Abstract
Objective: One potential nonstandard seating configuration for vehicles with automated driving systems (ADS) is a reclined seat that is rear-facing when in a frontal collision. There are limited biomechanical response and injury data for this seating configuration during high-speed collisions. The main objective of this study was to investigate thoracic biomechanical responses and injuries to male postmortem human subjects (PMHS) in a rear-facing scenario with varying boundary conditions.
Method: Fourteen rear-facing male PMHS tests (10 previously published and 4 newly tested) were conducted at two different recline angles (25-degree and 45-degree) in 56 km/h frontal impacts. PMHS were seated in two different seats; one used a Fixed D-Ring (FDR) seat belt assembly and one used an All Belts To Seat (ABTS) restraint. For thoracic instrumentation, strain gages were attached to ribs to quantify strain and fracture timing. A chestband was installed at the mid-sternum level to quantify anterior-posterior (AP) chest deflections. Data from the thorax instrumentation were analyzed to investigate injury mechanisms.
Results: The PMHS sustained a greater number of rib fractures (NRF) in the 45-degree recline condition (12 ± 7 NRF for ABTS45 and 25 ± 18 NRF for FDR45) than the 25-degree condition (6 ± 4 NRF for ABTS25 and 12 ± 8 NRF for FDR25), despite AP chest compressions in the 45-degree condition (−23.7 ± 9.4 mm for ABTS45 and −39.6 ± 11.9 mm for FDR45) being smaller than the 25-degree condition (−38.9 ± 16.9 mm for ABTS25 and −55.0 ± 4.4 mm for FDR25). The rib fractures from the ABTS condition were not as symmetric as the FDR condition in the 25-degree recline angle due to a belt retractor structure located at one side of the seatback frame. Average peak AP chest compression occurred at 45.7 ± 3.4 ms for ABTS45, 45.6 ± 3.1 ms for FDR45, 46.7 ± 1.9 ms for ABTS25, and 46.9 ± 2.3 ms for FDR25. Average peak seatback resultant force occurred at 43.9 ± 0.9 ms for ABTS45, 44.6 ± 0.8 ms for FDR45, 42.5 ± 0.2 ms for ABTS25, and 41.5 ± 0.5 ms for FDR25. The majority of rib fractures occurred after peak AP chest compression and peak seatback resultant force likely due to the ramping motion of the PMHS, which might create a combined loading (e.g., AP deflection and upward deflection) to the thorax. Although NRF in the 45-degree reclined condition was greater than the 25-degree recline condition, similar magnitudes of rib strains were observed regardless of seat and restraint types, while strain modes varied.
Conclusions: The majority of rib fractures occurred after peak AP chest compression and peak seatback force, especially in FDR25, ABTS45, and FDR45, while the PMHS ramped up along the seatback. AP chest compression, seatback load, and strain measured along the rib could not explain the greater NRF in the 45-degree recline conditions. A complex combination of AP chest deflection with upward deflection was discovered as a possible mechanism for rib fractures in PMHS subjected to rear-facing frontal impacts in this study.
Introduction
Unconventional seating configurations may be incorporated in interior cabin designs in future vehicles equipped with automated driving systems (ADS), and numerous variations have been suggested and reported by consumer surveys (Jorlöv et al. Citation2017; Koppel et al. Citation2019; Östling and Larsson Citation2019). In these surveys, rear-facing configurations have been proposed as desirable, in particular for long-distance travel with children and family members (Jorlöv et al. Citation2017; Koppel et al. Citation2019). However, information regarding occupant safety and injury risk in rear-facing seating configurations in high-speed frontal impacts is very limited in the literature. Therefore, biomechanical responses and assessment of human injury risks in the rear-facing seat configuration in high-speed frontal impact conditions will help develop safety and restraint systems for rear-facing occupants.
