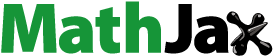
ABSTRACT
Membrane adsorbers (MA) are emerging as alternative unit operations in high-value protein separation due to their many benefits, including short residence times, low pressure drop, ease of scale-up and cost-effectiveness. Historically, the MA literature has been directed to new membrane materials and their use as a polishing step due to their low binding capacity. More recently, the emergence of membrane bind-elute modalities has extended their application at industrial scale, and a move from established resin chromatography to MA is feasible. To fill this gap and speed the development of MA, a review on the purification of monoclonal antibodies and other proteins in the pharmaceutical and food industries using commercially available MA and resin chromatography devices is presented. We compare MA with resins using three key criteria: product quality, industrial process feasibility and economic viability. In most studies, MA can produce comparable or even better product quality than resin chromatography. MA can be applied for multiple cycles within a single batch before disposal, leading to high productivity, savings from buffer consumption and the omission of cleaning verification. A cost analysis concludes that this approach can be more economical than resin chromatography.
INTRODUCTION
The COVID-19 pandemic has underscored the importance of rapid production of stable and efficacious therapeutic biomolecules. For this reason, there is an increasing focus on the optimization of the upstream bioreactor operation to facilitate both higher titer and shorter processing times[Citation1–5]. Optimization of the downstream unit operations is also essential to reduce processing times and the cost of the overall biomolecule production process. Indeed, the downstream process contributes between 50% and 90% of the total cost,[Citation1,Citation6] so any effort can yield considerable benefits.
Resin chromatography is a common purification technique seen in the downstream bioprocessing of therapeutic biomolecules. This approach relies on specific interactions of relevant species with functional groups or ligands, which are attached to polymer chains within porous spherical beads. In bind-elute mode, the target protein adsorbs to the ligands as the feed stream passes through a packed bed of the resin beads,[Citation7] while impurities are unaffected and are separated in an unbound fraction. In flow-through (FT) mode, it is the impurities that interact with the functional groups or ligands and the target protein is cleared and remains in the feed stream passing through the column.[Citation8]
The rate at which resin chromatography can proceed is limited by the slow diffusion of the relevant species into the polymer matrix of the beads. Typically, as the flow velocity increases, the dynamic binding capacity of the resins dramatically decreases and, in the case of large biomolecules, there is insufficient time for diffusion to occur within the resin pores.[Citation9,Citation10] These long residence times may result in modification of labile biomolecules through chemical degradation.[Citation11] The feed flowrate is also limited by the pressure drop through the resin bed, which can be increased through bed compression or fouling by accumulated colloidal materials.[Citation9,Citation11] Optimal purification of resin chromatography requires even flow distribution, which can be disrupted by channeling of the resin, compression resulting in head space, or fouling. Voids can also form within the packed bed structure and hence bed capacity decreases due to the uneven flow distribution.[Citation9] Typically, as the resin packing can impact on flow, regular performance qualification is required, which can be challenging and time-consuming.[Citation12] Many resins are soft and can be compressed if flow rates are too high.[Citation13] If air is inadvertently introduced to a column, the flow distribution is also compromised, and repacking is usually required. Empty columns and packing equipment are expensive and complex at large scale.
Control of processes is critical in an industrial setting, and the scale-up of resin chromatography is often a focus requiring significant attention to reproduce performance at small scale. The transport phenomena within resin beds are complex (bulk convection, film diffusion and pore diffusion), as shown in .[Citation9] These transport phenomena make optimization of a chromatographic process complex and challenging. During scale-up, the bed height and linear velocity of a column are typically kept constant. As the bed diameter increases and the wall support decreases, however, linear velocities must usually be reduced for large columns of compressible resins to avoid over-pressure. This often results in lower productivity upon scale-up.
Figure 1. An idealized comparison between packed bed chromatography and membrane chromatography for solute transport. In reality, the membrane pores are more tortuous and varied in size. Reproduced from Ghosh et al. (2002)[Citation9 with permission from Elsevier.
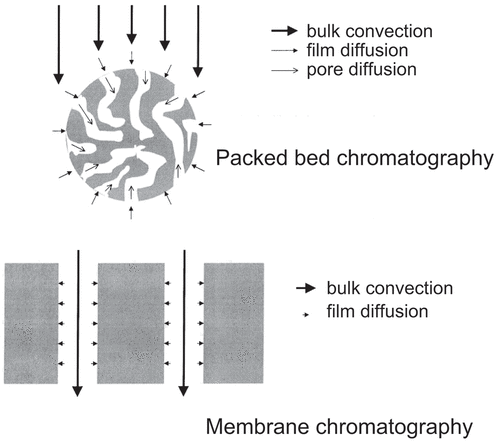
Due to these inherent limitations, much research has been performed on alternative technologies. For example, perfusive resins (e.g., POROS)[Citation7,Citation14] have larger “through pores” within the resin beads that allow for internal convective flow. Radial flow chromatography (e.g., Sepragen)[Citation15] uses radial distribution channels to allow a more uniform distribution of flow to the outer wall of a resin-filled column. Expanded bed chromatography (e.g., Streamline SP)[Citation16–19] uses an upward flow of the feed solution that causes the beads to separate and become suspended in the fluid phase. This reduces the external mass transfer resistance and ensures that cell debris is more effectively removed.
Membrane adsorbers (MA) are an alternative design. Rather than spherical beads, they consist of a mesh or mat of polymer fibers, giving a more open, micro- or macroporous structure. These fibers are functionalized with ligands similar to the resins.[Citation20] The larger pore size means that pore and film diffusion are no longer rate controlling. Convection is the main driving force for solute transport.[Citation21] The advantages of MAs over resins have been identified in the literature and can be summarized as follows:
Faster flow-rates and lower pressure drops: the binding sites are located along the flow pathway, reducing the diffusion length required to access these sites (see ).[Citation22] Since MAs are predominantly driven through convective mass transfer, faster flow rates and low pressure drop are enabled[Citation10];
Resolution is maintained at higher flow rates: MAs with minimal holdup volume inside the membrane can produce comparable resolution of biomolecules to resin chromatography under preparative conditions and at much higher volumetric flow rates[Citation23];
Reduced stress on proteins: due to faster flow rates, the contact time between biomolecules and the adsorbers is significantly reduced (e.g., minutes to seconds), increasing the potential of labile molecules, such as plasma proteins and plasmid DNA, to be preserved in their native conformation by reducing contact time with adsorbents[Citation24];
MA chromatography is easier to scale up because the flow dynamics are independent of scale[Citation25]; For example, scale up factors from 3 mL to 150 mL result in similar performance in terms of recovery and removal of impurities.[Citation26]
MAs are smaller in size because of their greater productivity and so their purchase cost is reduced.[Citation27] Single use (disposable) MA devices eliminate the cost and time associated with cleaning and validation and prevent the risk of cross-contamination between batches.[Citation28]
While these advantages are well known, the main challenge of MAs is low binding capacity. This is not a significant issue when used in flow-through applications, as only impurities bind to the membrane. Flow-through MAs, such as Sartobind® Q, have thus been commonly applied in both research[Citation29–33] and industry[Citation31,Citation32,Citation34] for many years. The use of MAs in bind-elute applications, such as affinity-based MA,[Citation6,Citation35,Citation36] is more challenging. Consequently, most of these products are still at the design phase, or only available at small scale.[Citation37–42] Only the recently released Sartobind Rapid A[Citation43] (available in sizes from 1.2 mL to 800 mL) and the GORE Protein Capture Device (available in sizes from 1 mL to 1 L) are GMP ready for commercial manufacture. Table S1 in the Supplementary Information provides a full list of MAs of varying modalities currently supplied by vendors. There is limited literature that justifies replacement of resins with commercially available MA at industrial scale, even though this has been highlighted as a critical need.[Citation42] In this review, we aim to address this need, with an assessment of the advantages and disadvantages of transitioning to this newer approach.
The choice between MA or resin chromatography in industrial applications such as monoclonal antibody (mAb) or dairy protein production can be based on three key criteria: 1) Product quality; 2) Process feasibility; and 3) Economic viability (). Product quality assesses the reduction in impurities that can be achieved within the final product while maintaining comparable protein recovery. Process feasibility considers operational parameters including dynamic binding capacity (DBC), buffer consumption, batch consistency over multiple batches and runs, chromatography skid upgrade requirements and data generation and analysis. The economic viability of MAs is favorable when there is an opportunity to streamline and optimize current downstream processing operations to minimize the overall cost.
CURRENT PROCESSES FOR PURIFYING THERAPEUTIC MACROMOLECULES
Applications of Membrane Adsorbents in Monoclonal Antibody Purification
Monoclonal antibodies (mAbs) are produced by a single clone of cells or cell lines[Citation44] and are a significant part of today’s biopharmaceutical market. In 2022, the global sales revenue for all mAb-based drugs was approximately US$ 188 billion,[Citation45] which was 70% of the total income for all biopharmaceutical products.[Citation46] In industry, mAbs are expressed into the culture media by genetically modified Chinese hamster ovary (CHO) cells. After a period of growth in the bioreactor, conditioned media containing the protein of interest are clarified to remove cells and cell debris from the bulk harvest. The purification process is governed by the characteristics of the specific mAb; however, a simplified version of the mAb purification process consists of clarification, capture, virus inactivation, polishing, and virus filtration. These steps remove impurities such as cell culture medium components, host cell proteins (HCPs) and deoxyribonucleic acid (DNA) ().[Citation47–50]
Figure 3. A typical process flow diagram for purification of mAbs, where the polishing steps indicated by the question marks may involve one of several types of chromatography.
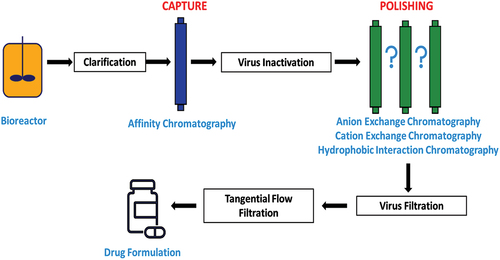
Affinity chromatography, commonly using Protein A ligands, is used in bind-elute mode to purify mAbs in what is known as the capture step.[Citation51,Citation52] After a series of washes, an elution buffer is usually used to elute the mAbs from the column. The purity of the protein after this step is normally greater than 90%.[Citation53] A viral clearance step is typically introduced to inactivate/remove adventitious viruses by holding the protein at low pH (≤3.6) for a defined duration. The intermediate process is then further purified through polishing steps, which remove residual impurities.[Citation54,Citation55] A range of chromatography modes can be applied here, such as anion exchange (AEX), cation exchange (CEX) or hydrophobic interaction (HIC). The selection of AEX or CEX is related to the molecular properties of the antibody e.g., isoelectric point (pI) and stability of the mAb. AEX is efficient in removing a variety of viruses,[Citation56] DNA[Citation57] and endotoxins.[Citation22,Citation53,Citation58] At neutral pH, impurities (viruses, DNA and HCPs) are negatively charged and strongly bind to AEX, which allows for selective binding away from mAbs, as the pI of most mAbs is above pH 7.5.[Citation8] Therefore, mAbs are purified using AEX in flow through mode.[Citation20,Citation22,Citation59,Citation60] CEX or HIC are sometimes applied after AEX to further remove protein aggregates, and the mode of operation can either be FT or bind/elute mode.[Citation20,Citation60,Citation61] In the final step of drug substance manufacturing, mAbs are further processed through a nanofiltration membrane for additional virus clearance, which is then often formulated using tangential flow filtration (TFF) to exchange buffer and concentrate the protein.
outlines a series of case studies of mAb purification using MA as alternatives to these resin chromatography operations that have been conducted over the last 30 years. Examples shown are predominantly of the polishing step. Only a limited number of papers have reported the application of MA in the capture step where the low dynamic binding capacity is of greater concern. To be economically viable, capture membranes require capacities similar to resins (>20 g L−1 bed volume). The other possible reason is that some membrane devices feature a large housing volume relative to the membrane volume, resulting in peak broadening and a low resolution if gradient elution is applied in the bind-elute mode.[Citation68–70]
Table 1. Case studies of commercial membrane adsorbents in mAb purification.
Other Applications of Membrane Adsorbents
MAs can be used to purify other biotherapeutic proteins (). Bind-elute mode is usually preferred, as the protein concentration is limited in the feed and enrichment of the product is the goal. However, it is possible to apply the flow-through mode in intermediate purification steps if the process can be optimized. For example, Nestola et al. used two FT platforms combining the Sartobind® STIC MA and core-shell CaptoCore® 700 resin to polish adenoviruses (AdVs) and virus-like particles (VLPs).[Citation76] The VLP purification achieved a recovery of 45%, while DNA and HCP reductions were 2.0 and 3.5 logs of clearance, respectively. These results are similar to other purification methods in the field of enveloped VLPs. For adenovirus purification, the recovery was almost 100%, and the DNA and HCP log reductions were 4 and 5, respectively.
Table 2. Case studies of MA used to purify other biotherapeutic proteins.
