ABSTRACT
This paper presents a novel plant-derived core-shell fiber carrier that can be used in self-healing applications. The study used abaca fiber lumens as a self-healing carrier for the first time. The fibers were characterized under SEM at different positions to know the lumen structure and achieved a 39.19% lumens fraction and 16.13 µm lumen diameter. Healing resins were embedded in the lumens using modified VARTM. The core was confirmed using FESEM-EDX. Thermal degradation was determined via TGA under a nitrogen atmosphere. The mechanical and self-healing properties, as well as a single-fiber tensile test, were conducted with a cell load of 5 kgf. The healing resin (VE-CN)-embedded fibers showed a strength improvement of 14.78% compared with the strength of pure abaca fibers. The improved strength is attributed to the reorientation of the crystalline cellulose in the tensile direction. Additionally, some of the tensile-fractured fibrils self-healed after 24 h and showed a restored strength of 100.71 MPa. The restored strength explains that the fiber lumens are the potential to carry a healing resin and release upon the damage.
摘要
本文介绍了一种新型植物源核壳纤维载体,可用于自愈应用.这项研究首次将阿巴卡纤维管腔用作自愈载体.在不同位置的SEM下对纤维进行表征,以了解管腔结构.使用改良的VARTM将愈合树脂嵌入管腔中. 使用FESEM-EDX确认岩芯.在氮气氛下通过TGA测定热降解.机械和自愈性能以及单纤维拉伸试验在电池负载为5kgf的情况下进行.愈合树脂(VE-CN)包埋纤维的强度比纯abaca纤维的强度提高了14.78%.提高的强度归因于结晶纤维素在拉伸方向上的重新定向.此外,一些拉伸断裂的原纤维在24小时后自行愈合,并显示出100.71MPa的恢复强度.恢复的强度说明纤维管腔有可能携带愈合树脂并在损伤时释放.
Introduction
In recent years, the development of self-healing structures has garnered significant attention in various fields of application. In mechanical engineering applications, polymer composite (PC) structures are susceptible to damage due to external stimuli, which create and propagate cracks until complete damage to the structures occurs (Zhao et al. Citation2021, Wang et al. Citation2022). The initiated crack leads to a severe rehabilitation challenge requiring significant time, money, and workforce. To prevent crack propagation and extend the lifespan of structures, different approaches have been used to achieve self-healing properties in materials (Naga Kumar, Prabhakar, and Song Citation2020). The development of multifunctional self-healing carriers, such as hydrogels (Swamy et al. Citation2013), microspheres (Venkata Chalapathi, Kumar Cheedarala, and Il Song Citation2021), and multiscale vascular network systems (Zhu et al. Citation2021), plays a vital role in the manufacturing of self-healing composites.
The self-healing property mainly depends on the intrinsic and extrinsic triggering mechanisms. The intrinsic self-healing mode mainly dependent on the supramolecular interactions of covalent bonds (Yong-Chao, Shao, and Zhan Citation2021, Sajin et al. Citation2022). However, the extrinsic self-healing mechanism is based on microencapsulation and hollow vascular network systems (Fidelis, Vitorino Castro Pereira, and Dias Toledo Filho Citation2013, Peng et al. Citation2021, Naga Kumar, Prabhakar, and Song Citation2021). Self-healing vascular network systems have been reported to exhibit high healing efficiencies and are capable of carrying out multiple healing cycles (Chalapathi, Venkata, and Il Song Citation2022). The main advantage of vascular carriers relies on capillary action to release the healing liquids into the obstructed zones. The dispersion of vascular systems in PCs is due to a high degree of rehabilitation when they experience external damage or environmental hazards. Owing to the brittle nature of vascular systems, they have a rapid healing response when they crack at the microscale. However, the vascular carriers’ diameter and the healing polymers’ surface tension also determine the self-healing rate.