Recently, a series of postmortem human subject (PMHS) sled tests was conducted in a high-speed rear-facing frontal impact at a change in velocity (ΔV) of 56 km/h to explore biomechanical responses and injuries that can be used to enhance anthropomorphic test devices (ATDs) and computational human body models (HBMs) (Kang et al. Citation2020; Kang et al. Citation2022). These studies investigated the effect of two seatback recline angles (25 and 45 degrees), two original equipment manufacturer (OEM) seats, and two seat belt conditions, an All Belts To Seat (ABTS) condition and a standard Fixed D-Ring (FDR) condition. PMHS injuries reported in both studies included minor cervical spine injuries, upper extremity injuries (e.g., scapula fractures due to the retractor structure installed on the seatback frame; clavicle fractures due to excessive ramping up motion in the 45-degree condition; ulna fractures due to flailing of the forearm), as well as lower extremity injuries (e.g., fibula and distal tibia fractures due to interaction with the front portion of the seat pan). AIS3+ injuries sustained by the PMHS included rib and pelvis fractures due to interaction with the seatback. In particular, rib fractures were the most common injury. All six PMHS tested by Kang et al. (Citation2020) and three out of four PMHS from Kang et al. (Citation2022) sustained thoracic injuries resulting in Maximum Abbreviated Injury Scale (MAIS) 3 due to multiple rib fractures during pocketing and ramping up phases.
The literature is very sparse regarding thoracic responses and injuries (e.g., rib fractures) in rear-facing or rear-impact scenarios. Viano and Parenteau (Citation2008) analyzed National Automotive Sampling System-Crashworthiness Data System (NASS-CDS) data to search for rear crashes that resulted in serious injuries. They found that serious injuries (AIS3+) to the thorax could occur in rear impacts (ΔV range from 18 to 73 km/h) likely due to high acceleration in an upright supported seat or posterior-to-anterior loading to the seatback due to intrusion from the second-row seating area. Zellmer et al. (Citation2018) searched high-speed rear-impact cases in the German In-Depth Accident Study (GIDAS) and found that occupants sustained thoracic injuries such as rib fractures and contusions (Zellmer et al. Citation2018). A few studies have investigated thoracic responses and injuries in posterior-to-anterior hub impact conditions (Viano et al. Citation2001; Parenteau et al. Citation2021). These studies used a 23.4 kg hub impactor and tested eight PMHS at 4.5 m/s and 6.7 m/s to generate biomechanical response corridors in posterior-to-anterior hub impact conditions. Five out of eight PMHS sustained posterior or lateral rib fractures. Although force-deflection data from these impact tests should help improve biofidelity of the posterior-anterior thoracic response for ATDs and HBMs in a highly repeatable setup, simplified hub impacts cannot replicate PMHS inertial loading of the thorax into the seatback. This inertial loading should be more distributed than the localized hub loading.
Furthermore, many previous studies could not identify rib fracture timing due to limited availability and installation techniques of rib and thorax instrumentation. Instrumentation techniques that provide specific rib fracture timing and rib strain are crucial to understanding injury mechanisms of the thorax and ultimately create more conservative and realistic injury risk curves and thresholds (Duma et al. Citation2011; Kemper et al. Citation2016; Kang et al. Citation2017; Murach et al. Citation2018; Shurtz et al. Citation2018). Recent rear-facing PMHS studies by Kang et al. (Citation2020 & 2022) installed strain gages at multiple rib locations, providing a clear link between rib fracture timing and corresponding whole body PMHS kinematics at ΔV of 56 km/h. However, a detailed analysis of rib strain magnitude and strain mode changes that may explain PMHS thoracic response and injury in high-speed rear-facing frontal impacts has not been conducted.
Therefore, the main objectives of this study were (1) to explore rib fracture location, distribution, and frequency with respect to chest deflections and (2) to quantify rib strain magnitudes and modes. In this way, the relationship between rib strains and rib fractures can be established to better understand potential injury mechanisms of the thorax in the rear-facing frontal-impact condition.
Methods
Rear-facing frontal-impact sled tests of 14 male PMHS were conducted at ΔV of 56 km/h (). Ten PMHS were tested previously (Kang et al. Citation2020; Kang et al. Citation2022, hereafter referred to as Kang studies), and four additional PMHS were evaluated in an identical sled setup and input acceleration (37 g and ΔV of 56 km/h as shown in Figure A1). PMHS were obtained through The Ohio State Whole Body Donation Program in accordance with all NHTSA and University guidelines on the ethical use of anatomical donationsFootnote1. Two different OEM seats (ABTS or FDR) in two different recline positions (i.e., seatback angle was limited to 25 or 45 degrees) were used in this study (hereafter referred to as ABTS25, ABTS45, FDR25, and FDR45). Six PMHS were tested in the ABTS condition, while eight PMHS were tested in the FDR condition. shows the test configuration for each PMHS. At least three PMHS were tested in each condition ().
Figure 1. Rib fracture location, frequency, and average timing for (a) ABTS45, (b) ABTS25, (c) FDR45, and (d) FDR25.