The food industry is a large user of membrane technology, typically through crossflow membrane filtration to produce protein or fruit juice concentrates. Resin chromatography is utilized for proteins of high commercial value, due to its greater specificity and selectivity. For example, lactoferrin and lactoperoxidase can be separated from skim milk or dairy whey using CEX. This is followed by ultrafiltration and diafiltration to remove salt used during elution and micro- and nano-filtration to remove bacteria and viruses.[Citation81] Approximately 20–30 tonnes of lactoferrin were produced using this approach in 2002.[Citation82] Proteins such as immunoglobulin G (IgG) have also been extracted from dairy streams with mixed mode resin as an additional step.[Citation83] Affinity or HIC chromatography is rarely used in the dairy industry, as more economical modalities can achieve the product purity and quality required in this industry. The challenges of resin chromatography in the dairy industry include long processing times (low flow rates), difficulties in scale-up and high process costs, due to complex pre- and post-processing steps,[Citation84] which could be mitigated by the use of MA. Protein concentrations in these systems are high, leading to very viscous feed streams that generate large pressure drops, which could be reduced if MA were applied.[Citation85,Citation86]
Researchers have considered MAs to replace resin chromatography in some food applications (), but it is unclear whether studies have progressed beyond the pilot scale. Both Voswinkel et al.[Citation85] and Brand et al.[Citation86] have shown that MA can achieve comparable purity and recovery to resins in such cases. Voswinkel et al. used a two-step radial flow approach to separate all the major and minor proteins in acid whey,[Citation85] AEX-MA was used in the first step to bind β-lactoglobulin and bovine serum albumin (BSA) while in the second step, the unbound fraction from the previous step was loaded onto CEX-MA to separate the minor proteins. These authors confirmed that a linear scale-up was possible with the MA approach, with a 50-fold linear scale-up producing comparable results in purity and yield. Brand et al. similarly used a radial flow membrane to separate lysozyme and ovotransferrin from egg white.[Citation86] In the first step, lysozyme was bound and eluted from the CEX-MA Sartobind® S giving a lysozyme purity of 96% and recovery of 99%. In the second step, the flow through from the first step was loaded onto Sartobind® S, and ovotransferrin was eluted with a purity of 84% and a recovery of 97%. The purity and protein recovery were independent of flow rate, which is a known advantage of the MA approach.
Table 3. Case studies of MA applied to separate molecules from food systems.
These studies show that MA has potential for large-scale application within both the pharmaceutical and food industries. However, most work to date has been conducted at small scale. To better compare the viability of MA to replace resin chromatography at large scale, these case studies are discussed with respect to product quality, process feasibility and economic viability, in the following sections.
COMPARISON OF PRODUCT QUALITY
The residual concentration of impurities such as DNA, HCPs and aggregates are critical parameters for quality control in biotherapeutic protein production. If not tightly controlled, these impurities can induce adverse events and seriously affect drug efficacy and stability.[Citation89–91]
Anion Exchange Resin Membrane Adsorbents
Numerous studies have demonstrated that AEX-MA can remove negatively charged impurities such as HCPs, viruses, DNA and endotoxins as efficiently as resin chromatography.[Citation7,Citation22,Citation26,Citation64] For instance, the contaminant clearance values by Sartobind® Q in the CAMPATH 1-H purification process are regarded as typical for resin chromatography[Citation92] (Table S2, Supporting Information). Trnovec et al. compared five different AEX-MA to comparable resins for their HCP clearance of mAb mixtures.[Citation60] Using NatriFlo® HD-Q and Sartobind® Q, a sixfold reduction of HCP was achieved, while lower reduction values were observed for the Mustang® Q even for a lower load. Gel electrophoresis demonstrated that the same bands of HCPs were removed with all membranes, so it is likely that the Mustang Q performance was due to lower binding capacity.[Citation31]
In another example, Muthukumar et al. compared two different AEX-MA against a DEAE Sepharose® resin in a mAb polishing operation.[Citation63] For the Sartobind® Q MA, pH and flow rate had a minimal impact on mAb recovery, while some operating parameters such as conductivity and loading density were more critical. In all cases, the host cell DNA was removed completely, with up to 5 kg L−1 load. Even at high flow rates, the host cell DNA was bound completely, with 94% mAb recovery. Similar results were obtained with a Sartobind® STIC MA. Both results were comparable to the DNA reduction and mAb recovery achieved in the process with the DEAE Sepharose® resin.
AEX-resin chromatography is accepted as an orthogonal tool to remove viruses, with a log reduction value (LRV) typically greater than 4.[Citation93–95] AEX can be effective over multiple runs depending on the chemical nature of the buffers, but regular cleaning to remove impurities is required. Norling et al. reported that AEX-resin chromatography when operated in FT mode did not lose viral clearance capacity even after extensive re-use when cleaned regularly with sodium hydroxide-based solutions.[Citation95] However, if cleaning was avoided, viral clearance capacity deteriorated. Typically, viral clearance cleaning processes are validated during development to ensure reproducible and effective clearances over the lifetime of the resin. The application of single-use technology to MA may reduce the need for such time-consuming validation, as cleaning processes would be redundant.
The AEX-MA Sartobind® Q showed effective virus clearance values >5 LRV even at high load[Citation92] (Table S3, Supporting Information). This performance was lower than that of the AEX-resin Q-Sepharose® Fast Flow (FF)[Citation22] (Table S4, Supporting Information). The comparison, however, was not realistic, as the MA was loaded at 40 times greater antibody load density and 9 times faster flow velocities than the resin. Virus removal was found to decrease with increasing antibody load, likely due to excess fouling of the membrane and/or saturation of available binding sites. The study recommended further testing under lower virus loads to confirm the result.
In another example, a NatriFlo® HD-Q AEX-MA was reported to remove viruses more efficiently than the equivalent resin.[Citation59] Three mAbs were considered with different isoelectric points (pIs). Under standard conditions, mAb2 and mAb3 flowed through the Sepharose® Q FF AEX resin as expected. Conversely, mAb1 was found to tightly bind to both the Sepharose® Q FF and to POROS® 50 HQ resins. This behavior could be explained by the asymmetric charge distribution on the surface of the mAb1. As illustrated in , a negatively charged cluster on the surface of a protein that is overall positively charged would orientate the protein toward the positively charged ligands on the AEX resin interface resulting in binding. In contrast, mAb1 was found to flow through the NatriFlo® HD-Q MA at the same pH, as expected from the protein pI. The authors argue that the network structure of the MA may have led to a greater “effective” electrical double-layer thickness, causing less re-orientation than the single charged interface offered by the resin. For this MA, one virus (murine leukemia virus) was not detected in the FT fraction and was only eluted in the 1000 mM sodium chloride fraction (LRV: 3.31). Trace amounts of a second virus (minute virus of mice) were found in the FT fraction at pH 7.5 but with a higher log reduction (2.26) than for the resins.
Figure 4. Explanation of the “steering effect” on an mAb with an asymmetric charge distribution. This figure was adapted from Masuda et al.[Citation59].
![Figure 4. Explanation of the “steering effect” on an mAb with an asymmetric charge distribution. This figure was adapted from Masuda et al.[Citation59].](/cms/asset/902a9257-83c2-443d-bfe9-6d86409b2c61/lspr_a_2226128_f0004_oc.jpg)
Woo et al. reported that both the ChromaSorb® and Mustang® Q MAs could achieve >4.5 LRV of DNA, while Sartobind® Q MA and Sepharose® Q Fast Flow (FF) resin achieved <1 LRV (). The early breakthrough for the Sartobind® Q MA was attributed to less ligand being available for binding, due to the reduced surface area of this device.[Citation64] Endotoxin removal was better using the MA devices than the Sepharose® Q FF resin () which showed considerably lower removal regardless of feed salt concentration. This may be a consequence of slower mass transfer in the resin, and hence less ligand contact with the endotoxin compared to the membrane.
Figure 5. Contaminant log reduction value (LRV) of ChromaSorb®, Mustang® Q and Sartobind® Q AEX-MA versus Q Sepharose® Fast Flow column (QSFF) AEX-resin chromatography.[Citation64] A) DNA LRV B) Endotoxin LRV at 0 and 250 mM NaCl. ChromaSorb® devices were loaded to 6 kg L−1, while the QSFF columns were loaded to 0.07 kg L−1. Reproduced with permission from Elsevier.
![Figure 5. Contaminant log reduction value (LRV) of ChromaSorb®, Mustang® Q and Sartobind® Q AEX-MA versus Q Sepharose® Fast Flow column (QSFF) AEX-resin chromatography.[Citation64] A) DNA LRV B) Endotoxin LRV at 0 and 250 mM NaCl. ChromaSorb® devices were loaded to 6 kg L−1, while the QSFF columns were loaded to 0.07 kg L−1. Reproduced with permission from Elsevier.](/cms/asset/4e1b110f-95b4-4f6b-8bb0-45e138865aa9/lspr_a_2226128_f0005_b.gif)
Another newly developed AEX MA device, the Emphaze® AEX Hybrid Purifier by 3 M, has demonstrated good performance in cell clarification. The highly anionic charge of this MA can capture many soluble impurities; therefore, a lower turbidity, >4 log DNA reduction and nominal 30% HCP reduction can be achieved.[Citation96–99] Almeida et al. indicated that, after clarification with Emphaze® AEX Hybrid Purifier, significantly smaller wash peaks were observed in downstream Protein A chromatography, indicating the presence of fewer contaminants.[Citation98]
Cation Exchange Resin Membrane Adsorbents
Separating aggregate impurities from mAbs can be challenging, because of their similar chemical and physical properties. CEX resins in bind-elute mode are widely used to take advantage of differing surface charges[Citation38] with salt and/or pH gradient elution used to separate the species. Historically, CEX-MAs have not been widely used in bind-elute mode to remove contaminating aggregates, due to their relatively low binding capacities,[Citation7,Citation58,Citation100] which can lead to reduced recovery.[Citation63] Liu et al. compared the performance of diffusive and perfusive CEX resins (SP-Sepharose® FF and Poros® 50HS), CEX-MA (Sartobind® S, Mustang® S) and a monolith resin (SO3 Monolith) for aggregate removal from two mAbs.[Citation7] All adsorbers were operated in bind-elute mode. Aggregate binding capacity for all MAs, but especially for Sartobind® S, was low for MAb1 (), as indicated by the more rapid breakthrough compared to the Poros® 50HS resin. For MAb2, the high molecular weight species (HMWS) bound at the start of the load cycle for all devices; however, the MA still broke through before both resins (). The difference in binding between the mAbs was attributed to the higher pI of MAb2, which induced a stronger charge interaction with the ligands. show the accumulated HMWS in each adsorbent. The MA and monolith reached their saturation binding capacity earlier than the resin, with significantly lower final values for binding capacity.
Figure 6. Removal of high molecular weight species (HMWS) by perfusive, diffusive and convective adsorbent devices.[Citation7] C/C0 is the ratio of HMWS in the eluent fraction to that in the load for a) MAb1 and b) MAb2. This data can be translated to give the accumulated HMWS bound to each adsorbent for c) MAb1 and d) MAb2. Reproduced with permission from Elsevier.
![Figure 6. Removal of high molecular weight species (HMWS) by perfusive, diffusive and convective adsorbent devices.[Citation7] C/C0 is the ratio of HMWS in the eluent fraction to that in the load for a) MAb1 and b) MAb2. This data can be translated to give the accumulated HMWS bound to each adsorbent for c) MAb1 and d) MAb2. Reproduced with permission from Elsevier.](/cms/asset/a88413f5-22cd-45e0-9e67-c79882befb04/lspr_a_2226128_f0006_b.gif)
While the binding capacity is lower in a membrane device, several studies by Madadkar et al. showed that the resolution can be considerably better.[Citation70,Citation101,Citation102] These authors used a laterally fed membrane device fabricated in-house from Sartobind S membranes. The lateral feed provides a much better flow distribution than a resin bed, as evidenced by a significant increase in the number of theoretical plates per meter. This device was able to clearly distinguish a lysozyme dimer peak from the monomer peak, which was difficult to observe in the chromatograms generated from a CEX-resin HiTrap SP HP column, even though the resin bed height was almost eight times greater than that of the membrane.[Citation101] The CEX-MA was also able to effectively separate hIgG1-CD4 charge variants with a very stable conductivity gradient, while the resin showed poorer resolution and jagged conductivity profiles due to the non-uniform flow distribution within the column.[Citation101] The MA also demonstrated good resolution in separating two whole IgG mAbs (Trastuzumab and Campath-1 H) and one heavy chain mAb (EG2-hFc) from aggregates, even when very shallow conductivity gradients were applied.[Citation102]
An alternative technique called frontal chromatography can be used to improve loading capacity. In this case, the mAb feed is applied with loading targets exceeding the normal target of the column.[Citation7,Citation38,Citation100,Citation103,Citation104] Aggregates preferentially bind to the ligands due to stronger ionic interaction, thereby displacing the weaker binding monomeric proteins. Monomeric mAbs are then collected in the unbound fraction until the aggregates are detected. The productivity for this mode is higher; however, the operating conditions for pH and conductivity must be controlled within a narrower range.[Citation100] The optimal conditions for removing aggregates may also not facilitate clearance of other impurities when frontal chromatography is used.