In recent years, plant-derivednatural fibers have been widely used as adequate reinforcements in PCs because of their specific properties, such as being lightweight, inexpensive, biodegradable, and high specific strength (Hu et al. Citation2022, Kukde, Ketan Sarangi, and Purohit Citation2019, Garg and Jain Citation2022). Plant fibers mainly possess a vascular lumen network in their structure, which is a good candidate for thermal insulation (Bernal-Chávez et al. Citation2022) and acoustic absorbents (Yan et al. Citation2015). Additionally, each lumen in the plant fiber consists of a thin xylem layer, ensuring that fluids flow in the appropriate direction. Therefore, the structure of the lumen is one of the most significant factors in plants that controls the transfer of food and minerals from the roots to the leaves to facilitate photosynthesis. The lumen structure comprises a cell wall or lamella that separates neighboring lumens. Technical unidirectional abaca fiber lumens have unique features in that they act as transverse thermal conductivity reinforcements rather than crystalline structures in PCs (Wang et al. Citation2021).
Zhang, Zhu, and Zhong (Citation2022) proposed a progressive micro-modeling technique to study the microstructure of unidirectional abaca fibers, including the lumens and unit cells composed of fiber bundles. A representative volume element model was established for the composite to study the properties of the lumens of empty fibers. Finally, the authors used the black box program to determine the effective thermal coefficient for various plant fiber volume fractions. Li et al. (Zhao et al. Citation2021) studied the mechanical performance of sisal fiber-reinforced epoxy-based composites and reported that the resin inside the sisal fiber lumens greatly influenced the mechanical strength. However, there was no significant effect on the tensile or flexural moduli. The acoustic emission, crack bridging effect, and bonding between the microfibrils was also studied to determine the performance of the composites with sisal fibers. It was concluded from the above literature that fiber vascular lumens are potentially suitable self-healing carriers in polymer-based composites. Incorporating of synthetic (glass) vascular systems in PCs as self-healing carriers has been explored to resolve fiber-matrix interaction problems. The reinforcement of synthetic carriers between fibers has created an uneven composite surface; additionally, it has a poor interaction with the natural fibers owing to the hydrophobic surface of the carriers (Chuanwei et al. Citation2022, Moreira and Marques Citation2022). Synthetic carriers are unsuitable for multiple healing cycles because of their fragile nature. The manufacturing of thermoplastic 3D-printed vascular systems for self-healing applications is also a great challenge, and the healing mechanism of the vascular network system is mainly dependent on carrier's physical and mechanical characteristics (Heng et al. Citation2021). Further development of healing carriers is essential in overcoming the drawbacks of existing carriers, such as high cost, complex process, significant interaction issues, and poor mechanical properties.
Therefore, the present study is the first to introduce natural fiber lumens as self-healing carriers, which can effectively replace existing carriers and overcome the above-mentioned drawbacks. Moreover, natural fiber lumens serve as a reinforcement for PCs and carriers; hence, their interaction would be more accessible, and they would become biodegradable. This study, considered unidirectional abaca fibers, and their physical properties and structural integrity were evaluated for self-healing purposes. A complete study was conducted using scanning electron microscopy (SEM) analysis to determine the properties of the fibers at various locations. SEM analysis revealed that the abaca fibers have a vascular lumen structure through the length of the fiber, which are good candidates for carrying healing liquids. Furthermore, the healing resin was infused using vacuum-assisted resin infusion molding (VARIM), considering a different experimental setup. After the infusion, the healing liquids inside the fiber lumens were confirmed using SEM and field-emission SEM coupled with energy dispersive X-ray (FESEM-EDX) spectroscopy mapping. In addition, the thermal degradation of the fiber and polymers was studied using thermogravimetric analysis (TGA). Finally, a single-fiber tensile test was performed to evaluate the mechanical properties of the fibers with and without a core. Processed abaca fiber lumens play a crucial role in the self-healing of PCs and as substitutes for synthetic vascular materials.
Materials and methods
Materials
The unidirectional abaca fibers (density and average diameter are 1.5 g/cm3 and 0.39 mm, respectively) were obtained from Soo Industries Co., South Korea. Vinyl ester (KRF-1031, viscosity: 150 cps, specific gravity: 1.03), cobalt naphthalate (CN) promoter, and methyl ethyl ketone peroxide (MEKP-BUTANOX M-60) catalyst were purchased from Kukdo Chemicals, Korea. Double-sided sealant tape was purchased from General Sealants, Inc. (JET Korea). Silicone rubber adhesive (Sil-Poxy) was obtained from Smooth – On, Inc., Korea.