A: anterior region of the rib, L: lateral region of the rib, P: posterior region of the rib, L1-L12: left side 1st rib through 12th rib, R1-R12: right side 1st rib through 12th rib, Y: no fractures, G: fracture occurred in one PMHS, O: fracture occurred in two PMHS, R: fracture occurred in three PMHS, B: fracture occurred in four PMHS, Numeric values: average fracture timing (ms) using available strain gage data
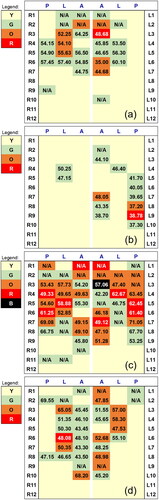
Table 1. PMHS characteristics and test matrix.
Experimental setup
The general setup for the ABTS (2018 Honda Odyssey manual seat) and FDR (2018 Honda Accord manual seat) conditions was described in the Kang studies (ABTS in Kang et al. Citation2020 and FDR in Kang et al. Citation2022). A new seat and seat belt were used for each test. In brief, reinforced frames were added behind the seatback, as shown in Figures A2 and A3, given current OEM seats were not designed to be durable and repeatable in high-speed rear-facing frontal impacts. Six load cells were installed between the reinforced frame and seatback to quantify occupant and seatback loads (Figure A2a and c). Load cell plates without the seats are shown in Figure A3a and b. The seatback dimensions of the OEM seats with respect to the load cell plate are also provided in Figure A3c (ABTS) and d (FDR). The ABTS has a retractor assembly installed on the left side of the seatback (Figure A4a), while the FDR has a more distributed and symmetric seatback support frame with thin seatback suspension (e.g., serpentine springs) in the middle (Figure A4b). Further detailed information on the experimental setup can be found in the Kang studies (Kang et al. Citation2020, Citation2022).
Figure 2. Average peak AP chest compression and timing. Error bars represent one standard deviation.
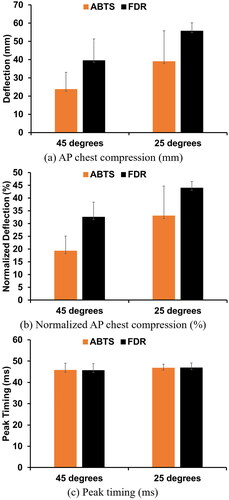
PMHS characteristics, instrumentation, and positioning procedure
shows a summary of PMHS characteristics. The fourteen male PMHS used in this study ranged in age from 53 to 71 years (average age of 60.9 ± 4.6). The PMHS average height of 176.0 ± 4.8 cm and weight of 80.0 ± 12.7 kg were consistent with accepted 50th percentile male demographics (Mertz et al. Citation2001). The average seated height was slightly taller than the 50th percentile male (94.3 cm vs. 90.7 cm). Dual-Energy X-ray Absorptiometry (DEXA) was used to quantify areal bone mineral density (aBMD), and lumbar aBMD T-scores ranged from −1.6 to 1.9 (). Since this study focuses on only thoracic response and injuries, additional thoracic anthropometry is provided in Table A1. For PMHS instrumentation, chest deflection was determined using a chestband installed at the mid-sternum level. To secure the chestband to the PMHS thorax, the chestband was stitched to the skin at four locations (anterior, two lateral, and posterior locations at the mid-sternum level). Uni-axial strain gages were secured on the periosteal surface of the cutaneous cortex of the anterior (ribs 3 through 9) and posterior (ribs 3 through 10) aspects of the rib. Additional instrumentation was installed in other body regions (Kang et al. Citation2020 & 2022), but will not be discussed as this study focuses only on results from thoracic instrumentation. PMHS were positioned on the seats following the same procedure outlined in the Kang studies (Kang et al. Citation2020 & 2022). The PMHS positioning targets were determined by using a regression model proposed in a previous volunteer study (Reed et al. Citation2019). The initial tensions for the shoulder and lap belts were set to 17.8 N and 26.7 N, respectively. More detailed PMHS positioning information can be found in the original Kang studies.