Fiber-based CEX-MA was tested by Winderl et al.[Citation38] in both frontal chromatography and bind-elute mode across a range of mobile-phase velocities. Initial linear salt gradient elution experiments at low load densities showed that the peak resolution of the fiber FRP S device was highest for all separations, followed by the SI-ATRP S fibers, Poros® 50 HS resin and Sartobind® S MA (). As resolution is known to vary as a function of the square root of the bed height, normalization of the data occurred by dividing by this parameter. After normalization, Sartobind® S MA gave the greatest resolution (). The higher normalized resolutions of the fiber-based and membrane devices were attributed to several factors: methacrylate in the grafted hydrogel layer leading to improved selectivity for aggregates via nonspecific hydrophobic interactions, and the improved mass transfer efficiencies for the fiber and MA when compared with the resin. In both bind-elute and frontal modes at higher load densities, the fiber-based devices met requirements for purity and yield up to very high mobile-phase velocities.
Figure 7. Comparison of the a) peak resolution and b) resolution normalized by the square root of the bed length; between the monomer and HMWS for different devices evaluated at pH 5.0.[Citation38] Reproduced with permission from Elsevier.
![Figure 7. Comparison of the a) peak resolution and b) resolution normalized by the square root of the bed length; between the monomer and HMWS for different devices evaluated at pH 5.0.[Citation38] Reproduced with permission from Elsevier.](/cms/asset/f059bba9-9ffe-48a4-9ca2-5ff4dfadad85/lspr_a_2226128_f0007_oc.jpg)
Other Chromatographic Modes
Affinity Chromatography
Affinity chromatography is a technique where ligand-specific interactions are used to preferentially separate proteins from impurities through selective binding. Performance will depend on the ligand-specific interactions; however, the matrix structure may also contribute to the specificity of this interaction. Several researchers have reported studies using affinity MAs[Citation36,Citation77] to purify biotherapeutic proteins (). Zhang et al. investigated the removal of single-arm antibody fragment impurities using MabSelect® SuRe LX and MabSelect® PrismA resins. The membrane adsorber, Fibro® PrismA was also considered, which uses a cellulose fiber matrix to enable a faster flow rate and a shorter residence time.[Citation36] MabSelect® SuRe LX was unable to differentiate between three antibody impurities and the intact antibody. MabSelect® PrismA resin was able to provide some resolution, with the mass percentage of by-product-containing fractions reducing from 29.7% to 15.9% upon buffer optimization. In contrast, the single-arm by-product was completely removed from intact antibodies when the Fibro® PrismA was used. It was argued that the MA had an enhanced resolution due to faster mass transfer during chromatography or through reduced nonspecific interaction with the matrix.
Hydrophobic Interaction Chromatography
HIC-MA can also be used in polishing operations.[Citation24,Citation63,Citation66,Citation67,Citation74,Citation75] Ribeiro et al. applied Sartobind® Phenyl MA to an intermediate purification of a recombinant protein, human factor IX (rFIX), and compared it with the current approach that uses a pseudo-affinity resin (Cellufine® Sulfate), for recovery and removal of impurities (DNA, HCP, endotoxins and activated rFIX) ().[Citation75] The recovery using the MA was lower than the process using the Sulfate resin . This study may not be a valid comparison of the MA with the resin, as two different ligands were used. However, based on these preliminary results, Sartobind® Phenyl achieved greater purity, as indicated by the ratio of rFIX activity to that in the starting material (purification factor) and the reduction in both HCP and DNA.
Table 4. Comparison between Cellufine® Sulfate and Sartobind® Phenyl[Citation75] Devices.
Table 5. Economic case studies for downstream processing.
Kuczewski et al. applied Sartobind® Phenyl as a HIC MA to polish an IgG 1-type mAb in bind-elute mode and achieved 90% recovery, 80% HCP reduction and less than 1% aggregates.[Citation66] Muthukumar et al. similarly obtained 100% monomeric mAb, using Sartobind® Phenyl MA; however, the recovery in this case was unacceptably low (8%).[Citation63] In cases where recovery was acceptable (>80%), the removal of aggregates was unacceptable (97% monomer). In contrast, current mAb industrial processes applying phenyl Sepharose® column chromatography can achieve 90% recovery with the required removal level of aggregates.
Mixed Mode Chromatography
The use of mixed mode chromatography is another option to improve clearance of aggregates[Citation106–108] when this cannot be achieved with frontal load chromatography. Improved performance may be attributed in mixed-mode to ligands having at least two different interactions, for example, ionic and hydrophobic interactions. In a recent study by Stein et al.,[Citation109] five mixed-mode ligands were attached to precursor Sartobind® membranes and compared to the Capto® Adhere resin. The membrane structure allowed only direct binding of ligands to the surface, while as an alternative, an extended 3-dimensional hydrogel was grafted to the membrane surface to form a 3D hydrogel membrane that increased binding volume. The specific ligand density was greater for the hydrogel membrane (30–80 mol m−2) than for the original membrane (10–30
mol m−2) and both considerably exceeded that of the resin (2–4
mol m−2). A reference ligand (N-Benzyl-N-methylethanolamine) used to assess binding capacity, indicated that the resin showed a tenfold greater binding capacity of both mAbs and aggregates than the 3D Hydrogel or surface membrane, due to the larger specific surface area (Resin: 34 m2 mL−1; surface membrane: 0.7–0.8 m2 mL−1; 3D hydrogen membrane: 0.7–0.8 m2 mL−1). However, the MA showed a greater selectivity toward the aggregates (ratio between binding capacity of aggregates and mAbs) than the resin, which might be related to the higher ionic capacity of the resin.
In summary, MAs produce acceptable product quality in most studies. The reduction in HCP, DNA and viruses by MA is comparable, or better, than that of resin chromatography. On the other hand, removal of aggregates by MAs at an industrial scale is still challenging due to the lower binding capacity. The use of frontal chromatography, or MA coupled with mixed mode ligands, may be options to overcome this issue.
COMPARISON OF PROCESS FEASIBILITY BETWEEN RESIN CHROMATOGRAPHY AND MEMBRANE ADSORBENTS
In this section, we focus on some common design considerations for downstream bioprocessing, such as dynamic binding capacity (DBC),[Citation110,Citation111] buffer consumption[Citation112,Citation113] and multi-use cycling performance[Citation114–116] that impact on process feasibility at an industrial scale.
Dynamic Binding Capacity
Dynamic Binding Capacity (DBC) describes the amount of target protein per unit volume (or mass) that can be loaded onto the stationary phase under operational conditions. It is impacted by the flow rate, molecular size, protein charge, ligand density, adsorbent pore diameter, mass transport efficiency and steric hindrance factors.[Citation117,Citation118]
A lower DBC than resin chromatography is achieved using MA due to a smaller number of available binding sites per unit volume and the use of a higher flow rate.[Citation7,Citation10,Citation53,Citation79,Citation84] One example from Liu et al. showed that two sulfuryl resins had higher DBC than either MA or monolith devices (Table S6, Supporting Information) but this was strongly affected by flow rate. The DBC for the resin decreased more than 50% (from 67 to 31 mg mL−1) with increasing flow rate. In contrast, the MA demonstrated a lower but stable DBC (22 mg mL−1 MV) for two different membrane scales. Winderl et al. found that while the DBC of the Poros 50 HS resin was much higher than three different MAs at low mobile-phase velocities, the value for the Fibers FRP S devices exceeded that of the Poros 50HS resin once this velocity was increased.[Citation38] Similarly, Kuczewski et al. found that a HIC-MA, Sartobind® Phenyl exhibited comparable or better DBC to resins using three different model proteins (ɤ-Globulin, ovalbumin and lysozyme), while the residence time was shorter (18 s versus 60 s, see ).[Citation26]
Figure 8. Dynamic binding capacity at 10% breakthrough for different hydrophobic interaction chromatography devices with three different model proteins (ɤ-globulin, ovalbumin, lysozyme).[Citation26] the residence time was 18 s for the Sartobind membrane and 60 s for the four other resins.
![Figure 8. Dynamic binding capacity at 10% breakthrough for different hydrophobic interaction chromatography devices with three different model proteins (ɤ-globulin, ovalbumin, lysozyme).[Citation26] the residence time was 18 s for the Sartobind membrane and 60 s for the four other resins.](/cms/asset/fb058177-253e-4403-96c2-c6e76a216526/lspr_a_2226128_f0008_oc.jpg)
Ribiero et al. showed both AEX-MA and monolith devices exhibited low DBC for pure BSA (66.5 kDa) compared with resins (Table S7, Supporting Information).[Citation79] However, this trend did not persist for an alternate protein of similar molecular weight (rFIX, 55 kDa) within a cell culture supernatant. In this case, the monolith displayed the highest DBC. It was argued that this was due to the presence of other proteins in the clarified harvest. Higher-binding proteins displaced lower binding proteins under the overloading conditions and the extent of this displacement was affected by different diffusional limitations in each device.
While the DBC is lower in an MA, all studies show that it is much more independent of flow rate, thus allowing for more stable operation.[Citation7,Citation22] The data from Boi et al.[Citation10] shown in clearly show this comparison. The ability to operate at higher flow rates with the same DBC means that the productivity of bind-elute MA can be greater than for a resin process over a complete chromatographic cycle, if the same flow rates are used during the equilibration, wash and elution steps. In the study conducted by Boi et al., the cross-sectional area of the membrane (Sartobind® Q Nano) was also greater than that of the resin bed (Q SepharoseTM Fast Flow) and this led to a greater volumetric flow rate, even when the superficial velocities were identical. The combination of both factors meant that the MA achieved 3.3 times greater maximum productivity than that achieved by the resin.[Citation10] Similarly, in a study by Vogg et al. using frontal mode CEX chromatography, a maximum productivity greater than 1000 g L−1h−1 was achieved for the MA compared to a resin at 470 g L−1 h−1 productivity.[Citation65] In this case, the MA also provided a higher yield and was less sensitive to reductions in flow rate. This in turn allows for the accommodation of an improved safety margin, giving greater process robustness.
Figure 9. Comparison of dynamic binding capacity DBC10% of BSA solutions in the membrane adsorbent Sartobind® Q Nano and resin chromatography Q SepharoseTM Fast Flow.[Citation10] Effect of flow rate on breakthrough curves for a) MA and b) a resin packed column. Comparing DBC for both the membranes and the resin packed column of c) superficial velocity and d) residence time. Reproduced with permission from Elsevier.
![Figure 9. Comparison of dynamic binding capacity DBC10% of BSA solutions in the membrane adsorbent Sartobind® Q Nano and resin chromatography Q SepharoseTM Fast Flow.[Citation10] Effect of flow rate on breakthrough curves for a) MA and b) a resin packed column. Comparing DBC for both the membranes and the resin packed column of c) superficial velocity and d) residence time. Reproduced with permission from Elsevier.](/cms/asset/0def3d96-438b-4f63-b4a7-8214339d43c1/lspr_a_2226128_f0009_oc.jpg)
All studies discussed so far only consider the DBC when operating in bind and elute mode. In FT mode, MA is expected to have a comparable capacity to resins to bind impurities. Indeed, Merck claims that the clearance capacity of DNA for NatriFlo® HD-Q MA is 27 mg mL−1, which is 20 times greater than for AEX resins.[Citation119] They argue that the greater pore size for NatriFlo® HD-Q (400 nm) compared to the resin (typically 50 nm[Citation120]) means that DNA can enter the pores more easily to interact with the ligands. Jacquemart et al. showed that the same NatriFlo® HD-Q MA gives comparable removal of HCP in FT mode to that of a HiTrap QFF AEX column.[Citation121] When combined with the increased flow capacity for MA however, they argued that productivity was significantly improved. In this case, the 5 mL HiTrap QFF column can process 250 g mAb per liter of resin with a 4 min residence time, while the 0.04 mL MA can process 20 kg mAb per liter of membrane with a 0.1 min residence time. Taking account of the different volumes of the two devices, the resin column can process 0.31 g mAb per minute, while the membrane can process 8 g mAb per minute.
Buffer Consumption
Some examples report that MAs consume less buffer than resins because of the smaller device volume required.[Citation53,Citation122,Citation123] Single-use MA can also save the buffer associated with steps such as column validation, sanitization, cleaning and storage, as these steps are not required. Zhou et al. argued that a single-use Q-MA saved up to 95% of the buffer consumption and 66% of the process time in processing a 2700 L feed volume, relative to a Q resin (Table S8, Supporting Information).[Citation53] Mora et al. similarly showed that a 1.6 L MA device can perform an equivalent mAb polishing process as a 220 L resin.[Citation92] On this basis, the MA reduced buffer consumption by over 95%, once the steps of equilibration, washing and cleaning were also included.