Processing of fibers and resin infusion
The cluster unidirectional abaca fibers were washed and straightened in water for 30 min and dried in an oven at 105°C for 2 h. Well-arranged, hackled fibers were cut to the desired length. The entire fiber process is shown in . The resin infusion process involves a unique setup to infuse the healing resin into each lumen, as shown in . In this process, a rubber adhesive was first selected, then the fibers were merged in 10-mm before the inlet of the fibers. The applied rubber adhesive was firmly fixed at the inlet of each fiber to create a circular resin stopper after drying. This resin stopper is useful for preventing the resin from passing on fiber’s surface, ensuring that the resin is only located inside the fiber lumens. Double-sided sealant tape was used before and after the resin stopper at the top and bottom sides of the fibers. The prepared fibers were kept in a pre-prepared rectangular aluminum mold covered with double-sided sealant tape. The entire setup was covered with a vacuum bag to create a vacuum inside the mold. After placing the vacuum bag tightly on the mold, a pressure of 0.04 MPa was applied using an air compressor, and any leakage from the setup was observed for 1 min. Finally, cobalt naphthalate-diluted vinyl ester and MEKP were separately infused through the inlet pipe until the resin entered the outlet pipe.
Tests and characterization
The morphology of the fibers was studied using SEM (Emcrafts Cube 2, Korea) at 20 kV, and the chemical composition of the fibers was determined using FESEM-EDX (TESCAN, LYRA3XM, Czech Republic) within the range of 5–30 kV. The thermal decomposition of the fibers was evaluated using a thermogravimetric analyzer (TGA; Perkin Elmer STA 6000). The sample mass ranged from 10 to 12 mg, and the temperature was increased from 30 to 700°C, at a rate of 20 °C/min, under a nitrogen atmosphere. A single-fiber tensile test was performed on the unidirectional fibers with a micro-load (model: UU-K5, capacity: 5 kgf, cell load: Dacell, Korea), then emery paper strips were used to grip the fiber to minimize slippage during the tests. All tested fibers failed at the center of the fiber, and only the average strength of the five specimens was reported.
Results and discussion
Physical properties
The physical properties of the fibers are essential parameters for estimating their strength and lifespan. According to the literature, the density of abaca fibers is approximately 1.5 g/cm3. The mass of each fiber was determined by weighing 100 random fibers simultaneously, each with a length of 300 mm, and then taking an average. As a result, the average mass of a single fiber is approximately 0.025 g. After resin infusion, the mass of the fibers was re-calculated to determine the amount of resin inside each fiber. Therefore, the selected 100 fibers were first subjected to resin infusion. Thereafter, the resin-infused fibers were weighed, and the calculated average mass of the resin inside a single fiber was approximately 0.18 g. The calculation shows that the average mass of each fiber increases from 0.025 to 0.205 g after the resin infusion.
Structural features of abaca lumens
Most of the abaca fibers have a circular cross-section; however, some have an elliptical cross-section. The fibers consist of many lumens depending on the diameter of each fiber. The diameter of the abaca fibers was measured using SEM, which is 397 µm on average. shows a single fiber’s cross-section with the exposed lumen network system. It can be observed that each lumen is connected to another with the support of a protective cell wall or lamella (Yan et al. Citation2015, Selvan et al. Citation2022). A middle lamella binds each cell to its neighbors, and the primary and secondary walls compose the majority of the walls in abaca fiber. Primary cell walls have a role in controlling cell growth and promoting cell adhesion, whereas secondary cell walls provide cells mechanical strength. The secondary cell wall is composed of more cellulose content with a linear chain of at least 500 glucose residues that are covalently linked to one another to form ribbon-like structures, which are more stabilized by hydrogen bonds within the chain. Using Eq. 1, the average area of each abaca fiber was calculated based on 10 fibers, and the average value is approximately 0.124 mm2. clearly shows that the vascular lumen structure contains a thick layer of xylem inside each lumen, which protects and facilitates the transport of water from the roots to the leaves to promote photosynthesis (Jun-Feng et al. Citation2019). In plant fibers, the primary function of the xylem is to store food in the form of starch/fat and other substances. The typical xylem cell wall composes of lignin, which consists of tracheids, sclerenchyma, and vessel elements.
Figure 2. (a) Cross-sectional view of the abaca fiber, (b) internal vascular structure of fiber lumens, (c) boundary of lumen cell walls or lamellas, and (d) lumens filled with healing material (highlighted in green-yellow using Microsoft paint).