Data collection, reduction, and analysis
A data acquisition system (SLICE PRO, DTS, Seal Beach, CA, USA) was utilized to collect all PMHS data using a sampling rate of 20,000 Hz with 4,000 Hz anti-aliasing filter. Time zero was defined at 0.5 g of the sled acceleration. The same filtering techniques used in the Kang studies were also applied here. Strain gage and chestband data were not filtered (Kemper et al. Citation2016). A custom post-processing program, Crashstar, was used to calculate PMHS chest deflections. Anterior-posterior (AP) chest compression (i.e., negative deflection) and expansion (i.e., positive deflection) were determined using chestband data (i.e., sternum gage - spine gage). Normalized chest deflections were determined using the half-deflection method (i.e., deflection divided by half the initial chest depth) also used in the Kang studies. Unlike the Kang studies (i.e., only peak strain rate was used), both strains and strain rates were used to identify the timing of rib fractures. Strain rates were inspected first to identify if ribs experienced high strain rate, and then rib fracture timing was determined where a strain drop-off occurred in the strain data. Strain rates were computed using a numerical differentiation method (i.e., forward difference) in MATLAB (The MathWorks, Inc, Natick, MA, USA). Exemplar plots for strain and its rate to determine fracture timings for each test condition are shown in Figures A5–A8. To summarize the rib fractures for each test condition, tables were created to visualize rib fracture locations, frequencies, and timings for each rib level and aspect (). Given the sample size was too small (N = 3 for each ABTS recline angle and N = 4 for each FDR recline angle) to achieve meaningful statistical outcomes, data from each testing configuration were compared using descriptive statistics (e.g., mean and standard deviation).
Results
AP chest deflection
Average peak AP chest compressions for each test condition are shown in . For both ABTS and FDR conditions, average AP chest compressions from the 25-degree conditions (−38.9 ± 16.9 mm for ABTS25 and −55 ± 4.4 mm for FDR25) were higher than those from the 45-degree conditions (−23.7 ± 9.4 mm for ABTS45 and −39.6±.11.9 mm for FDR45) shown in . Similar trends were found in the normalized AP chest compressions (). When comparing ATBS to FDR, the average and normalized peak AP chest compressions from the FDR conditions were higher than the ABTS conditions regardless of the different recline angles, implying the ABTS dissipated energy more effectively than the FDR. Timing for peak compressions was consistent between conditions shown in (45.7 ± 3.4 ms for ABTS45, 45.6 ± 3.1 ms for FDR45, 46.7 ± 1.9 ms for ABTS25, and 46.9 ± 2.3 ms for FDR25). Peak AP chest compression and its timing for each PMHS are shown in Table A2. Chest contours at peak compression, the number of rib fractures (NRF), and the number of fractured ribs (NFR) for each PMHS are also provided in Table A3 and Figures A9 and A10. Chest contours from the ABTS conditions had more asymmetric deflection than the FDR, due to the difference in seatback geometry shown in Figure A4.
Seatback load
Peak seatback sum resultant load and timing are provided in Table A4. Peak seatback loads in the 45-degree recline conditions (26.0 ± 3.0 kN for ABTS45 and 32.5 ± 3.7 kN for FDR45) were smaller than the 25-degree recline conditions (36.1 ± 6.5 kN for ABTS25 and 45.9 ± 4.3 kN for FDR25). Peak seatback loads in the 25-degree recline conditions occurred earlier (42.5 ± 0.2 ms for ABTS25 and 41.5 ± 0.5 ms for FDR25) than the 45-degree recline conditions (43.9 ± 0.9 ms for ABTS45 and 44.6 ± 0.8 ms for FDR45), but the difference was less than 3 ms. It should be noted that the retractor structure in the ABTS seatback shown in Figure A4 caused asymmetric seatback loads, which were not observed in the FDR condition (Figure A11).
Rib fracture location, frequency, and timing
shows rib fracture locations, frequency, and average fracture timing resulting from ABTS () and FDR () conditions. A detailed injury summary for newly tested PMHS is provided in Table B1, while similar injury information for the other PMHS is provided in the Kang studies. For both ABTS and FDR conditions, PMHS sustained a greater NRF in the 45-degree compared to the 25-degree recline seatback angle (Table A3). This is likely due to excessive ramping of the PMHS up the reclined seatback (Table A5 and Figure B1) and upward movement of the abdominal contents based on qualitative analysis in Figure B2, which might generate combined loading (e.g., inertial loading components of the thorax and combined chest deflections as shown in Figure A12). Figures B3 and B4 show exemplary sequential whole-body kinematics of the PMHS for FDR45 and FDR25.
For ABTS25, more rib fractures occurred on the left side than the right side, particularly in the posterior region of the ribs, due to interaction with the retractor housing installed in the seatback frame (Figure A4a). However, rib fractures on the left posterior aspect were not observed in the PMHS tested in ABTS45. More rib fractures occurred in the FDR conditions than the ABTS conditions (). Unlike the ABTS seatback, the symmetric geometry of the FDR seatback structure (Figure A4b) resulted in more evenly distributed rib fracture patterns as shown in . For FDR45, rib fractures occurred on all regions (anterior, lateral, and posterior), while most rib fractures occurred only in the anterior and lateral regions in FDR25.