When MAs are re-used for multiple cycles, they can potentially consume more buffer than resin chromatography due to their lower DBC. For example, Vogg et al. indicated a buffer consumption of 0.06 L g−1 for resin chromatography and 0.10 L g−1 for MA.[Citation65] This was caused by a lower loading for the MA, which meant that more cycles were required that consumed more cleaning buffer than in a resin chromatography operation.
Our own internal trials with Fibro PrismA affinity MA suggest that buffer volumes for MA are slightly larger than for resin chromatography (). The eluate peak from MA is wider than for resins, and more buffer is consumed during washing. This difference is amplified when MA is used for a number of cycles. MA may consume more buffer when smaller MA formats are used, because a significant amount of buffer is used for washing the chromatographic skid instead of the membrane itself. In addition, a larger pump is required to provide high operational flow rates that potentially consumes more buffer than the smaller pump used for the resin process. The practicality of this consumption in commercial settings needs to be balanced with the cost of resin and MA devices, as these can be significant compared to buffer costs which are typically low. Careful consideration to optimize processes can reduce the cost of goods (COGs) during production. This impact of COGs is discussed in a later section.
Figure 10. Elution peaks generated from resin chromatography (MabSelect® PrismA: 4.7 mL) and MA (Fibro® PrismA: 0.4 mL) the elution volumes for resin chromatography and MA are 5 CV and 15 MV, respectively.
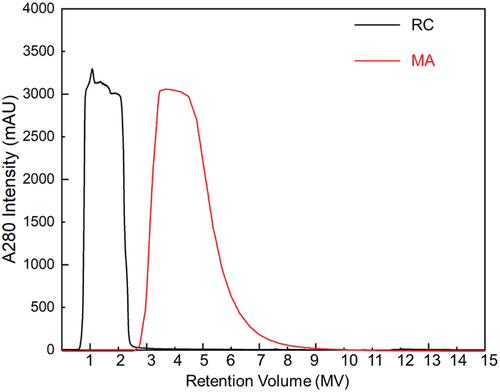
One option for further improvement is to reduce buffer consumption by continuous purification processes. Bramer et al. demonstrated the successful application of MA coupled with periodic counter-current (PCCC) chromatography in a continuous mode, which resulted in a 20% buffer saving when compared with batch runs.[Citation124]
Cycling Performance
Chromatographic resins can be used repeatedly, as long as they remain physically and chemically stable.[Citation125] After extended use, however, reductions in binding capacity and loss of protein recovery can occur. In GMP facilities during clinical batch manufacturing, resins are dedicated to a single product and often result in resin under-usage relative to their potential maximum lifetime. There are several studies investigating monitoring systems to allow such resins to be applied for multi-product re-uses. For example, the methodology described by Sharnez et al. considered three key criteria to monitor a multi-product resin re-use process: 1) system suitability parameters, such as peak shape, transition and HETP data; 2) operational parameters, such as flow rate and pressure drop across the column; and 3) process performance parameters, such as impurity profiles, product quality and recovery.[Citation126] Process capability limits are established for these parameters to ensure column integrity and binding capacity.
MAs can similarly be used for multiple cycles to purify a biomolecule from within the same cell harvest batch, although validation is required to ensure suitability and safety under GMP conditions.[Citation8] Information provided by several vendors can be used as references, but more academic studies are needed in this area to understand practicality for application in industry. For example, the affinity membrane Fibro® PrismA, developed by Cytiva®, showed consistent mAb recovery (~90%) and HCP removal (200–300 ppm) over 200 cycles.[Citation127] Less than 0.1 MPa pressure buildup was observed over 200 cycles. These results were found to be comparable to the equivalent resin chromatography process.[Citation128,Citation129] Efficient impurity removal by a protein A membrane was also found by Sartorius® over 200 cycles, with log reduction of HCPs in the range of >2.5 and for DNA of >2.[]Citation130
As in resin chromatography processes, the efficacy of clean-in-place (CIP) becomes a critical step in repeated use of MAs to prevent fouling, which would otherwise decrease the lifetime of the device. Typically, sodium hydroxide is used at an industrial scale as a cleaning and sanitizing solution, due to its effective performance in denaturing and removing proteins and clearance of viruses at low cost. The design of the CIP protocol is predicated on the tolerance of the membrane to cleaning solutions. The concentration of sodium hydroxide and contact time are the two main parameters in developing the CIP protocol, and these parameters must balance cleaning effectiveness with membrane polymer degradation and functionality. Higher concentrations of sodium hydroxide can reduce the contact time required for effective clearance but lead to longer term declines in polymer integrity.[Citation129] Manufacturers recommend different CIP strategies for different MAs, possibly reflecting the differing capacity of the underlying polymer to withstand this treatment, but commercially these strategies need to accommodate effective validated cleaning. Cytiva® recommends that the CIP be performed every cycle with 0.5 to 1 M sodium hydroxide for 1 min for Fibro® PrismA, which is a cellulosic structure. The DBC clearly declines over 100 cycles when sodium hydroxide of over 1 M is used.[Citation127] Conversely, for Sartobind® Rapid A, which is made of stabilized reinforced cellulose, Sartorius recommends 0.1-0.2 M sodium hydroxide (2 min) as the regeneration step in each cycle with a long (30 min) sanitization step optional for long-term use.Citation131 .[] Effective cleaning by CIP (0.5–1.0 M NaOH) requires not only the clearance of impurities but also the inactivation of adventitious agents[Citation132–134] that might otherwise be present during the process or upon storage of the devices.
Other Operational Considerations
Apart from the feasibility of repeated membrane use, a successful transition from resin chromatography to MA also needs to consider operational differences between the two methods of purification. As an example, the application of MAs in industry has raised concerns for chromatogram monitoring and review. During chromatographic operations, operators monitor the process and will intervene if performance is out of trend by stopping the pumps. This enables time for investigation and to address the source of the problem. There are multiple parameters to monitor, and an operator must be vigilant throughout. For membrane operation, the rate at which the automated control system steps through the phases will be much faster and it will be more challenging for an attentive operator to monitor. There could thus be a higher risk of the operator having insufficient time to manually intervene if required. There is currently a requirement for operators to review chromatograms and acknowledge they are normal prior to commencing the next cycle, and this review process could result in delays.
Advanced data analytics are useful to process big data using high-level methods and tools to observe dependencies, identify cause and effect and predict future trends. Multivariate data analytics (MVDA) is an option that effectively generates process understanding from such complex datasets with more than one variable at a time.[Citation135,Citation136] Current applications of MVDA in bioprocessing heavily focus on upstream processes.[Citation135,Citation137–139] However, recent developments in MVDA software, such as SIMCA® (Sartorius, Germany), make data analysis and prediction of downstream processes more straightforward. These programs can detect and analyze deviations from normal operating conditions through a digital twin approach.Citation140 These software packages can be beneficial to monitor MA processing cycles that generate a large amount of data. Their predictive function may also assist operators to act before production is affected.
In summary, although MAs exhibit lower DBC in most case studies, they are much less dependent on flow rate than resin chromatography, making them more robust. MAs can process protein loads in a shorter time, thus resulting in a higher productivity per hour than resin beds. The implementation of single-use MA may save time and buffer, eliminate CIP cycles and cycling validation making these processes suitable for downstream operations. MAs are also useful in multiple-cycle formats, showing consistent process output albeit potentially consuming more buffer than in resin chromatography. Recent progress in big data analysis can support the transition from resin chromatography to MA; however, there is still much further scope for development in this area.
COMPARISON OF ECONOMIC VIABILITY
The last criterion for comparison is economic viability. Cost models published to date tend to focus on the single-use format MA () and can be characterized as either batch mode or continuous in nature.
Batch Mode Cost Models
Device capacity and disposability (e.g., disposable plastics with lower costs than stainless steel) are two critical factors to consider in the calculation of unit operation costs for new bioprocesses. In two articles written by the Sartorius staff and their collaborators, the BioPharm® Services (Biopharm, UK) software package was used to predict costs for an AEX-FT mAb polishing operation at two different target loads.[Citation92] This cost model assumed:
Process lifetime of 10 years, with 400 batches over this lifetime.
Each disposable MA module was used for a single cycle giving 400 MA devices in total. The resin was used for 100 batches, so that 4 columns were used in total.
A batch size of 15 kg mAb.
Device sizes of 220 L for the resin and 1.6 L for the MA.
Costs included capital fixed charges, consumables, materials and labor.[Citation141] clearly reveals that at 10 kg L−1 target load, MA saved about 80% of the costs compared to resin chromatography. At 2 kg L−1 target load, MA saved less than 20% of these costs (). Despite increased consumable costs for the MA approach, there were significant reductions in labor and materials (buffer consumption). A large volume of resin was required due to flow rate limitations and this vast discrepancy in sizes (MA at 1.6 L versus resin chromatography at 220 L) contributed to a significant difference in capital charges.
Figure 11. Graphical results for a COG comparison of a resin and MA process of AEX-FT polishing. Costs are proportional to the total cost of the resin case. A load of a) 10 kg L−1 and c) of 2 kg L−1. c) a load of 10 kg L−1 after intermediate purification with CEX. d) a load with only initial Protein a capture. Data is from Mora et al.[Citation92] and Lim et al.[Citation28].
![Figure 11. Graphical results for a COG comparison of a resin and MA process of AEX-FT polishing. Costs are proportional to the total cost of the resin case. A load of a) 10 kg L−1 and c) of 2 kg L−1. c) a load of 10 kg L−1 after intermediate purification with CEX. d) a load with only initial Protein a capture. Data is from Mora et al.[Citation92] and Lim et al.[Citation28].](/cms/asset/0a2bcd21-1276-4acb-a3e2-c06f52216876/lspr_a_2226128_f0011_oc.jpg)
Lim et al.[Citation28] performed a similar analysis. They compared a mAb stream with a high impurity load at 2 kg mAb L−1 target load () with a stream that had undergone intermediate purification with CEX at a target load of 10 kg mAb L−1 (). For the 10 kg L−1 target load case, MA saved about 80% of costs relative to the resin. There are again increased consumable costs but significant reductions in labor and materials. There are also significant reductions in capital charges (approximately 50%) due to the elimination of the stainless steel column, including its design and installation. For the 2 kg L−1 target load case, the costs of both the MA and resin approaches are more comparable as the fraction of the cost attributed to the MA consumables increases (). The overall cost savings as a function of load is evident in .
Figure 12. Cost savings of MA capsules relative to resin columns as a function of mAb loading capacity, with data taken from Lim et al..[Citation28] The value in brackets indicates the number of MA capsules used.
![Figure 12. Cost savings of MA capsules relative to resin columns as a function of mAb loading capacity, with data taken from Lim et al..[Citation28] The value in brackets indicates the number of MA capsules used.](/cms/asset/e3a4a217-4f75-4ac2-aea6-50c4b8b971c9/lspr_a_2226128_f0012_oc.jpg)
Zhou et al. developed a similar COG model.[Citation53,Citation123] At low MA loadings (500 g mAb m−2), the resin loaded at 70 g L−1 was cheaper (). At higher MA loadings of 2000 g mAb m−2, the MA cost fell, predominantly due to the reduced number of MA units required, in addition to reduced buffer consumption. This comparison, however, did not include the costs associated with column packing and validation incurred with resin reuse. To better reflect these additional costs, the manufacturing cost, inclusive of development, manufacturing and validation was also estimated, based on a 10-year operational life. Despite the higher costs associated with the MA hardware, this approach saved 65% of the labor costs and >75% of the buffer costs, relative to a resin column. Across the ten years, MA saved approximately 22% of the costs associated with existing resin processes.
Figure 13. Cost comparison between Q Sepharose® Fast Flow and Sartobind Q Membrane for polishing 13.5 kg mAb. This graph is reproduced using data presented by Zhou et al.[Citation53].
![Figure 13. Cost comparison between Q Sepharose® Fast Flow and Sartobind Q Membrane for polishing 13.5 kg mAb. This graph is reproduced using data presented by Zhou et al.[Citation53].](/cms/asset/ebf5e119-90eb-489d-b73f-d0d33d4263bd/lspr_a_2226128_f0013_oc.jpg)
In another example, Muthukumar et al. compared the economics of AEX-, CEX- and HIC-MAs with resins in polishing 2 kg mAbs ().[Citation63] The chromatographic media for CEX- and HIC-MA are about 28 times more expensive than resins (), because the high loadings needed for bind-and-elute mode means many MA units are required. Conversely, the low concentrations of impurities absorbed during FT AEX mean that few units are required; the total cost of these units is thus five times lower than the equivalent resin process. There are similar trends in buffer consumption, with only the FT mode providing significant savings (). Conversely, the labor cost and batch times for MA are lower than the resin for both bind-elute and FT mode (). Overall, the FT nature of AEX-MA means that its total costs are considerably lower than for resin chromatography. In bind-elute mode, the costs are equivalent. Nonetheless, for CEX- and HIC-MA, the total costs are lower than resins if limited to only 10 reuse cycles.