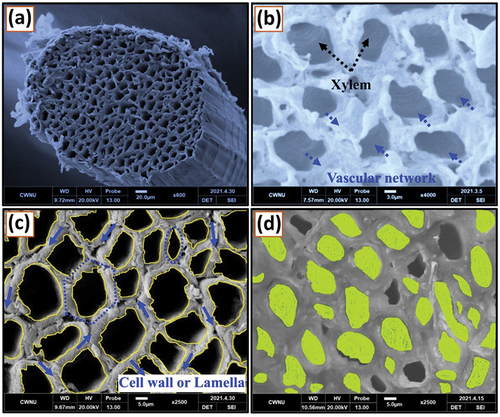
shows the lumens are separated from each other by a thick cell wall or lamella, and the structure of the lamella is in the form of circular, hexagonal, elliptical, and square shapes. The cell wall is rigid and provides the cell with structural support and protection (2021, Zijing et al. Citation2020). The cell wall is mainly composed of cellulose, hemicellulose, and pectin, while lignin is anchored onto each cell wall. The outer boundary of the lumen is highlighted with some annulus trails, indicated with yellow color in –d shows the resin-filled lumens, which have been highlighted with a green-yellow color (using MS paint). The vascular lumen structure of abaca fibers provides a significant advantage. A single fiber bundle contains around 200–300 vascular lumens, allowing for more self-healing solutions in the composites. Area of the abaca fiber (Af) = ℿr2 = 3.16 (0.198)2 = 0.124 mm2 Percentage of Lumen fraction = ALxNLx100/Af = 0.00020x243x100/0.124 = 39.9%, where AL is the area of the lumen, Af is the area of the fiber, NL isthe average number of lumens in a single fiber, and r is the radius of the abaca fiber.
Fiber lumen size, diameter, and bore
and shows the microscopic images of the cross-section of the abaca fiber lumens to gain visual access to the diameter of each lumen. A typical abaca fiber possesses lumens with different diameters that range from 4 to 30 µm, and the average diameter of a single lumen is 16.13 µm. shows the size distribution of the abaca fibers’ lumen diameters (with 10 different fibers involved). The average lumen diameter value of 16.13 µm was calculated from the 10 selected fibers. The relatively high deviation in lumen diameter was caused by the diversity of abaca fiber cells, such as ribbon and xylem fibers. Most plant fibers have a continuous lumen (bore) throughout the fiber length to transport/store minerals and food from the roots to the leaves. shows that abaca fibers possess larger lumen diameters and lumen fractions than those of other plant fibers. The large diameter of the lumens is sufficient for resin infusion and accommodates the self-healing mechanism. A set of lumens in the abaca fiber lumen network is composed of a thin layer of xylem that shields the healing resin inside the lumens. Fiber lumens are restricted by rigid cell walls and can potentially replace synthetic vascular carriers in PCs. The benefit of these lumens is that they only release resin from damaged lumens, while undamaged lumens retain the resin. These undamaged lumens aid in the second failure cycle.
Table 1. Comparison of the lumen diameter and lumen fraction in various plant fibers.
Table 2. Mechanical and self-healing properties of unidirectional abaca fibers.
Evaluation of the healing resin inside the fiber lumens
SEM analysis
shows an SEM image of the abaca fiber cross-section with empty fiber lumens before infusion of the healing resin. After successfully embedding the resin into the fiber lumen, the presence and morphology of the healing resin inside the fiber lumen were determined. shows the presence of a VE-CN core inside the fiber lumens, and the infused resin appears in the form of spherical, which confirms that the healing resin is capable of releasing from the lumens. shows that the abaca fiber lumens are filled with the MEKP catalyst because of its low viscosity. –e show the number of lumens filled with VE-CN and MEKP, after successful resin infusion. After resin infusion, nine fibers from each group were selected and characterized, and the data were reported. From the observed data, it is noticed that approximately 76% of the lumens are filled with healing liquids, which further supports the improvement of the healing mechanism.
Figure 4. Abaca fiber lumens (a) before resin infusion, (b) after core-infusion of VE-CN, and (c) after core-infusion of MEKP. Number of lumens filled with (d) resin and (e) catalyst in each fiber. (f) Cured resin inside the fiber lumens and unfilled lumens.