For the rib fracture timing in ABTS25 (), posterior rib fractures occurred earlier than anterior and lateral rib fractures, implying posterior fractures were induced directly from the interaction of the retractor structure while the PMHS translated into the seatback. This is supported because all posterior fractures shown in occurred earlier than peak AP chest compression timings (46.7 ± 1.9 ms from ABTS25 in and Table A2) and peak seatback load timings (42.5 ± 0.2 ms from ABTS25 in Table A4), while most anterior and lateral fractures occurred after peak chest deflections and peak seatback loads during the ramping motion and visual upward movement of the abdominal contents into the PMHS chest in ABTS25. The rib fracture timing with the NRF and the NFR for each PMHS are also provided in Figures A13 and A14. In ABTS25, only 31% of fractures occurred after peak AP chest compression (Figure A13). However, the majority of rib fractures occurred after peak AP chest compression in FDR25, ABTS45, and FDR45 conditions (Figures A13 and A14). Based on the available intact strain gage data (i.e., broken gages were excluded in this analysis), 69% of fractures in FDR25, 68% of fractures in ABTS45, and 98% of fractures in FDR45 occurred after the peak AP chest compressions determined from each testing condition. This implies that rib fractures in the high-speed rear-facing conditions occurred not only due to AP chest compression induced by the inertia of the thorax but also due to AP chest expansion and likely an upward loading during the ramping motion and upward movement of the abdominal contents into the thorax (Figure B2), which was subsequent to peak AP chest compression.
Rib strain
shows average peak strains for each condition. For the ABTS conditions, all peak strains measured from left posterior gages were in compression, while those measured from the other locations were in tension (), regardless of the recline angles. The compressive strains in the left posterior region and asymmetric peak strain patterns were due to the interaction with the retractor structure in the ABTS conditions and could also be explained by asymmetric seatback load distribution (Figure A11a and b), Unlike the ABTS conditions, more evenly distributed strain patterns were observed in the FDR conditions. It should be noted that the PMHS sustained more rib fractures in ABTS45, even though average peak strains were consistent with those from ABTS25 (strain ranged from −0.93 to 1.03% for ABTS45 and from −0.89 to 1.24% for ABTS25). This suggests there were other strain directions (e.g., off-axis strain) that influenced rib fractures in the rear-facing frontal-impact scenarios.
For the FDR conditions, strain modes for the posterior strain gages were in tension in FDR45, which was inconsistent with the compression mode in FDR25. Excessive ramping in FDR45 minimized the interaction of the seatback (Figure B1) and could have caused the tensile strain on the posterior ribs. Peak average strains ranged from 0.21% to 1.05% in FDR45 and −0.39% to 1.03% in FDR25. Peak average tensile strains were similar in both FDR45 and FDR25, even though PMHS tested in FDR45 sustained a greater NRF. No obvious trend was observed between the average peak strains and the NRF, regardless of seat type and recline angle.
Discussion
This study focused on (1) investigating rib fracture location, distribution, and frequency and (2) exploring rib strains and strain modes, in order to understand potential injury mechanisms of the thorax in the rear-facing frontal impact condition. Regardless of the type of seat and restraint, lower AP chest compressions and more rib fractures were found in the 45-degree recline conditions than the 25-degree recline conditions. However, the peak strains measured from the 45-degree conditions were consistent with the 25-degree conditions, indicating strains that affected rib fractures might be occurring in directions that were not captured by the uniaxial strain gage installed along the ribs. Posterior rib fractures that were related to asymmetric strain modes and seatback loads were found in ABTS25, due to the interaction of the retractor structure in the seatback, while evenly distributed fractures and strain modes were observed in the FDR conditions. The PMHS sustained many rib fractures after peak AP chest compression, in particular in FDR25, ABTS45, and FDR45 conditions.