Figure 14. Comparison of costs for resin chromatography versus membrane adsorbent. A) Cost of chromatographic media. B) Cost of buffers. C) Cost of labor and batch time. D) Overall costs.[Citation63] Reproduced with permission from John Wiley & Sons.
![Figure 14. Comparison of costs for resin chromatography versus membrane adsorbent. A) Cost of chromatographic media. B) Cost of buffers. C) Cost of labor and batch time. D) Overall costs.[Citation63] Reproduced with permission from John Wiley & Sons.](/cms/asset/1030c116-404a-4d0a-a4c8-efe462bcb7aa/lspr_a_2226128_f0014_b.gif)
In all batch mode cost models studied to date, AEX-MAs in FT mode are more economical compared with the equivalent resin process. Reductions in cost are more pronounced with increasing loading capacity because of the reduced number of MA devices required and reduced buffer consumption. Conversely, CEX- and HIC-MA cost savings are less pronounced due to more expensive media and greater buffer consumption. Indeed, CEX- and HIC-MAs are only cheaper than resin chromatography if a small batch (equal to 10 times resin use) is needed. For a manufacturing campaign requiring 100 times of resin reuse, CEX- and HIC-MA are less economical.
Continuous Processing Cost Models
Workers from Pall Corporation compared the cost of an mAb downstream purification process using resin chromatography in a fixed manufacturing suite (stainless steel vessels (SS)) to a similar batch approach using some single-use MA steps (SU) and to a continuous bioprocessing (ICB) platform where all unit operations were assumed to run in parallel.[Citation105] The calculation considered both clinical (0.1–19 kg year−1) and commercial scales (17–1600 kg year−1) of mAb production.
The study showed that the resin batch approach (SS) had the highest COG at the clinical scale. The SU batch started out with the lowest annual cost, but these costs increased more rapidly than the SS model with increasing mAb production due to the increasing device cost. The COG for the continuous (ICB) process was the lowest and the least sensitive to increasing mAb production.
Similarly, the SS batch process exhibited the highest COG at the commercial scale, with the single use (SU) batch process the second most expensive. The continuous ICB process showed the lowest COG across the whole commercial space. This can be illustrated by the commercial-scale case of 20 batches of 6000 L per year at 5 g L−1. Protein A purification and polishing are the two most costly steps. The SS batch process had the highest capital cost, while ICB was the lowest. ICB also had reduced labor costs and only suffered from increased consumable costs for the clarification step.
Processes such as the clarification and formulation step do not vary substantially when comparing SS to SU, as the hardware and consumables for these unit operations are similar. ICB costs are higher due to increased consumable use due to the greater requirement for supporting depth filter area compared with SS and SU applications. However, in both purification and polishing unit operations, infrastructure costs and resin costs result in higher cost of goods for SS. Typically, membrane sizes can be reduced in polishing steps as the operation is performed at higher flow rates. This ultimately reduces the COG for disposable systems as the chromatographic skids are smaller and there is reduced time in plant. An understanding of the optimum use of either resin columns or disposable MA in each unit operation is critical to maintaining a lowered COG. Longer process development time, high upfront costs and reduced flexibility in changing products are other factors that also need consideration.
CONCLUSIONS AND PERSPECTIVES
In this review, the advantages and challenges for the bioprocessing industry to transition from resin chromatography to MA are discussed. The key advantages of MA include:
MA can provide faster flow rates and low pressure drop, thus the productivity remains high and results in short residence time.
MA can produce comparable product quality to resin chromatography, with effective clearance of HCP, DNA, endotoxins and viruses while maintaining protein recovery.
MA can be disposable to avoid re-use validation and save buffer consumption by eliminating the steps of pre-equilibration, cleaning and storage and qualification per use. In flow-through mode, MA can save approximately 95% buffer and 66% processing time. At higher protein titers (e.g., 10 kg L−1) MA can save up to 60% of the Cost of Goods relative to resin chromatography.
MA can achieve consistent functionality over multiple cycles within a single batch. Vendor studies confirm that up to 200 cycles can be conducted using a single disposable MA, with consistent eluate attributes and yield. However, extra cleaning steps are required compared to single-use format MA. More studies are required to understand the practicality of this approach.
MA can be more economical especially when operated in a flow-through mode. A cost model also shows that the continuous process could save up to 20% buffer consumption and exhibit the lowest COGs at both clinical and commercial scale compared to either resin chromatography or MA in batch mode. Nevertheless, continuous chromatography is not yet mature at an industrial scale, due to the long process development time and low flexibility when changing products.
There are, however, limitations of MA, especially for large-scale applications where more research is required. These include:
Low dynamic binding capacity, particularly when operating in bind-elute mode. The use of mixed mode ligands has the potential to improve binding capacity but commercial scale devices are not currently available. The use of MA in frontal chromatography is another recent approach which can overcome the low productivity in bind-elute CEX-MA.
High buffer consumption per elution cycle due to the low loading density.
Poor resolution, or peak broadening, due to large housing volumes.
The need for multiple cycles in a large-scale application. This results in extensive data generation in a short period of time, which can be challenging for operators to monitor. Greater process control and online data processing will be needed for these situations.
The total cost of large-scale membrane operations remains questionable with this review showing that at lower protein concentrations (e.g., 2 kg L-1) the total cost of MA is not significantly different from resin chromatography. More extensive operational cost data is required to confirm the economics, particularly for bind-elute membrane devices.
Overall, MA chromatography shows strong potential to accelerate the development of downstream bioprocessing of therapeutic biomolecules and to significantly reduce costs, while limited studies of MA chromatography are found in food applications. While MA can replace any resin-based process, FT mode is the easiest to implement because it is less impacted by the low binding capacity and so can function with high productivity. The use of MA in bind-elute mode is more challenging but can be overcome through the use of a large number of repeat cycles with automated process control.
Abbreviations
AdVs | = | Adenoviruses |
AEX | = | Anion exchange |
BSA | = | Bovine Serum Albumin |
CEX | = | Cation exchange |
CIP | = | Cleaning-in-place |
COGs | = | Cost of goods |
CV | = | Column volume |
DBC | = | Dynamic binding capacity |
DNA | = | Deoxyribonucleic acids |
FF | = | Fast flow |
FT | = | Flow-through |
HCP | = | Host cell protein |
HETP | = | Height equivalent to the theoretical plate |
HIC | = | Hydrophobic interaction chromatography |
HMW | = | High molecular weight |
HMWS | = | High molecular weight species |
IgG | = | Immunoglobulin G |
LRV | = | Log reduction value |
MAs | = | Membrane adsorbers |
mAbs | = | Monoclonal antibodies |
MV | = | Membrane volume |
MVDA | = | Multivariate data analytics |
MW | = | Molecular weight |
PCCC | = | Periodic counter-current chromatography |
pI | = | Isoelectric point |
rFIX | = | Recombinant human factor IX |
SD | = | Standard deviation |
VLPs | = | Virus-like particles |
Acknowledgments
This research was financially supported by the Victorian High Education State Investment Fund (VHESIF). We gratefully acknowledge CSL InnovationTM for providing relevant data to estimate costs for the capture step of mAb purification.
Disclosure statement
No potential conflict of interest was reported by the author(s).
Additional information
Funding
References
- Tripathi, N. K.; Shrivastava, A. Recent Developments in Bioprocessing of Recombinant Proteins: Expression Hosts and Process Development. Front. Bioeng. Biotechnol. 2019, 7, 420–420. DOI: 10.3389/fbioe.2019.00420.
- Wilson, L. J.; Lewis, W.; Kucia-Tran, R.; Bracewell, D. G. Identification of Upstream Culture Conditions and Harvest Time Parameters That Affect Host Cell Protein Clearance. Biotechnol. Prog. 2019, 35, e2805. DOI: 10.1002/btpr.2805.
- Xu, J.; Xu, X.; Huang, C.; Angelo, J.; Oliveira, C. L.; Xu, M.; Xu, X.; Temel, D.; Ding, J.; Ghose, S., et al. Biomanufacturing Evolution from Conventional to Intensified Processes for Productivity Improvement: A Case Study. mAbs. 2020, 12, 1770669. DOI: 10.1080/19420862.2020.1770669.1.
- Gronemeyer, P.; Ditz, R.; Strube, J. Trends in Upstream and Downstream Process Development for Antibody Manufacturing. Bioeng. (Basel). 2014, 1(4), 188–212. DOI: 10.3390/bioengineering1040188.
- Kateja, N.; Tiwari, A.; Thakur, G.; Rathore, A. S. Complete or Periodic Continuity in Continuous Manufacturing Platforms for Production of Monoclonal Antibodies? Biotechnol. J. 2021, 16, 2000524. DOI: 10.1002/biot.202000524.
- Brämer, C.; Tünnermann, L.; Gonzalez Salcedo, A.; Reif, O. W.; Solle, D.; Scheper, T.; Beutel, S. Membrane Adsorber for the Fast Purification of a Monoclonal Antibody Using Protein a Chromatography. Membr. (Basel). 2019, 9(12), 120159. DOI: 10.3390/membranes9120159.
- Liu, H. F.; McCooey, B.; Duarte, T.; Myers, D. E.; Hudson, T.; Amanullah, A.; van Reis, R.; Kelley, B. D. Exploration of Overloaded Cation Exchange Chromatography for Monoclonal Antibody Purification. J. Chromatogr. A. 2011, 1218, 6943–6952.DOI: 10.1016/j.chroma.2011.08.008.
- Liu, H. F.; Ma, J.; Winter, C.; Bayer, R. Recovery and Purification Process Development for Monoclonal Antibody Production. mAbs. 2010, 2, 480–499. DOI: 10.4161/mabs.2.5.12645.
- Ghosh, R. Protein Separation Using Membrane Chromatography: Opportunities and Challenges. J. Chromatogr. A. 2002, 952(1–2), 13–27. https://doi.org/10.1016/S0021-9673(02)00057-2.
- Boi, C.; Malavasi, A.; Carbonell, R. G.; Gilleskie, G. A Direct Comparison Between Membrane Adsorber and Packed Column Chromatography Performance. J. Chromatogr. A. 2020, 1612, 460629. DOI: 10.1016/j.chroma.2019.460629.
- Harkensee, D.; Kökpinar, Ö.; Walter, J.; Kasper, C.; Beutel, S.; Reif, O.-W.; Scheper, T.; Ulber, R. Fast Screening for the Purification of Proteins Using Membrane Adsorber Technology. Eng. Life Sci. 2007, 7(4), 388–394. https://doi.org/10.1002/elsc.200720194
- Siu, S. C.; Chia, C.; Mok, Y.; Pattnaik, P. Packing of Large-Scale Chromatography Columns with Irregularly Shaped Glass Based Resins Using a Stop-Flow Method. Biotechnol. Prog. 2014, 30(6), 1319–1325. DOI: 10.1002/btpr.1962.
- Kong, D. Y.; Gerontas, S.; McCluckie, R. A.; Mewies, M.; Gruber, D.; Titchener-Hooker, N. J. Effects of Bed Compression on Protein Separation on Gel Filtration Chromatography at Bench and Pilot Scale. J. Chem. Technol. Biotechnol. 2018, 93(7), 1959–1965. DOI: 10.1002/jctb.5411.
- Wu, Y.; Abraham, D.; Carta, G. Comparison of Perfusion Media and Monoliths for Protein and Virus-Like Particle Chromatography. J. Chromatogr. A. 2016, 1447, 72–81. DOI: 10.1016/j.chroma.2016.03.077.
- Demirci, A.; Leu, F.; Bailey, F. J. Comparison of Radial and Axial Flow Chromatography for Monoclonal Antibody Downstream Processing at Bench and Pilot Scales. Am. J. Biochem. Biotechnol. 2012, 8(4), 255–262. DOI: 10.3844/ajbbsp.2012.255.262.
- Silvino Dos Santos, E.; Guirardello, R.; Teixeira Franco, T. Preparative Chromatography of Xylanase Using Expanded Bed Adsorption. J. Chromatogr. A. 2002, 944(1–2), 217–224. https://doi.org/10.1016/S0021-9673(01)01368-1.
- Shepard, S. R.; Boyd, G. A.; Schrimsher, J. L. Routine Manufacture of Recombinant Proteins Using Expanded Bed Adsorption Chromatography. Bioseparation. 2001, 10(1/3), 51–56. DOI: 10.1023/A:1012096020895.
- Kennedy, R. M. Expanded-Bed Adsorption Chromatography. Curr. Protoc. Protein Sci. 2005, 40(1), .8.8.1–.8.8.25. DOI: 10.1002/0471140864.ps0808s40.
- Lan, Q.; Bassi, A.; Zhu, J. X.; Margaritis, A. Continuous Protein Recovery from Whey Using Liquid-Solid Circulating Fluidized Bed Ion-Exchange Extraction. Biotechnol. Bioeng. 2002, 78(2), 157–163. DOI: 10.1002/bit.10171.
