT) scans conducted during the screening process. It is important to note that two separate phases of testing were conducted, and each phase contained five PMHS, tested once each. Thus, in , PMHS 1-5 were used in Phase I testing and PMHS 6-10 were used in Phase II testing.
Table 1. Subject information.
Test setup
All ten PMHS were subjected to a realistic near-side impact loading condition, including both the base motion of the impacted vehicle and an intruding door. Data from a series of NHTSA and Insurance Institute for Highway Safety (IIHS) side impact vehicle impact tests along with data from laboratory component tests were used in a finite element simulation to determine sled test boundary conditions in which an elderly female occupant might receive serious thoracic injuries. The main basis for the simulation and the sled test series input conditions was the impact of a 2016 compact vehicle by the Federal Motor Vehicle Safety Standard (FMVSS) No. 214 Moving Deformable Barrier (MDB) test condition. The base acceleration of the Hydraulic-controlled, Gas-Energized (HyGE) sled was tuned to match the acceleration profile of the impacted vehicle as determined by the finite element simulation with a delta-V of 50 kph instead of the experimental 54 kph to slightly decrease the risk of thoracic injury (Sugaya et al. Citation2019). Door intrusion was replicated using the Advanced Side Impact System (ASIS) (DSD, Linz, Australia) on the HyGE sled (Kittanning, PA). The ASIS contains four independently controlled pneumatic rams that can be programmed to enable door intrusion to be included in a sled test with ATDs or PMHS (Kinoshita et al. Citation2011). The pneumatic cylinders were controlled by a closed-loop system that used displacement as feedback. Each cylinder intrusion profile was based on recorded accelerometer data taken from the inner steel structure of the 2016 compact vehicle door impacted by a FMVSS No. 214 MDB. (Supplementary material) reveals the four sections of the door and the approximate body region of the occupant that each section interacts with. Finally, the four door sections were covered with a production door liner from the 2016 compact vehicle.
Figure 1. Pretest photos showing the PMHS in a vehicle seat with standard three-point restraint system, side airbag with a single fold, and the door liner attached to the four ASIS pneumatic actuators.
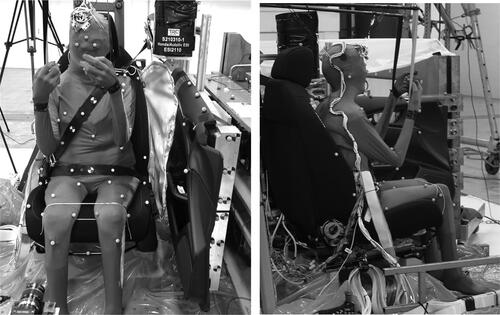
The test setup also included a modern mass-production driver’s seat equipped with a side airbag and a standard three-point restraint with a pretensioner, all of which are standard in the 2016 compact vehicle (). Prior to the test, the side airbag was extended from behind the seat and positioned with a single fold in the bag in order to ensure repeatable inflation across all tests. The seatbelt pretensioner and the airbag were both triggered at approximately 9 msec into the event. Typically, the airbag is fired 1 msec before the pretensioner, but because the airbag was placed external to the seat, it was delayed to match the pretensioner. ATD tests were performed prior to the PMHS tests to ensure the placement and timing of the airbag inflation were similar to results documented in prior NHTSA FMVSS and IIHS side impact tests. Phase I (tests 1-5) included a standard production airbag used in the 2016 compact vehicle. This resulted in a combined thoracic loading scenario due to anterior/posterior (A/P) loading from the pretensioner occurring at the same time as lateral airbag loading. Phase II (tests 6-10) included an experimental c-shaped airbag which was designed to delay the loading of the thoracic region, such that A/P loading from the seatbelt pretensioner was finalized before initiation of lateral loading from the airbag. The differences in the airbags used for Phase I and Phase II testing can be visualized in . Although side curtain airbags are also standard on the 2016 vehicle, they were not included in the sled simulation given that the testing focus was on thoracic response and not on the head or cervical spine. To protect the head from direct impact with the top of the door, a simple foam block was placed on the upper side of the door.