Rib fracture in 45-degree configuration
Thorax injuries (e.g., rib fractures) in high-speed rear impact conditions have been identified in crash databases (Zellmer et al. Citation2018; Viano and Parenteau Citation2008). However, it is still unclear how recline angles of the seatback influence rib fracture risks in high-speed rear-facing configurations. The results from the current study showed that peak chest deflections were greater in the 25-degree conditions than the 45-degree conditions. However, the NRF was greater in the 45-degree conditions, which indicates that AP chest compression may not be an appropriate injury predictor in the high-speed rear-facing frontal impact, especially for reclined seatback conditions. Interestingly, in the 45-degree conditions, most fractures occurred after peak AP chest compression while abdominal contents visually translated into the thoracic cavity induced from the ramping up of the PMHS along the seatback (Figures B1 and B2). This suggests a new potential rib fracture mechanism to be considered: combined loading induced from AP chest deflection and upward deflection (Figure A12). To provide visualization of the potential combined loading, an exemplar chest contour, time-history plots of the AP chest deflection, shoulder belt tension, seatback resultant force, PMHS sequential motions, and accumulated fracture information for PMHS05 are provided in . It should be noted that the shoulder belt tension was less than 500 N when the PMHS sustained rib fractures (). Peak AP chest compression occurred at 42.95 ms, but the PMHS had sustained no rib fractures. The rib fractures occurred between 51.20 ms and 60.10 ms while the PMHS chest expanded (i.e., AP chest deflection returned to 0 mm and even became tensile deflection) likely due to the upward movement of the abdominal contents shown in Figure B2 and the PMHS ramping up the seatback shown in . The PMHS thorax and ribs would therefore experience upward deflection and loading this far into the event. A similar trend was observed in all 45-degree PMHS tests regardless of the seat type (Figures A13 and A14). Table A2 shows the AP chest deflection at the last rib fracture times of each PMHS. AP chest deflections determined at the last rib fractures were tension (i.e., positive chest deflection) for all PMHS tested in 45-degree recline conditions, except PMHS04. The results from the 45-degree conditions exhibited lower AP chest compressions and similar rib strains but more rib fractures compared to 25-degree conditions, suggesting that the conventional AP chest deflection measures (i.e., one-dimensional measures) and uniaxial strain along the rib may be too oversimplified to explain complex rib fracture mechanisms in reclined conditions. Future work could consider multidirectional deflection and strain measures in high-speed rear-facing scenarios in order to confirm the combined loading injury mechanism (Figure A12).
Rib fracture in 25-degree configuration
In ABTS25, all posterior rib fractures occurred on the left side and before peak AP chest compression based on strain gage data, while approximately half of the rib fractures in the anterior and lateral regions occurred after peak AP chest compression. Compressive strains were observed in the posterior rib fractures, while tensile strains were recorded in the anterior rib fractures in ABTS25. Interestingly, in ABTS45, posterior fractures occurred on the right side as opposed to those on the left side in ABTS25, while the number of posterior rib fractures was fewer in ABTS45 than ABTS25. Posterior fractures from ABTS45 occurred after peak AP chest compression (Figure A13) and exhibited tensile strain, as opposed to the compressive strain in ABTS25 (), indicating a difference in the posterior rib fracture mechanisms between ABTS25 and ABTS45 conditions. The posterior fractures in ABTS25 were due to direct interaction with the retractor structure, while those in ABTS45 were due to a more distributed but complex combined loading, again likely from rearward and upward motion of the abdominal contents during ramping of the PMHS.
It should be noted that very few posterior rib fractures and relatively smaller posterior strains were found in FDR25 compared to ABTS25 due to different seatback structures and foam properties. However, more anterior and lateral rib fractures were found in FDR25 than ABTS25. In FDR25, approximately 69% of rib fractures occurred after the peak AP chest compressions as the PMHS ramped up along the seatback (see an exemplar chest contour, AP chest deflection, seatback sum load, and shoulder belt tension time-history plots, PMHS sequential motions, and fracture information in Figure B5). Therefore, the combined loading injury mechanism could also influence the injury outcomes in FDR25. However, the influence of the seatback properties on rib fractures cannot be overlooked in the 25-degree conditions. Kang et al. (Citation2022) found the FDR seatback properties were softer than the ABTS based on the acceleration measured from the thoracic vertebrae and the force from the seatback load cells. Therefore, the influence of the seatback properties and designs on rib fracture risk, as well as the combined loading mechanism, should be explored further in the future. One PMHS (PMHS21) tested in FDR25 did not sustain any rib fractures. Given that chest deflection from PMHS21 was consistent with those from the other PMHS tested in the same condition, the injury outcome might be affected more by human variation. PMHS21 was the youngest and lightest PMHS evaluated in FDR25 but had a similar aBMD (L2-L4) as the other three PMHS (), which has been shown not to predict rib properties well (Agnew et al. Citation2018). Future studies should include more comprehensive measures that correlate with rib fracture risk. Specifically, age, weight, bone quality, structural and material variations, thorax shape, and rib angle of the PMHS are crucial factors to explore in the future.