- Giovannoni, L.; Ventani, M.; Gottschalk, U. Antibody Purification Using Membrane Adsorbers. BioPharm. Int. 2008, 2009. https://www.biopharminternational.com/view/tools-and-technologies-for-robust-method-lifecycle-management-in-liquid-chromatography
- Dimartino, S.; Boi, C.; Sarti, G. C. A Validated Model for the Simulation of Protein Purification Through Affinity Membrane Chromatography. J. Chromatogr. A. 2011, 1218(13), 1677–1690. https://doi.org/10.1016/j.chroma.2010.11.056.
- Knudsen, H. L.; Fahrner, R. L.; Xu, Y.; Norling, L. A.; Blank, G. S. Membrane Ion-Exchange Chromatography for Process-Scale Antibody Purification. J. Chromatogr. A. 2001, 907(1–2), 145–154. DOI: 10.1016/s0021-9673(00)01041-4.
- Bhut, B. V.; Christensen, K. A.; Husson, S. M. Membrane Chromatography: Protein Purification from E. Coli Lysate Using Newly Designed and Commercial Anion-Exchange Stationary Phases. J. Chromatogr. A. 2010, 1217(30), 4946–4957. DOI: 10.1016/j.chroma.2010.05.049.
- Fan, J.; Luo, J.; Song, W.; Chen, X.; Wan, Y. Directing Membrane Chromatography to Manufacture α1-Antitrypsin from Human Plasma Fraction Iv. J. Chromatogr. A. 2015, 1423, 63–70. DOI: 10.1016/j.chroma.2015.10.050.
- Kökpinar, O.; Harkensee, D.; Kasper, C.; Scheper, T.; Zeidler, R.; Reif, O. W.; Ulber, R. Innovative Modular Membrane Adsorber System for High-Throughput Downstream Screening for Protein Purification. Biotechnol. Prog. 2006, 22(4), 1215–1219. DOI: 10.1021/bp050427f.
- Kuczewski, M.; Fraud, N.; Faber, R.; Zarbis-Papastoitsis, G. Development of a Polishing Step Using a Hydrophobic Interaction Membrane Adsorber with a PER.C6 ® -Derived Recombinant Antibody. Biotechnol. Bioeng. 2010, 105(2), 296–305. https://doi.org/10.1002/bit.22538.
- Cramer, S. M.; Holstein, M. A. Downstream Bioprocessing: Recent Advances and Future Promise. Curr. Opin. Chem. Eng. 2011, 1, 27–37. DOI: 10.1016/j.coche.2011.08.008.
- Lim, J.; Sinclair, A.; Kim, D. S.; Gottschalk, U. Economic Benefits of Single-Use Membrane Chromatography in Polishing. BioProcess. Int. 2007, 5, 60–64. https://bioprocessintl.com/downstream-processing/chromatography/economic-benefits-of-single-use-membrane-chromatography-in-polishing-20120076/.
- Kalbfuss, B.; Wolff, M.; Geisler, L.; Tappe, A.; Wickramasinghe, R.; Thom, V.; Reichl, U. Direct Capture of Influenza a Virus from Cell Culture Supernatant with Sartobind Anion-Exchange Membrane Adsorbers. J. Membr. Sci. 2007, 299, 251–260. DOI: 10.1016/j.memsci.2007.04.048.
- Vicente, T.; Sousa, M. F. Q.; Peixoto, C.; Mota, J. P. B.; Alves, P. M.; Carrondo, M. J. T. Anion-Exchange Membrane Chromatography for Purification of Rotavirus-Like Particles. J. Membr. Sci. 2008, 311(1–2), 270–283. DOI: 10.1016/j.memsci.2007.12.021.
- Weaver, J.; Husson, S. M.; Murphy, L.; Wickramasinghe, S. R. Anion Exchange Membrane Adsorbers for Flow-Through Polishing Steps: Part I. Clearance of Minute Virus of Mice. Biotechnol. Bioeng. 2013, 110(2), 491–499. https://doi.org/10.1002/bit.24720.
- Weaver, J.; Husson, S. M.; Murphy, L.; Wickramasinghe, S. R. Anion Exchange Membrane Adsorbers for Flow-Through Polishing Steps: Part Ii. Virus, Host Cell Protein, DNA Clearance, and Antibody Recovery. Biotechnol. Bioeng. 2013, 110(2), 500–510. https://doi.org/10.1002/bit.24724.
- Nascimento, A.; Rosa, S. A. S. L.; Mateus, M.; Azevedo, A. M. Polishing of Monoclonal Antibodies Streams Through Convective Flow Devices. Sep. Purif. Technol. 2014, 132, 593–600. DOI: 10.1016/j.seppur.2014.06.005.
- Clutterbuck, A.; Kenworthy, J.; Liddell, J. Endotoxin Reduction Using Disposable Membrane Adsorption Technology in cGMP Manufacturing. BioPharm. Int. 2007, 20, 40–45. <https://www.biopharminternational.com/view/separation-purification-endotoxin-reduction-using-disposable-membrane-adsorption-technology-cgmp-man>.
- Asena Özbek, M.; Çimen, D.; Bereli, N.; Denizli, A. Metal-Chelated Polyamide Hollow Fiber Membranes for Ovalbumin Purification from Egg White. J. Chromatogr. B. 2022, 1203, 123293. DOI: 10.1016/j.jchromb.2022.123293.
- Zhang, T.-M.; Wan, Y.; Wang, Y.; Li, Y. Removing a Single-Arm Species by Fibro Prisma in Purifying an Asymmetric Igg-Like Bispecific Antibody. Protein Expr. Purif. 2021, 182, 105847. DOI: 10.1016/j.pep.2021.105847.
- Guo, M.; Ye, J.; Zheng, C.; Meng, J. Dual-Recognition Membrane Adsorbers Combining Hydrophobic Charge-Induction Chromatography with Surface Imprinting via Multicomponent Reaction. J. Chromatogr. A. 2022, 1668, 462918. DOI: 10.1016/j.chroma.2022.462918.
- Winderl, J.; Neumann, E.; Hubbuch, J. Exploration of Fiber-Based Cation Exchange Adsorbents for the Removal of Monoclonal Antibody Aggregates. J. Chromatogr. A. 2021, 1654, 462451. DOI: 10.1016/j.chroma.2021.462451.
- Winderl, J.; Bürkle, S.; Hubbuch, J. High Throughput Screening of Fiber-Based Adsorbents for Material and Process Development. J. Chromatogr. A. 2021, 1653, 462387. DOI: 10.1016/j.chroma.2021.462387.
- Song, C.; Wang, M.; Liu, X.; Wang, H.; Chen, X.; Dai, L. Fabrication of High-Capacity Polyelectrolyte Brush-Grafted Porous Aao-Silica Composite Membrane via Raft Polymerization. Mater. Sci. Eng. C. 2017, 78, 748–755. DOI: 10.1016/j.msec.2017.03.042.
- Chenette, H. C. S.; Robinson, J. R.; Hobley, E.; Husson, S. M. Development of High-Productivity, Strong Cation-Exchange Adsorbers for Protein Capture by Graft Polymerization from Membranes with Different Pore Sizes. J. Membr. Sci. 2012, 423-424, 43–52. DOI: 10.1016/j.memsci.2012.07.040.
- Beuscher, U.; Kappert, E. J.; Wijmans, J. G. Membrane Research Beyond Materials Science. J. Membr. Sci. 2022, 643, 119902. DOI: 10.1016/j.memsci.2021.119902.
- SARTORIUS. Plug. Play. Change the Game. Sartobind Rapid a for mAb Capture. 2022; https://www.sartorius.com/en/products/process-chromatography/chromatography-consumables/chromatography-membranes/sartobind-rapid-a?utm_source=google&utm_medium=cpc&utm_campaign=na_en_search_Sartobind_Rapid-A&gclid=CjwKCAiA8OmdBhAgEiwAShr40xdJI6NEYh7yJ0XxR05_Dau2qxYY5x57V0uZb9oba__7imE9Gjky8RoCdHUQAvD_BwE.
- Nelson, P. N.; Reynolds, G. M.; Waldron, E. E.; Ward, E.; Giannopoulos, K.; Murray, P. G. Monoclonal Antibodies. Mol. Pathol. 2000, 53(3), 111. DOI: 10.1136/mp.53.3.111.
- Markets, R. A. Monoclonal Antibodies (Mabs) Global Market Report 2022: Increasing Prevalence of Cost-Efficient Biosimilar Monoclonal Antibodies to Drive Growth - Researchandmarkets.Com. 2022;
- Ecker, D. M.; Jones, S. D.; Levine, H. L. The Therapeutic Monoclonal Antibody Market. mAbs. 2015, 7(1), 9–14. DOI: 10.4161/19420862.2015.989042.
- Brämer, C.; Tünnermann, L.; Gonzalez Salcedo, A.; Reif, O.-W.; Solle, D.; Scheper, T.; Beutel, S. Membrane Adsorber for the Fast Purification of a Monoclonal Antibody Using Protein a Chromatography. Membranes. 2019, 9(12), 159. DOI: 10.3390/membranes9120159.
- Shukla, A. A.; Hubbard, B.; Tressel, T.; Guhan, S.; Low, D. Downstream Processing of Monoclonal Antibodies—Application of Platform Approaches. J. Chromatogr. B Analyt. Technol. Biomed. Life Sci. 2007, 848(1), 28–39. DOI: 10.1016/j.jchromb.2006.09.026.
- Shukla, A. A.; Wolfe, L. S.; Mostafa, S. S.; Norman, C. Evolving Trends in Mab Production Processes. Bioeng. Transl. Med. 2017, 2(1), 58–69. DOI: 10.1002/btm2.10061.
- Xenopoulos, A. A New, Integrated, Continuous Purification Process Template for Monoclonal Antibodies: Process Modeling and Cost of Goods Studies. J. Biotechnol. 2015, 213, 42–53. DOI: 10.1016/j.jbiotec.2015.04.020.
- Fahrner, R. L.; Blank, G. S. Real-Time Control of Antibody Loading During Protein a Affinity Chromatography Using an On-Line Assay. J. Chromatogr. A. 1999, 849(1), 191–196. DOI: 10.1016/s0021-9673(99)00539-7.
- Fahrner, R. L.; Whitney, D. H.; Vanderlaan, M.; Blank, G. S. Performance Comparison of Protein a Affinity-Chromatography Sorbents for Purifying Recombinant Monoclonal Antibodies. Biotechnol. Appl. Biochem. 1999, 30(2), 121–128. DOI: 10.1111/j.1470-8744.1999.tb00902.x.
- Zhou, J. X.; Tressel, T. Basic Concepts in Q Membrane Chromatography for Large-Scale Antibody Production. Biotechnol. Prog. 2006, 22(2), 341–349. https://doi.org/10.1021/bp050425v.
- Fahrner, R. L.; Knudsen, H. L.; Basey, C. D.; Galan, W.; Feuerhelm, D.; Vanderlaan, M.; Blank, G. S. Industrial Purification of Pharmaceutical Antibodies: Development, Operation, and Validation of Chromatography Processes. Biotechnol. Genet. Eng. Rev. 2001, 18, 301–327. DOI: 10.1080/02648725.2001.10648017.
- Fish, B. Concepts in Development of Manufacturing Strategies for Monoclonal Antibodies. 2004, pp. 1–23. DOI: 10.1007/978-1-4419-8875-1_1.
- Darling, A. Validation of Biopharmaceutical Purification Processes for Virus Clearance Evaluation. Mol. Biotechnol. 2002, 21(1), 57–83. DOI: 10.1385/MB:21:1:057.
- Hernández, L.; Rodríguez, A.; Peña, V.; Zumalacárregui, L.; Amaro, D. DNA Removal on Different Chromatography Supports Used for Epo Purification by Spike Studies. J. Liq. Chromatogr. Relat. 2016, 39(16), 762–767. DOI: 10.1080/10826076.2016.1247713.
- Petsch, D.; Anspach, F. B. Endotoxin Removal from Protein Solutions. J. Biotechnol. 2000, 76(2–3), 97–119. DOI: 10.1016/S0168-1656(99)00185-6.
- Masuda, Y.; Ogino, Y.; Yamaichi, K.; Takahashi, Y.; Nonaka, K.; Wakamatsu, K. The Prevention of an Anomalous Chromatographic Behavior and the Resulting Successful Removal of Viruses from Monoclonal Antibody with an Asymmetric Charge Distribution by Using a Membrane Adsorber in Highly Efficient, Anion-Exchange Chromatography in Flow-Through Mode. Biotechnol. Prog. 2020, 36(3), e2955. DOI: 10.1002/btpr.2955.
- Trnovec, H.; Doles, T.; Hribar, G.; Furlan, N.; Podgornik, A. Characterization of Membrane Adsorbers Used for Impurity Removal During the Continuous Purification of Monoclonal Antibodies. J. Chromatogr. A. 2020, 1609, 460518. DOI: 10.1016/j.chroma.2019.460518.