Instrumentation
The sled buck and ASIS were instrumented with accelerometers to quantify the acceleration of the test fixture and door intrusion in the Y-direction (SAEJ211, Citation2014). The three-point restraint system was instrumented with four load cells to measure seatbelt tension. The tension load cells were placed: (1) between the D-ring and retractor, (2) between the D-ring and occupant’s shoulder, (3) on the shoulder belt segment at the buckle, and (4) on the lap belt segment between the occupant’s hip and the belt anchor point. Each side airbag was instrumented with two pressure transducers to quantify airbag pressure at an upper, forward location and at a lower, rearward location as each airbag had two separate pressure chambers.
After breast tissue removal, each subject was instrumented with a combination of 6DX Pro motion blocks (18 K deg/sec, DTS, Seal Beach, CA), strain gages (Model CEA-13-062UW-350/P2, Micro Measurements, Wendell, NC), and chestbands (Humanetics Innovative Solutions, Plymouth, MI) to fully quantify occupant response in side impacts (Pintar et al. Citation1996; Yoganandan et al. Citation2011). The 6DX Pro motion blocks were installed on each subject’s manubrium, sacrum, left iliac wing, and the T1, T4, T12, and S1 vertebrae. Uni-axial strain gages were placed on the lateral aspect of the left clavicle, the body of the sternum, and both anterior and posterior aspects of the thoracic cage. Specifically, gages were placed on the anterior aspect of ribs 2-10 on the left side and ribs 3-10 on the right side and on the posterior aspect of ribs 4-9 on the left side and ribs 4-7 on the right side. Two chestbands were secured to the thorax under 20 lbs. of tension and were used to measure chest deflection at the level of the axilla and the xiphoid process. The anatomical locations of all the instrumentation are shown in (Supplementary material).
Subject positioning
The mass-production seat with the seatback set to an angle of 22° and featuring a seat pan angle of 15°, was placed in a middle-track position. The PMHS were placed onto the seat by positioning the pelvis into the seat bight as posteriorly as the seat back would allow. The thoracic spine was then positioned to be at approximately 0°, creating space between the upper seatback and the shoulders of each PMHS. The lower extremities were allowed to flex naturally across the front edge of the seat, and the feet did not touch the ground in many cases. The arms were extended to 40° with respect to the thorax and the forearms were positioned as if holding a steering wheel. Finally, the head was positioned within 5° of the Frankfort Plane. After positioning each PMHS, the 3D coordinates of anatomical landmarks were digitized using a FARO arm device (Edge FaroArm, Faro Arm Technologies, Lake Mary, FL, USA) to ensure similar positioning across all tests.
Data analysis
Data were collected at a sampling rate of 20,000 Hz and filtered in accordance with SAE J211 guidelines for each data channel using a second order, phaseless, Butterworth filter, except for strain gage and chestband data which were not filtered (SAE J211, Citation2014). Chestband processing was completed using CrashStar 2.7 software. Full lateral thorax deflection was calculated as the change in distance between the two lateral-most points on the chestband determined from the pre-impact contour. Half-thorax lateral deflection was assessed using the chestband analysis methods explained in detail by Pintar et al. (Citation2009). Lateral compression was calculated based on the initial chest breadth from the pre-impact contour at the location of each chestband. A/P deflection was calculated as the change in distance between the chestband gages on the spine and the sternum. Strain data from strain gages installed on the ribs were numerically differentiated to obtain strain rate, which was used to determine fracture timing. An exemplar plot for strain and strain rate is shown in (Supplementary material). A strain rate value greater than 10 strains per second was used as an indication of fracture, as the exemplar plot shows at 51 msec. High-speed cameras (MotionXtra N3, Integrated Design Tools, Tallahassee, FL) were used across all tests to record the event at 1,000 frames per second. The main camera view used to analyze injury mechanisms throughout the test series was mounted on the HyGE sled at the height of the PMHS’ umbilicus for a frontal view of the interaction between the PMHS and the airbag and intruding door.
Results
Test input
The setup of Phase I and Phase II tests were designed to replicate an FMVSS No. 214 near-side impact to a 2016 compact vehicle by an MDB with an impact velocity of 50 kph. Despite differences in airbag design between the two test Phases, the HyGE sled pulse and HyGE sled velocity across all 10 tests were similar (Figure A4, Supplementary material). The ASIS system had to be triggered prior to the HyGE sled, so slight differences in HyGE initiation resulted in differences in the performance of the independent intrusion cylinders. The two most critical intrusion cylinders in this test series were cylinder 1 (red) and cylinder 3 (green) which loaded the upper thorax and pelvis, respectively, as shown in Figures A1 and A5 (Supplementary material). The intrusion into the upper thorax and shoulder area of the PMHS was very similar except for PMHS 1 and PMHS 4 in Phase I testing. These two tests were both within 5 cm of the other 8 PMHS tests that were conducted. The intrusion into the lower thorax and pelvis of the PMHS, driven by cylinder 3, were all within 5 cm throughout both test phases. There was more variation recorded in cylinder 2 (yellow, upper extremities) and cylinder 4 (blue, lower extremities). However, these cylinders had limited interaction with the small-statured PMHS and are not considered critical in affecting thoracic injury or kinematic response. The lower and upper airbag pressures, along with the in-line belt forces at the retractor for all tests are also shown in the Appendix (Figures A6–A8, Supplementary material).