Limitations
A total of 14 rear-facing sled tests were conducted at ΔV of 56 km/h using 14 PMHS. Since two different seats and two different recline seatback angles were used, the sample size per condition was somewhat small. However, since AP chest deflections, rib strains, and corresponding injury outcomes are limited in the literature, particularly for reclined scenarios, this study could assist to better understand thoracic injuries and responses in high-speed rear-facing scenarios.
The sled pulse was based on closing speed and duration derived from acceleration data from 267 crash tests from the U.S. New Car Assessment Program (US NCAP); however, the sled pulse shape could not replicate a typical acceleration shape from the US NCAP tests due to the complexity of acceleration signals. Therefore, a half-sine wave was used to simplify the sled pulse (Kang et al. Citation2020). The US NCAP pulse was used for simulating rear-facing frontal impact scenarios, such as when crash avoidance features do not function as intended. Therefore, the input loading to the PMHS might be extreme, suggesting the injuries sustained by PMHS in this study could be severe.
The seat was rigidized to avoid unpredictable permanent deformations of the seat during the event, which could lead to an inconsistent and non-repeatable experimental setup. However, NRF and NFR from this study could be overrepresented from this rigidized experimental setup. Although the rigidized seat conditions should provide useful information for assessing biofidelity of safety tools (e.g., ATDs and HBMs), various seats, including a non-rigidized seat, should be explored to determine definitive thoracic injury criteria and risk curves for realistic rear-facing scenarios. Therefore, more PMHS tests would be required to confirm the feasibility and applicability of the combined loading mechanism to various seats. It would be useful to employ validated ATDs and HBMs to help understand the thoracic injury mechanisms and potential countermeasures.
PMHS lungs were not pressurized; therefore, thoracic responses and injuries could be overestimated due to the absence of pressure in the PMHS lungs. The influence of lung pressurization on thoracic responses and injuries in rear-facing frontal impacts is not well understood and should be explored further in the future.
Chest deflection was measured using a 59-channel chestband. If the chestband experienced local deformation (e.g., high curvature or local corrugation usually in frontal thorax impacts) during the events, the chestband might produce inaccurate chest deflection measurements (Bass et al. Citation2000; Hagedorn and Pritz Citation1991). However, the PMHS thoraces experience more distributed loading in the rear-facing scenarios compared to frontal thoracic impacts (e.g., thoraces experiencing local deformations induced by the seatbelt). In addition, a maximum gage chestband (e.g., 59 channels with 12.7 mm and 25.4 mm of gage spacing) was used to reduce errors from gage spacing. However, no studies have yet evaluated the chestband validity in rear-facing nor rear-impact conditions, which could be investigated in future studies.
The use of strain gages allowed for identifying rib fracture timings and providing important non-censored biomechanical data (Duma et al. Citation2011; Kemper et al. Citation2016) and was further strengthened by installing them at various locations in the current study. Although extensive instrumentation was utilized in an attempt to generate useful biomechanical data in this study, even more instrumentation to measure multi-directional responses on the thorax should be considered in future studies. Specifically, strain rosettes could be attached to ribs to further understand complex strain patterns and modes of PMHS thoraces in rear-facing conditions. Additionally, quantifying chest deformations in the vertical direction could also help better understand the potential combined loading injury mechanism.
Supplemental Material
Download Zip (4.1 MB)Acknowledgments
We would like to thank the anatomical donors. Without these selfless gifts, it would not be possible to conduct this research. This study would not have been possible without the contributions of students, staff, and faculty at the Injury Biomechanics Research Center.
Additional information
Funding
Notes
1 The research protocol for this study was approved by The Ohio State University Body Donation Program Advisory Committee, our internal ethics committee on April 6, 2021, before any testing was conducted.
Reference
- Agnew AM, Murach MM, Dominguez VM, Sreedhar A, Misicka E, Harden A, Bolte J, Moorhouse K, Stammen J, Kang Y. 2018. Sources of variability in structural bending response of pediatric and adult human ribs in dynamic frontal impacts. Stapp Car Crash J. 62:119–192. doi: 10.4271/2018-22-0004
- Bass C, Wang C, Crandall J. 2000. Error analysis of curvature-based contour measurement devices. Paper presented at: SAE World Congress; Detroit Michigan, USA. doi: 10.4271/2000-01-0054.