- Stein, D.; Thom, V.; Hubbuch, J. Process Development Exploiting Competitive Adsorption-Based Displacement Effects in Monoclonal Antibody Aggregate Removal—A New High-Throughput Screening Procedure for Membrane Chromatography. Biotechnol. Appl. Biochem. 2021, 69(4), 1663–1678. DOI: 10.1002/bab.2236.
- Dolan, S.; Nolan, P.; Carey, S.; Quinney, C.; Littlejohn, L. Using Anion Exchange Membrane Adsorbers to Ensure Effective Virus Clearance of Challenging Parvoviruses. BioPharm. Int. 2021, 34, 32–37. <https://www.biopharminternational.com/view/using-anion-exchange-membrane-adsorbers-to-ensure-effective-virus-clearance-of-challenging-parvoviruses>.
- Muthukumar, S.; Muralikrishnan, T.; Mendhe, R.; Rathore, A. S. Economic Benefits of Membrane Chromatography versus Packed Bed Column Purification of Therapeutic Proteins Expressed in Microbial and Mammalian Hosts. J. Chem. Technol. Biotechnol. 2017, 92(1), 59–68. https://doi.org/10.1002/jctb.5064
- Woo, M.; Khan, N. Z.; Royce, J.; Mehta, U.; Gagnon, B.; Ramaswamy, S.; Soice, N.; Morelli, M.; Cheng, K.-S. A Novel Primary Amine-Based Anion Exchange Membrane Adsorber. J. Chromatogr. A. 2011, 1218(32), 5386–5392. https://doi.org/10.1016/j.chroma.2011.03.068.
- Vogg, S.; Pfeifer, F.; Ulmer, N.; Morbidelli, M. Process Intensification by Frontal Chromatography: Performance Comparison of Resin and Membrane Adsorber for Monovalent Antibody Aggregate Removal. Biotechnol. Bioeng. 2020, 117, 662–672. DOI: 10.1002/bit.27235.
- Kuczewski, M.; Schirmer, E.; Lain, B.; Zarbis-Papastoitsis, G. A Single-Use Purification Process for the Production of a Monoclonal Antibody Produced in a Per.C6 Human Cell Line. Biotechnol. J. 2011, 6, 56–65. DOI: 10.1002/biot.201000292.
- Zarbis-Papastoitsis, G.; Kuczewski, M.; Frau, N.; Hirai, M. Hydrophobic Membrane Adsorbers for Large-Scale Downstream Processing. BioPharm. Int. 2009, 2009. Supplement. <https://www.biopharminternational.com/view/hydrophobic-membrane-adsorbers-large-scale-downstream-processing>.
- Nath, A.; Zin, M. M.; Molnár, M. A.; Bánvölgyi, S.; Gáspár, I.; Vatai, G.; Koris, A. Membrane Chromatography and Fractionation of Proteins from Whey—A Review. Processes. 2022, 10. DOI: 10.3390/pr10051025.
- Ghosh, R.; Wong, T. Effect of Module Design on the Efficiency of Membrane Chromatographic Separation Processes. J. Membr. Sci. 2006, 281, 532–540. DOI: 10.1016/j.memsci.2006.04.023.
- Madadkar, P.; Ghosh, R. High-Resolution Protein Separation Using a Laterally-Fed Membrane Chromatography Device. J. Membr. Sci. 2016, 499, 126–133. DOI: 10.1016/j.memsci.2015.10.041.
- Pereira Aguilar, P.; Reiter, K.; Wetter, V.; Steppert, P.; Maresch, D.; Ling, W. L.; Satzer, P.; Jungbauer, A. Capture and Purification of Human Immunodeficiency Virus-1 Virus-Like Particles: Convective Media Vs Porous Beads. J. Chromatogr. A. 2020, 1627, 461378. DOI: 10.1016/j.chroma.2020.461378.
- Fortuna, A. R.; van Teeffelen, S.; Ley, A.; Fischer, L. M.; Taft, F.; Genzel, Y.; Villain, L.; Wolff, M. W.; Reichl, U. Use of Sulfated Cellulose Membrane Adsorbers for Chromatographic Purification of Cell Cultured-Derived Influenza a and B Viruses. Sep. Purif. Technol. 2019, 226, 350–358. DOI: 10.1016/j.seppur.2019.05.101.
- Brämer, C.; Ekramzadeh, K.; Lammers, F.; Scheper, T.; Beutel, S. Optimization of Continuous Purification of Recombinant Patchoulol Synthase from Escherichia coli with Membrane Adsorbers. Biotechnol. Prog. 2019, 35(4), e2812. DOI: 10.1002/btpr.2812.
- Miladys Limonta, L. Z.; Rregui, U. V. N. L. K.; Urska, V.; Nika, L. K. Comparison of cim® C4-Hld Monolithic Column with Sartobind Phenyl Membrane Column for Pidke2 Purification. Chin. J. Chromatogr. 2017, 35(10), 1028–1036. DOI: 10.3724/SP.J.1123.2017.05005.
- Ribeiro, D. A.; Passos, D. F.; Ferraz, H. C.; Castilho, L. R. Intermediate Purification of Cho-Derived Recombinant Human Factor Ix Using Hydrophobic Interaction Membrane-Based Chromatography and Its Comparison to a Sulfated Resin. Electrophoresis. 2017, 38(22–23), 2900–2908. https://doi.org/10.1002/elps.201700226.
- Nestola, P.; Peixoto, C.; Villain, L.; Alves, P. M.; Carrondo, M. J. T.; Mota, J. P. B. Rational Development of Two Flow through Purification Strategies for Adenovirus Type 5 and Retro Virus-Like Particles. J. Chromatogr. A. 2015, 1426, 91–101. DOI: 10.1016/j.chroma.2015.11.037.
- Burdick, M. M.; Reynolds, N. M.; Martin, E. W.; Hawes, J. V.; Carlson, G. E.; Cuckler, C. M.; Bates, M. C.; Barthel, S. R.; Dimitroff, C. J. Isolation and Characterization of Chimeric Human Fc-Expressing Proteins Using Protein a Membrane Adsorbers and a Streamlined Workflow. J. Vis. Exp. 2014, (83). DOI: 10.3791/51023.
- Mönster, A.; Villain, L.; Scheper, T.; Beutel, S. One-Step-Purification of Penicillin G Amidase from Cell Lysate Using Ion-Exchange Membrane Adsorbers. J. Membr. Sci. 2013, 444, 359–364. DOI: 10.1016/j.memsci.2013.05.054.
- Ribeiro, D. A.; Passos, D. F.; Ferraz, H. C.; Castilho, L. R. Anion-Exchange Purification of Recombinant Factor Ix from Cell Culture Supernatant Using Different Chromatography Supports. J. Chromatogr. B. 2013, 938, 111–118. DOI: 10.1016/j.jchromb.2013.09.002.
- Suck, K.; Walter, J.; Menzel, F.; Tappe, A.; Kasper, C.; Naumann, C.; Zeidler, R.; Scheper, T. Fast and Efficient Protein Purification Using Membrane Adsorber Systems. J. Biotechnol. 2006, 121, 361–367. DOI: 10.1016/j.jbiotec.2005.07.023.
- Gokavi, S. New Technologies for Isolation and Analysis of Bioactive Compounds. In Bioactive Components in Milk and Dairy Products, Park, Y. W. Ed. Wiley, 2009; pp 329–345. https://doi.org/10.1002/9780813821504.ch14.
- Tomita, M.; Wakabayashi, H.; Yamauchi, K.; Teraguchi, S.; Hayasawa, H. Bovine Lactoferrin and Lactoferricin Derived from Milk: Production and Applications. Biochem. Cell. Biol. 2002, 80(1), 109–112. DOI: 10.1139/o01-230.
- Du, Q. Y.; Lin, D. Q.; Zhang, Q. L.; Yao, S. J. An Integrated Expanded Bed Adsorption Process for Lactoferrin and Immunoglobulin G Purification from Crude Sweet Whey. J. Chromatogr. B Analyt. Technol. Biomed. Life Sci. 2014, 947-948, 201–207. DOI: 10.1016/j.jchromb.2013.12.020.
- Kreuß, M.; Kulozik, U. Separation of Glycosylated Caseinomacropeptide at Pilot Scale Using Membrane Adsorption in Direct-Capture Mode. J. Chromatogr. A. 2009, 1216(50), 8771–8777. https://doi.org/10.1016/j.chroma.2009.01.084.
- Voswinkel, L.; Kulozik, U. Fractionation of All Major and Minor Whey Proteins with Radial Flow Membrane Adsorption Chromatography at Lab and Pilot Scale. Int. Dairy. J. 2014, 39(1), 209–214. DOI: 10.1016/j.idairyj.2014.06.012.
- Brand, J.; Dachmann, E.; Pichler, M.; Lotz, S.; Kulozik, U. A Novel Approach for Lysozyme and Ovotransferrin Fractionation from Egg White by Radial Flow Membrane Adsorption Chromatography: Impact of Product and Process Variables. Sep. Purif. Technol. 2016, 161, 44–52. DOI: 10.1016/j.seppur.2016.01.032.
- Voswinkel, L.; Etzel, M. R.; Kulozik, U. Adsorption of Beta-Lactoglobulin in Anion Exchange Membrane Chromatography versus the Contacting Mode and Temperature. LWT - Food Sci. Technol. 2017, 79, 78–83. DOI: 10.1016/j.lwt.2017.01.016.
- Saufi, S.; Fee, C. Recovery of Lactoferrin from Whey Using Cross-Flow Cation Exchange Mixed Matrix Membrane Chromatography. Sep. Purif. Technol. 2011, 77, 68–75. DOI: 10.1016/j.seppur.2010.11.021.
- Vázquez-Rey, M.; Lang, D. A. Aggregates in Monoclonal Antibody Manufacturing Processes. Biotechnol. Bioeng. 2011, 108(7), 1494–1508. https://doi.org/10.1002/bit.23155.
- Sharma, B. Immunogenicity of Therapeutic Proteins. Part 3: Impact of Manufacturing Changes. Biotechnol. Adv. 2007, 25(3), 325–331. DOI: 10.1016/j.biotechadv.2007.01.007.
- Wang, W.; Nema, S.; Teagarden, D. Protein Aggregation—Pathways and Influencing Factors. Int. J. Pharm. 2010, 390, 89–99. DOI: 10.1016/j.ijpharm.2010.02.025.
- Mora, J.; Sinclair, A.; Delmdahl, N.; Gottschalk, U. Downstream Disposable Membrane Chromatography Performance Analysis and Economic Cost Model. Bio Process International 2006, 4, 38–43.
- Strauss, D. M.; Gorrell, J.; Plancarte, M.; Blank, G. S.; Chen, Q.; Yang, B. Anion Exchange Chromatography Provides a Robust, Predictable Process to Ensure Viral Safety of Biotechnology Products. Biotechnol. Bioeng. 2009, 102(1), 168–175. https://doi.org/10.1002/bit.22051.
- Curtis, S.; Lee, K.; Blank, G. S.; Brorson, K.; Xu, Y. Generic/Matrix Evaluation of sv40 Clearance by Anion Exchange Chromatography in Flow-Through Mode. Biotechnol. Bioeng. 2003, 84(2), 179–186. https://doi.org/10.1002/bit.10746.
- Norling, L.; Lute, S.; Emery, R.; Khuu, W.; Voisard, M.; Xu, Y.; Chen, Q.; Blank, G.; Brorson, K. Impact of Multiple Re-Use of Anion-Exchange Chromatography Media on Virus Removal. J. Chromatogr. A. 2005, 1069(1), 79–89. https://doi.org/10.1016/j.chroma.2004.09.072.
- 3M. 3m™ emphaze™ Aex Hybrid Purifier. 2023; <https://www.3m.com/3M/en_US/p/d/b40066769/>.
- Koehler, K. C.; Jokondo, Z.; Narayan, J.; Voloshin, A. M.; Castro-Forero, A. A. Enhancing Protein a Performance in Mab Processing: A Method to Reduce and Rapidly Evaluate Host Cell DNA Levels During Primary Clarification. Biotechnol. Prog. 2019, 35(6), e2882. https://doi.org/10.1002/btpr.2882.
- Almeida, A.; Chau, D.; Coolidge, T.; El-Sabbahy, H.; Hager, S.; Jose, K.; Nakamura, M.; Voloshin, A. Chromatographic Capture of Cells to Achieve Single Stage Clarification in Recombinant Protein Purification. Biotechnol. Prog. 2022, 38(2), e3227. DOI: 10.1002/btpr.3227.
- Gilgunn, S.; El-Sabbahy, H.; Albrecht, S.; Gaikwad, M.; Corrigan, K.; Deakin, L.; Jellum, G.; Bones, J. Identification and Tracking of Problematic Host Cell Proteins Removed by a Synthetic, Highly Functionalized Nonwoven Media in Downstream Bioprocessing of Monoclonal Antibodies. J. Chromatogr. A. 2019, 1595, 28–38. DOI: 10.1016/j.chroma.2019.02.056.