Injury summary
The primary injury findings from this study were numerous rib fractures which are summarized in . The table specifies the total number of rib fractures documented for each test, the specific rib levels that were fractured, and the thoracic abbreviated injury score (AIS) for each subject. Other injuries documented in the study are shown in (Supplementary material). The tibia and fibula fractures were due to the unconstrained bottom edge of the intruding door, which is not considered a realistic boundary condition and thus will not be discussed further. Phase I testing with the standard production airbag resulted in a greater number of overall rib fractures than Phase II testing with the experimental c-shaped airbag (51 versus 38 rib fractures, respectively). Every PMHS in Phase I obtained AIS level 3 thoracic injuries. In 4 of the 5 tests, AIS level 3 was achieved within the initial 30 msec, which was during the period when the subject was interacting with the airbag. During Phase II testing, two subjects resulted in an AIS thoracic score <3, and one subject scored a thoracic AIS level 4 due to a pneumothorax which was coded based on a moderate-sized (∼1 cm) pleural laceration between the left 7th and 8th ribs. The timing of severe thoracic injury for the three PMHS in Phase II that achieved AIS level 3 occurred between 39-46 msec, which is later in the event than the Phase I thoracic injuries and aligns in time with airbag deflation and initial door liner interaction.
Table 2. Documented rib injuries and resulting thoracic AIS scores (AIS 2015 Version 7).
Chest deflection
Plots of exemplar chestband contours for Phase I (Test 2) are shown in . The chestband contours observed in Phase I tests show evidence of combined loading: A/P thoracic deflection from the pretensioner loading and lateral deflection from the airbag loading and door intrusion. While the plots reveal the difference in deflection magnitudes between (a) maximum deflection and (b) the deflection at the time of AIS 3 injury, they also reveal that there is deflection in both A/P and lateral directions at the time of injury. Half-lateral and A/P deflections recorded at the axillary level for both peak deflection and at the time the injuries reached AIS level 3 for Phase I and Phase II subjects are shown in , along with the time of max deflection and the time of AIS 3 occurrence. Across all Phase I tests, the pretensioner fired at approximately 9 msec with peak A/P deflection occurring around 20 msec, while lateral loading from interaction with the airbag began at approximately 15 msec and peaked at approximately 40-45 msec. The chestband contours during the Phase II tests show less evidence of combined loading due to the design of the experimental c-shaped airbag. For Phase II tests, the pretensioner fired at approximately 9 msec with peak A/P deflection occurring around 20 msec, while lateral loading from interaction with the airbag began at approximately 16-18 msec but peaking much later at approximately 50 msec due to the increased time to inflate the thoracic region of the bag. Lateral and half-lateral thoracic compression values for the axillary and xiphoid process levels are reported in .
Table 3. Deflections from axilla-level chestband.
Table 4. Compression at both axillary and xiphoid process levels.
Fracture timing
Rib fracture timing was investigated further in relation to the time histories of the chestband deflections, seat belt loading, and airbag loading to determine the mechanism of injury across all tests. shows an exemplar plot from the Phase I series, revealing that AIS level 3 injury (time of third rib fracture, shown in red) was achieved very early in the event while the pretensioner is near peak A/P loading and the side airbag is just beginning to load the lateral aspect of the thorax. This is in contrast to the exemplar plot from the Phase II series, , in which AIS level 3 injury is achieved later in the event when the airbag pressure is decreasing, and the door begins to contact the PMHS through the airbag. Finally, video stills recorded at the time thoracic AIS reaches a level 3 injury for each test are shown in Figures A9 and A10 (Supplementary material). The time at which the still was taken is shown under each figure. It is important to visualize the relative positions of the PMHS with respect to the intruding door liner and also the amount of pressure left in the airbag. From the two figures, the injuries occurred with the airbag still in position and largely inflated during Phase I testing versus the images from the tests in Phase II testing where the airbag is deflating and the PMHS is interacting more with the intruding door liner.