- Duma SM, Kemper AR, Stitzel JD, McNally C, Kennedy EA, Matsuoka F. 2011. Rib fracture timing in dynamic belt tests with human cadavers. Clin Anat. 24(3):327–338. doi: 10.1002/ca.21130.
- Hagedorn A, Pritz H. 1991. Evaluation of Chest Deformation Measurement Band, NHTSA, DOT HS-807-838.
- Jorlöv S, Bohman K, Larsson A. 2017. Seating positions and activities in highly automated cars – a qualitative study of future automated driving scenarios. Paper presented at: International Research Council on Biomechanics of Injury (IRCOBI); Antwerp, Belgium; September 13–15.
- Kang Y, Agnew A, Icke K, Bolte J. 2017. Elderly PMHS thoracic responses and injuries in frontal impacts. Paper presented at: International Council on Biomechanics of Injury (IRCOBI). Antwerp, Belgium; September p. 13–15.
- Kang Y, Stammen J, Ramachandra R, Agnew AM, Hagedorn A, Thomas C, Kwon HJ, Moorhouse K, Bolte JH. 2020. Biomechanical responses and injury assessment of post mortem human subjects in various rear-facing seating configurations. Stapp Car Crash J. 64:155–212. doi: 10.4271/2020-22-0005.
- Kang Y, Stammen J, Bendig A, Agnew AM, Hagedorn A, Thomas C, Ramachandra R, Kwon HJ, Moorhouse K, Bolte JH. 2022. Effects of seatback recline and belt restraint type on PMHS responses and injuries in rear-facing frontal impacts. SAE Int. J. Trans. Safety. 10(2):235–289. doi:10.4271/09-10-02-0012.
- Kemper AR, Beeman SM, Porta DJ, Duma SM. 2016. Non-censored rib fracture data during frontal PMHS sled tests. Traffic Inj Prev. 17(sup1):131–140. doi: 10.1080/15389588.2016.1203069.
- Koppel S, Jiménez Octavio J, Bohman K, Logan D, Raphael W, Quintana Jimenez L, Lopez-Valdes F. 2019. Seating configuration and position preferences in fully automated vehicles. Traffic Inj Prev. 20(sup2):S103–S109. doi: 10.1080/15389588.2019.1625336.
- Murach MM, Kang Y, Bolte JH, Stark D, Ramachandra R, Moorhouse K, Stammen J, Agnew AM. 2018. Quantification of skeletal and soft tissue contributions to thoracic response in a dynamic frontal loading scenario. Stapp Car Crash J. 62:193–269. doi: 10.4271/2018-22-0005.
- Östling M, Larsson A. 2019. Occupant activities and sitting positions in automated vehicles in China and Sweden. Paper presented at: 26th International Technical Conference on the Enhanced Safety of Vehicles (ESV), Eindhoven, The Netherlands, June 10–13.
- Parenteau C, Viano D, Hardy W. 2021. Response corridors for blunt impacts to the back. SAE Int J Trans Saf. 9(1):123–138. doi:10.4271/09-09-01-0003.
- Reed MP, Eber SM, Jones ML. 2019. Posture and belt fit in reclined passenger Seats. Traffic Inj Prev. 20(sup1):S38–S42. doi: 10.1080/15389588.2019.1630733.
- Shurtz BK, Agnew MA, Kang Y, Bolte J. 2018. Application of scaled deflection injury criteria to two small, fragile females in side impact motor vehicle crashes. SAE Technical Paper 2018-01-0542. doi:10.4271/2018-01-0542.
- Viano DC, Hardy WN, King AI. 2001. Response of the head, neck and torso to pendulum impacts on the back. J. Crash Prev Injury Control. 2(4):289–306. doi: 10.1080/10286580108902573.
- Viano DC, Parenteau CS. 2008. Serious injury in very-low and very-high speed rear impacts. SAE Technical Paper 2008-01-1485. doi: 10.4271/2008-01-1485.
- Mertz HJ, Jarrett K, Moss S, Salloum M, Zhao Y. 2001. The Hybrid III 10-Year-Old Dummy. Stapp Car Crash J. 45:319–328. PMID: 17458751.
- Zellmer H, Lubbe N, Sander U. 2018. Assessing the injury risk of car occupants on rearward facing seats—an analysis of GIDAS cases. Paper presented at: 8th ESAR Conference, Hannover, Germany. April 19–20.