- Reck, J. M.; Pabst, T. M.; Hunter, A. K.; Carta, G. Separation of Antibody Monomer-Dimer Mixtures by Frontal Analysis. J. Chromatogr. A. 2017, 1500, 96–104. DOI: 10.1016/j.chroma.2017.04.014.
- Madadkar, P.; Sadavarte, R.; Ghosh, R. Performance Comparison of a Laterally-Fed Membrane Chromatography (Lfmc) Device with a Commercial Resin Packed Column. Membr. (Basel). 2019, 9(11), 9. DOI: 10.3390/membranes9110138.
- Madadkar, P.; Sadavarte, R.; Butler, M.; Durocher, Y.; Ghosh, R. Preparative Separation of Monoclonal Antibody Aggregates by Cation-Exchange Laterally-Fed Membrane Chromatography. J. Chromatogr. B. 2017, 1055-1056, 158–164. DOI: 10.1016/j.jchromb.2017.04.036.
- Suda, E. J.; Thomas, K. E.; Pabst, T. M.; Mensah, P.; Ramasubramanyan, N.; Gustafson, M. E.; Hunter, A. K. Comparison of Agarose and Dextran-Grafted Agarose Strong Ion Exchangers for the Separation of Protein Aggregates. J. Chromatogr. A. 2009, 1216(27), 5256–5264. https://doi.org/10.1016/j.chroma.2009.05.021.
- Stone, M. T.; Cotoni, K. A.; Stoner, J. L. Cation Exchange Frontal Chromatography for the Removal of Monoclonal Antibody Aggregates. J. Chromatogr. A. 2019, 1599, 152–160. DOI: 10.1016/j.chroma.2019.04.020.
- Hummel, J.; Pagkaliwangan, M.; Gjoka, X.; Davidovits, T.; Stock, R.; Ransohoff, T.; Gantier, R.; Schofield, M. Modeling the Downstream Processing of Monoclonal Antibodies Reveals Cost Advantages for Continuous Methods for a Broad Range of Manufacturing Scales. Biotechnol. J. 2019, 14(2), 1700665. https://doi.org/10.1002/biot.201700665.
- Wang, J.; Jenkins, E. W.; Robinson, J. R.; Wilson, A.; Husson, S. M. A New Multimodal Membrane Adsorber for Monoclonal Antibody Purifications. J. Membr. Sci. 2015, 492, 137–146. DOI: 10.1016/j.memsci.2015.05.013.
- Voitl, A.; Müller-Späth, T.; Morbidelli, M. Application of Mixed Mode Resins for the Purification of Antibodies. J. Chromatogr. A. 2010, 1217(37), 5753–5760. DOI: 10.1016/j.chroma.2010.06.047.
- Toueille, M.; Uzel, A.; Depoisier, J. F.; Gantier, R. Designing New Monoclonal Antibody Purification Processes Using Mixed-Mode Chromatography Sorbents. J. Chromatogr. B Analyt. Technol. Biomed. Life Sci. 2011, 879, 836–843. DOI: 10.1016/j.jchromb.2011.02.047.
- Stein, D.; Thom, V.; Hubbuch, J. Streamlined Process Development Procedure Incorporating the Selection of Various Stationary Phase Types Established in a Mab Aggregate Reduction Study with Different Mixed Mode Ligands. Biotechnol. Prog. 2022, 38(2), e3230. DOI: 10.1002/btpr.3230.
- Fahrner, R. L.; Iyer, H. V.; Blank, G. S. The Optimal Flow Rate and Column Length for Maximum Production Rate of Protein a Affinity Chromatography. Bioprocess. Eng. 1999, 21, 287–292. DOI: 10.1007/s004490050677.
- Kramberger, P.; Urbas, L.; Štrancar, A. Downstream Processing and Chromatography Based Analytical Methods for Production of Vaccines, Gene Therapy Vectors, and Bacteriophages. Hum. Vaccin. Immunother. 2015, 11, 1010–1021. DOI: 10.1080/21645515.2015.1009817.
- Cytiva. How Effective Buffer Management Facilitates Biopharmaceutical Production. Genetic Eng. Biotechnol. News. 2019. <https://www.genengnews.com/sponsored/how-effective-buffer-management-facilitates-biopharmaceutical-production/>.
- Haigney, S. The Importance of Buffers in Downstream Processing. BioPharm. Int. 2016, 29, 26–29. <https://www.biopharminternational.com/view/importance-buffers-downstream-processing>.
- Behere, K.; Cha, B.; Yoon, S. Protein a Resin Lifetime Study: Evaluation of Protein a Resin Performance with a Model-Based Approach in Continuous Capture. Prep. Biochem. Biotechnol. 2018, 48, 242–256. DOI: 10.1080/10826068.2018.1425711.
- Hardick, O.; Dods, S.; Stevens, B.; Bracewell, D. G. Nanofiber Adsorbents for High Productivity Downstream Processing. Biotechnol. Bioeng. 2013, 110(4), 1119–1128. https://doi.org/10.1002/bit.24765.
- Contributor, B. Establishing Resin Lifetime. BioProcess. Int. 2003. <https://bioprocessintl.com/downstream-processing/chromatography/establishing-resin-lifetime-1120039/>.
- Harinarayan, C.; Mueller, J.; Ljunglöf, A.; Fahrner, R.; Van Alstine, J.; van Reis, R. An Exclusion Mechanism in Ion Exchange Chromatography. Biotechnol. Bioeng. 2006, 95(5), 775–787. DOI: https://doi.org/10.1002/bit.21080.
- Hahn, R.; Deinhofer, K.; Machold, C.; Jungbauer, A. Hydrophobic Interaction Chromatography of Proteins: Ii. Binding Capacity, Recovery and Mass Transfer Properties. J. Chromatogr. B. 2003, 790(1–2), 99–114. https://doi.org/10.1016/S1570-0232(03)00080-1.
- Merck. Comparison of Process-Related Impurity Clearance for Membrane Adsorbers and Resin-Based Chromatography Technologies. 2022; https://www.sigmaaldrich.com/AU/en/technical-documents/technical-article/pharmaceutical-and-biopharmaceutical-manufacturing/monoclonal-antibody-manufacturing/membrane-chromatography-vs-resin-chromatography#DNA-Binding-Capacity-Study.
- Nweke, M. C.; McCartney, R. G.; Bracewell, D. G. Mechanical Characterisation of Agarose-Based Chromatography Resins for Biopharmaceutical Manufacture. J. Chromatogr. A. 2017, 1530, 129–137. DOI: 10.1016/j.chroma.2017.11.038.
- Jacquemart, R.; Stout, J. Membrane Adsorbers, Columns: Single-Use Alternatives to Resin Chromatography. BioProcess. Int. 2017. <https://bioprocessintl.com/downstream-processing/filtration/membrane-adsorbers-columns-single-use-alternatives-resin-chromatography/>.
- Orr, V.; Zhong, L.; Moo-Young, M.; Chou, C. P. Recent Advances in Bioprocessing Application of Membrane Chromatography. Biotechnol. Adv. 2013, 31, 450–465. DOI: 10.1016/j.biotechadv.2013.01.007.
- Gottschalk, U. Disposables in Downstream Processing. In Disposable Bioreactors, Regine Eibl & Dieter Eibl Eds. Heidelberg, Germany: Springer Berlin, 2009; pp 171–183. DOI: 10.1007/s00253-009-2422-9.
- Brämer, C.; Schreiber, S.; Scheper, T.; Beutel, S. Continuous Purification of Candida Antarctica Lipase B Using 3-Membrane Adsorber Periodic Counter-Current Chromatography. Eng. Life Sci. 2018, 18(7), 414–424. DOI: 10.1002/elsc.201700159.
- Bracewell, D. G.; Pathak, M.; Ma, G.; Rathore, A. S. Re-Use of Protein a Resin: Fouling and Economics. BioPharm. Int. 2015, 28. <https://www.biopharminternational.com/view/re-use-protein-resin-fouling-and-economics>
- Sharnez, R.; Doares, S.; Manning, S.; Mehta, K.; Mahajan, E.; To, A.; Daniels, W.; Glynn, J.; Dhamane, S.; Wen, X., et al. Multiproduct Resin Reuse for Clinical and Commercial Manufacturing—Methodology and Acceptance Criteria. PDA J. Pharm. Sci. Technol. 2018, 72, 584. DOI: 10.5731/pdajpst.2016.007245.6.
- Cytiva. Hitrap Fibro Prisma Unit Hiscreen Fibro Prisma Units. 2021; https://cdn.cytivalifesciences.com/api/public/content/digi-33339-pdf.
- Cytiva. Evaluation of Protein a Resin Lifetime During Extensive Use (Overloading) in Continuous Chromatography Mode. 2020; http://www.processdevelopmentforum.com/ppts/posters/Protein-A-lifetime-overloading-chromatography-CY13921-27May20-AN.pdf.
- Cytiva. Efficient Cleaning-In-Place Methods for Protein-Based Antibody Affinity Chromatography Resins. 2020; https://cdn.cytivalifesciences.com/api/public/content/digi-24266-original.
- Busse, R. A.; Gruenberg, M.; Kuchemueller, K. B.; Toeppner, K.; Adametz, P.; Thom, V. Sartobind Rapid A: Comparable High Product Quality as Protein A Resins at High Productivity. 2022. https://www.sartorius.com/download/1289864/sartobind-rapid-a-comparability-to-resin-application-note-en-1--data.pdf.
- SARTORIUS. Instructions for Use-Sartobind Rapid A. 2022. https://www.sartorius.com/shop/medias/Sartobind-Rapid-A.pdf?context=bWFzdGVyfGRvY3VtZW50c3wxMzg4NzU0fGFwcGxpY2F0aW9uL3BkZnxkb2N1bWVudHMvaGIyL2hhNS85MTk4MzQyMjA5NTY2LnBkZnxjYmUxM2RmNzE2YzlmMTg3YjJmMTZhYTdhMzJlNTU4NmU1ZWUxNTZhMGMzODg5ZTllMTYyMWE5ZWQ3NzgwMTg5
- Cytiva. Use of Sodium Hydroxide for Cleaning and Sanitization of Chromatography Resins and Systems. 2020; https://cdn.cytivalifesciences.com/api/public/content/digi-20986-original#:~:text=Sodium%20hydroxide%20is%20widely%20accepted,detection%2C%20removal%2C%20and%20disposal.
- Boschetti, N.; Wyss, K.; Mischler, A.; Hostettler, T.; Kempf, C. Stability of Minute Virus of Mice Against Temperature and Sodium Hydroxide. Biologicals. 2003, 31(3), 181–185. https://doi.org/10.1016/S1045-1056(03)00037-X.
- Grönberg, A.; Hjorth, R. A. Cleaning-in-Place and Santization. In Biopharmaceutical Processing, Günter Jagschies, Eva Lindskog, Karol Łącki, & Parrish Galliher, Eds. Elsevier: United Kingdom. 2018; pp 675–699. DOI: 10.1016/B978-0-08-100623-8.00033-5.
- Rathore, A. S.; Mittal, S.; Pathak, M.; Arora, A. Guidance for Performing Multivariate Data Analysis of Bioprocessing Data: Pitfalls and Recommendations. Biotechnol. Prog. 2014, 30(4), 967–973. DOI: 10.1002/btpr.1922.
- Bernard, J.; Hutter, M.; Reinemuth, H.; Pfeifer, H.; Bors, C.; Kohlhammer, J. Visual-Interactive Preprocessing of Multivariate Time Series Data. Comput. Graph. Forum. 2019, 38(3), 401–412. DOI: 10.1111/cgf.13698.
- Singh, S. K.; Rathore, A. S. Use of Multivariate Data Analysis in Bioprocessing. BioPharm. Int. 2015, 28. <https://www.biopharminternational.com/view/use-multivariate-data-analysis-bioprocessing>
- Banner, M.; Alosert, H.; Spencer, C.; Cheeks, M.; Farid, S. S.; Thomas, M.; Goldrick, S. A Decade in Review: Use of Data Analytics within the Biopharmaceutical Sector. Curr. Opin. Chem. Eng. 2021, 34, 100758. DOI: 10.1016/j.coche.2021.100758.
- Dahotre, S.; Dai, L.; Kjenstad, K.; Stella, C.; Camperi, J. Real-Time Monitoring of Antibody Quality Attributes for Cell Culture Production Processes in Bioreactors via Integration of an Automated Sampling Technology with Multi-Dimensional Liquid Chromatography Mass Spectrometry. J. Chromatogr. A. 2022, 1672, 463067. DOI: 10.1016/j.chroma.2022.463067.
- SARTORIUS. Digital Twins Offer a Data-Based Approach to More Effective Biopharma Process Control. https://www.sartorius.com/en/knowledge/science-snippets/digital-twins-offer-a-data-based-approach-to-more-effective-biopharma-process-control-602752. (accessed June25, 2023).
- Sinclair, A.; Monge, M. Quantitative Economic Evaluation of Single Use Disposables in Bioprocessing. Pharm. Eng. 2002, 22, 20–34. https://www.researchgate.net/publication/279703195.