Discussion
The objective of this research was to test frail 5th percentile, elderly female PMHS in a repeatable, realistic near-side impact crash scenario. The boundary conditions for the series were primarily based on FMVSS No. 214, which features an MDB impacting the near-side occupant compartment. An impact velocity of 50 kph was selected from finite element simulations of the MDB impact as the appropriate velocity for a small female occupant to achieve a thoracic AIS level 3 injury. In addition, the door intrusion profile was based on recorded accelerometer data taken from the inner steel structure of a door from a 2016 compact vehicle impacted by an FMVSS No. 214 MDB. Prior to running both PMHS phases, a series of similar sled tests were run with the SID-IIs build level D (BLD) using the original Phase I airbag. These ATD tests resulted in a maximum thoracic deflection of 27 mm, with an average deflection of 26 mm across the three thoracic ribs, corresponding to less than a 2% chance of an AIS 3+ injury (Irwin et al. Citation2016). In addition, these values can also be compared to the IIHS ratings, which state a “good” outcome is when all rib deflections are less than 50 mm and the average peak rib deflections are less than 34 mm (IIHS, Citation2014). Overall, the ATD results, along with the published injury risk curve for the ATD, suggest that small female PMHS should not sustain serious thoracic injury from this type of near-side impact loading scenario.
Contrary to what the ATD testing would suggest, the main injury findings across all PMHS tests were numerous rib fractures, mainly in the anterior region of the thoracic cage toward the junction with the costal cartilage. The upper ribs on the left (impact) side, ribs L2, L3, and L4, were fractured in 90% of the tests. The second most frequent region of thoracic fractures was the anterior aspect of the upper right, non-impact side of each PMHS. Finally, four of the PMHS resulted in 13 or more separate rib fractures, including several fractures on the posterior region of the thorax on the impacted side. In total, eight of the ten PMHS tests, or 80%, resulted in a maximum thoracic AIS (MAIS) of 3+ despite the SID-IIs data only predicting a 2% risk. This indicates that the ATD injury risk curve is not applicable to this population that was subjected to realistic side impact scenarios. As noted, there were four clavicle fractures on the impacted side of the PMHS, which is a common injury in side impact testing and real-world near-side crashes when the glenohumeral joint is loaded through the intruding door (Bolte et al. Citation2000). In a number of the tests, the short-statured PMHS used in this study caused the shoulder to be aligned with the top of the intruding door, thus resulting in the high number of clavicle fractures (Figures A9 & A10, Supplementary material).
In this study, two chestbands, one at the level of the axilla and one at the level of the xiphoid process, were used to calculate thoracic deflections during the realistic impacts. Across both phases of testing, the maximum half-lateral axillary deflection of the PMHS averaged 21 mm with a standard deviation of 7 mm (). The SID-IIs testing completed prior to running the PMHS tests also included a chestband at the axillary level, which resulted in an average maximum half-lateral deflection of 25 mm. Overall, all but one of the PMHS measured less than 25 mm in the Phase I test series, which might point to the SID-IIs thorax being less stiff than the thorax of small, elderly females.
The deflection was normalized by initial chest breadth at both the axillary level and the xiphoid process level to calculate percent compression, as shown in . For both full- and half-lateral compression, greater compression was measured at the xiphoid level than at the axillary level. For full lateral compression for both phases, the average xiphoid maximum compression, 20%, was more than double the average maximum compression recorded at the axilla. This relationship also was true when comparing the compression values for half-lateral compression. These findings are due to the shape of the thorax as it typically expands outwards as one moves inferior along the spine resulting in the xiphoid level interacting with the safety restraints first and thus absorbing more energy. Finally, there was a clear difference in the amount of maximum compression at the level of the xiphoid between Phase I and Phase II tests. The thoracic compressions recorded in the Phase II tests were approximately half of that documented in Phase I due to the changes in the airbag which altered the timing of the restraint interactions, as shown in and .
Along with the importance of having realistic boundary conditions for this test series, it was also critical to include strain gages on the ribs to identify precisely when each rib fractured. Knowing the timing of each rib fracture makes it possible to identify the time during the event when the PMHS achieved an AIS 3 injury. Given that most thoracic injury risk curves are based on censored data, i.e., the time of achieving a level of injury was unknown, it is critical to collect data which may allow for new risk curves to be generated using non-censored data. In addition, the timing allows for the event to be analyzed to better understand the underlying mechanisms of injury from each test. shows the time at which each PMHS achieved their MAIS during the event based on rib strain gage data. During Phase I, 4 of the 5 PMHS achieved MAIS = 3 at or before 30 msec. Figure A9 (Supplementary material) reveals that during this time the occupant appears to be restrained by the belt and interacts with the airbag as intended during this crash scenario. The difference between the thoracic deflection at the level of the axilla at the time of AIS = 3 injury versus maximum deflection over the entire event is displayed in for Test 2. While the maximum half-lateral deflection for Test 2 was 31 mm, the PMHS achieved AIS = 3 at only 7 mm of half-lateral deflection, or 3% compression. The chestband contours also reveal that at the same instant in time, there was 14 mm of A/P deflection due to the pretensioner pulling the occupant back into the seat. The realistic boundary conditions in the Phase I test series revealed that in a near-side crash using a standard restraint with pretensioner and an airbag, the thorax of the occupant undergoes a period of combined loading.
To better understand the timing of critical events in the Phase I tests, shows airbag pressures, seatbelt tension at the retractor, half-lateral deflection at both the axillary and xiphoid process levels, A/P displacement at the axillary level, and the time at which the ribs fractured up to the point of AIS = 3. This exemplar plot from Test 2 revealed that AIS = 3 occurred at the point of maximum A/P deflection when the occupant just began to interact with the side airbag. The pretensioner fired at 9 msec and reached maximum load within a few msec of the peak pressure in either chamber of the side airbag. In contrast, the peak half-lateral deflections at both thoracic levels do not occur until 20 msec later in the event. Due to the standard restraint system in Phase I resulting in combined loading, the experimental c-shaped bag was used in Phase II to delay the interaction of the thorax with the airbag. The result is shown in the exemplar timing plot of . The peak pressure in the airbag is delayed roughly 10 msec allowing for the A/P loading from the pretensioner to dissipate prior to loading the thorax. While AIS = 3 injuries still occurred in 3 of the 5 tests, those injuries were more aligned with the maximum half-lateral deflection of the thorax, not the combined loading as seen in Phase I.
The need to better understand combined loading to the thorax is critical given that the majority of anthropomorphic test devices (ATDs) have been designed and built for single-axis loading, i.e., purely frontal or lateral. The historical biomechanical data used to not only design current ATDs, but also to validate finite element human body models have largely been single-axis hub impacts, or sled tests with generic load walls that simplified loading to the PMHS. These data from Phase I show that when there is combined loading from safety systems currently being used around the world, the mechanism of injury changes and simple single-axis deflection may no longer accurately predict injury. A previous study investigating rib fractures under combined loading reported that ribs fractured earlier and with less deflection compared to the side-matched rib from the same subject under A/P loading only (Agnew et al. Citation2021). That result is consistent with findings from this study, further supporting that thoracic injuries occurring due to combined loading may not be accurately predicted by conventional injury metrics.
These data also revealed that further testing is likely needed to determine the appropriateness of utilizing injuries sustained by mid-size male PMHS as well as censored data to define injury risk curves for small, female occupants. Other studies have documented that increased chest deflection could occur due to accumulating rib fractures as fractures allow for increased compression of the thorax (Kang et al. Citation2017, Kemper et al. Citation2016, Kemper et al. Citation2011). As stated, the SID-IIs BLD data from the same sled set-up predicted approximately a 2% chance of AIS 3+ injury, yet 80% of the PMHS tests resulted in MAIS = 3. When examining the axillary level half-lateral deflection of these eight sled tests at the time of AIS = 3, displacements ranged between 1-28 mm, with an average of 12.6 mm at the time of AIS = 3. If converted to thoracic compression, the injuries occurred between 0.4% and 11.6% with an average compression of only 4.4%. The only PMHS not to have any rib fractures through the series recorded a maximum half-lateral axillary deflection of 8 mm and a maximum half-lateral axillary compression of 3.2%, both of which are below the average values of PMHS that resulted in AIS = 3. It is important to remember that these PMHS were of reduced bone density (osteopenia), which was not considered in calculating the injury-risk curves from Irwin et al. (Citation2016) or in the design of the SID-IIs BLD.
Limitations
As with many studies focused on PMHS testing, the number of tests conducted was limited to only five samples for each airbag design. Biomechanical response and injury tolerance have been shown to vary substantially between individuals, likely due to differences in age, anthropometry, thoracic geometry, and skeletal material properties. While the inclusion criteria limited the height and mass of the PMHS tested to be similar to a 5th percentile female, the data were not further normalized during the analysis. Despite subjects being selected to meet the clinical standard for osteopenia (-2.5 < aBMD t-score < -1.0), the actual relationship of BMD with fracture risk is inconsistently reported and debated in the literature. In a scoping review of previous PMHS studies utilizing BMD, it was found that 78% of articles (7/9) found no relationships between aBMD and injury outcomes (Haverfield et al. Citation2022) as also seems to be the case in the current study. BMD in the ribs (the focus here), specifically, has not been explored in the context of predicting or explaining rib fracture risk, and a recent study identified significant variation in BMD between skeletal sites suggesting that site-specific (e.g., ribs) evaluation of bone quality is necessary in future work (Haverfield et al. Citation2022) and will be critical in order to evaluate thoracic vulnerability. Furthermore, some subjects fell outside the targeted inclusion range for aBMD (t-scores and z-scores), particularly in Phase II, but there appear to be no clear trends between aBMD in these subjects and rib fractures (e.g., PMHS 9 and 10 had the same t-scores, but sustained 19 and 0 rib fractures, respectively).
The ASIS used to represent door intrusion during the sled series was not perfectly repeatable due to its use on the HyGE sled. Although there was up to 5 cm of difference in intrusion for cylinders 1 and 3 for two of the tests, the resulting injuries did not appear to be related to the differences in intrusion. Finally, head and neck injuries were not included in this study due to not using a side curtain airbag. The use of the side curtain airbag was not considered critical when considering thoracic response and injury mechanisms.
Supplemental Material
Download Zip (3.6 MB)Acknowledgements
We would like to thank the anatomical donors. Without these selfless gifts, it would not be possible to conduct this research. Also thank you to all students and staff of the Injury Biomechanics Research Center (IBRC) who assisted with the sled test series.
Funding
The authors would like to thank NHTSA and Autoliv for sponsoring this research.
References
- Agnew A, Sreedhar A, Bolte J, Kang Y. 2021. Exploratory comparison of human rib structural behavior in two dynamic loading scenarios. IRCOBI. IRC-21-92: 775-786.
- Baudrit P, Petitjean A, Potier P, Trosseille X, Vallencien G. 2014. Comparison of the thorax dynamic responses of small female and midsize male post mortem human subjects in side and forward oblique impact tests. Stapp Car Crash J. 58:103–121.
- Bolte IJ, Hines MH, McFadden JD, Saul RA. 2000. Shoulder response characteristics and injury due to lateral glenohumeral joint impacts. Stapp Car Crash J. 44:261–280. doi: 10.4271/2000-01-SC18.
- Cavanaugh J, Zhu Y, Huang Y, King A. 1993. Injury and response of the thorax in side impact cadaveric tests. SAE Technical Paper 933127. doi: 10.4271/933127.
- Haverfield Z, Hunter RL, Loftis KL, Agnew AM. 2022. Skeletal site and method-dependent variability of bone mineral density in injury biomechanics research. IRCOBI. IRC-22-49: 332-360.
- Insurance Institute for Highway Safety. 2014. Side impact crashworthiness evaluation; Guidelines for rating injury measures; Version III. Arlington, VA: Insurance Institute for Highway Safety.
- Irwin A, Crawford G, Gorman D, Wang S, et al. 2016. Thoracic injury risk curves for rib deflections of the SID-IIs build level D. SAE Technical Paper 2016-22-0016. doi: 10.4271/2016-22-0016.
- Kang Y, Agnew A, Icke K, Bolte J. 2017. Elderly PMHS thoracic responses and injuries in frontal impacts. IRCOBI. IRC-17-69: 539-557.
- Kanis J, Melton J, Christiansen C, Johnston C, Khaltaev N. 1994. The diagnosis of osteoporosis. J Bone Miner Res. 9(8):1137–1141. doi: 10.1002/jbmr.5650090802
- Kemper AR, Kennedy EA, Mcnally C, Manoogian SJ, Stitzel JD, Duma SM. 2011. Reducing chest injuries in automobile collisions: rib fracture timing and implications for thoracic injury criteria. Ann Biomed Eng. 39(8):2141–2151. doi: 10.1007/s10439-011-0311-8
- Kemper AR, Beeman SM, Porta DJ, Duma SM. 2016. Non-censored rib fracture data during frontal PMHS sled testing. Traffic Inj Prev. 17(sup1):131–140. doi: 10.1080/15389588.2016.1203069
- Kimpara H, Iwamoto M, Miki K, Lee J, Begeman P, Yang K, King A. 2003. Biomechanical properties of the male and female chest subjected to frontal and lateral impacts. IRCOBI. 5(1):235–247.
- Kinoshita A, Shigeno N, Fukushima T. 2011. Development of a side impact sled test method using multiple actuators. 22th ESV. 11–72.
- Kong JS, Kim OH, Youk H, Lee HY, Kang CY, Sung S, Jang JY, Woong Yoon T, Lee KH. 2018. Analysis of injury mechanism of the elderly and non-elderly groups in minor motor vehicle accidents. Traf Inj Prev. 19(sup2):S151–S153. doi: 10.1080/15389588.2018.1532210
- Kuppa S, Eppinger R, McKoy F, Nguyen T, Pintar F, Yoganandan N. 2003. Development of side impact thoracic injury criteria and their application to the modified ES-2 dummy with rib extensions (ES-2re). Stapp Car Crash J. 47:189–210.
- Maltese M, Eppinger R, Rhule H, Donnelly B, Pintar F, Yoganandan N. 2002. Response corridors of human surrogates in lateral impacts. Stapp Car Crash J. 46:321–351. doi: 10.4271/2002-22-0017
- Mertz H, Irwin A, Prasad P. 2003. Biomechanical and scaling bases for frontal and side impact injury assessment reference values. Stapp Car Crash J. 47:155–188. doi: 10.4271/2003-22-0009
- Morgan R, Marcus J, Eppinger R. 1986. Side impact: the biofidelity of NHTSA’s proposed ATD and efficacy of TTI. 30th Stapp Car Crash Conference. doi: 10.4271/861877. doi: 10.4271/861877
- National Highway Traffic Safety Administration (NHTSA). 2022. Older population: 2020 data, traffic safety facts. 2022. Available at: https://crashstats.nhtsa.dot.gov/Api/Public/ViewPublication/813341. Accessed November 1, 2022.
- Pintar F, Yoganandan N, Sances A, Eppinger R. 1996. Instrumentation of human surrogates for side impact. Proc. Society of Automotive Engineers. 40th Stapp Car Crash Conference. 29–42.
- Pintar F, Humm J, Yoganandan N, Martin P. 2009. Test program to define oblique chest loading in side impact. 21st ESV:09–0057.
- SAEJ211. 2014. Instrumentation for impact test—Part 1: electronic instrumentation. SAE paper no. J211/1_201403. SAE International.
- Sugaya H, Takahashi Y, Ayyagari M, Gomez J, Whitcomb B, Markusic C, Ramachandra R, Kang YS, Agnew A, Bolte J. IV. 2019. Identification of accident representative scenario for elderly female occupants in side impact. IJAE. 10(2):150–155. doi: 10.20485/jsaeijae.10.2_150
- Sunnevång C, Rosén E, Boström O, Lechelt U. 2010. Thoracic injury risk as a function of crash severity – car-to-car side impact tests with WorldSID compared to real-life crashes. Ann Adv Automot Med. 54:159–168.
- Wood L, Miller C, Madura N, Reed M, Schneider L, Klinich K, Rupp J. 2014. Response and tolerance of female and/or elderly PMHS to lateral impact. Stapp Car Crash J. 58:423–463. doi: 10.4271/2014-22-0015
- Yoganandan N, Pintar F. 2005. Deflection, acceleration, and force corridors for small females in side impacts. Traffic Inj Prev. 6(4):379–386. doi: 10.1080/15389580500256888
- Yoganandan N, Humm J, Pintar F, Brasel K, Rudd R, Ridella S. 2012. Thoraco-abdominal deflection responses of post mortem human surrogates in side impacts. Stapp Car Crash J. 56:49–64. doi: 10.4271/2012-22-0002
- Yoganandan N, Pintar FA, Humm JR, Hallman JJ, Maiman DJ. 2011. Analytical and experimental data of chest deflections and injuries in side impacts. 22nd ESV:11–0256.