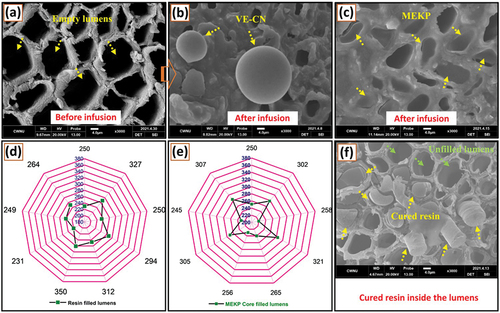
For a clear understanding of the healing liquids, the curing mechanism with the selected ratio (VE-CN:MEKP = 100-2:2) of the polymers was mixed gently and embedded into the fiber lumens via VARIM, and after 24 h of curing, the fiber cross-section was examined using SEM at different locations. From SEM analysis, it is noticed that the fiber lumens appeared with the cured resin. The fiber lumens appear to have curing resins, which confirms that the healing liquids used are embedded in the lumens and promote better polymerization when both liquids are in contact. On the other hand, some of the unfilled lumens (less than 20%) in the fiber are observed, which may be because the unfilled individual lumen outlet is closed while processing the fibers, or some of the cell walls may be damaged. However, the majority of lumens are filled, through the adopted resin-infusion technique and the healing liquids are successfully stored, as shown in .
FESEM-EDX mapping
FESEM-EDX mapping was conducted to determine the elemental composition of the abaca fiber lumens before and after infusion of the healing liquids. shows the elemental composition of the pure abaca fibers. From the data, it is noticed that the abaca fibers show empty vascular lumens consisting of carbon (C = 54.42 wt.%), oxygen (O = 45.26 wt.%), and calcium (Ca = 0.32 wt.%). As shown in , healing resin (VE-CN)-embedded inside the abaca fibers was characterized, and an embedded VE core was observed inside the lumens. The VE-CN core-embedded abaca fibers show an increased amount of carbon (C = 64.97 wt.%) owing to the presence of esters in the promoter (cobalt naphthalate (Co = 0.19 wt.%)). Cobalt naphthalate was added to the vinyl ester resin in the ratio of VE:CN = 100:2 to rapidly promote the curing process, and oxygen (O = 34.47 wt.%) and a small quantity of calcium (0.37 wt.%) were also observed. The EDX spectra of MEKP core-embedded abaca fibers also show an increased amount of carbon (C = 60.93 wt.%) due to the presence of MEKP inside the abaca lumens, while oxygen (O = 38.60 wt.%) is also observed, as shown in .
Thermogravimetric analysis (TGA)
shows the thermograms of the unidirectional pure abaca fibers and those infused with the healing materials (VE-CN and MEKP). The pure and core-embedded fibers show nearly the same onset thermal degradation temperature (Tonset) of approximately 120°C. The initial degradation is mainly due to the evaporation of free and absorbed moisture, ash, holocellulose, and alpha-cellulose in the fibers. The initial degradation of pure and core-embedded fibers occurs at around 300°C, and this degradation is mainly due to the decomposition of hemicellulose in the abaca fiber. After the initial degradation, the amounts of VE-CN and MEKP in the fibers are 30 and 10 wt.%, respectively. The variation in the amounts of MEKP and VE-CN in the abaca fibers is mainly due to the corresponding boiling points of the embedded healing materials. As the temperature increases, other constituents, such as cellulose, rapidly decompose, and lignin begins to degrade. The final degradation temperatures (Tmax) of the pure abaca fibers, as well as the MEKP and VE-CN core-embedded abaca fibers, are 396.55, 400.25, and 452.53°C, respectively. After the final decomposition temperature of the fibers, a constant decomposition trend up to 700°C is observed, which directly indicates that char remained after the complete decomposition of the fibers. The decomposed final char residues of the pure abaca fibers, MEKP, and VE-CN core-embedded abaca fibers are 16.37, 12.50, and 14.45 wt.%, respectively. According to the TGA results, the presence of a healing resin inside the fiber lumens and its evaporation during the initial degradation indicates that the fiber lumens store the healing resin.
Structural properties of healing resin-infused abaca fibers
Mechanical properties: Single-fiber tensile test
The single-fiber tensile test was conducted using a single-fiber tensile testing machine as per ASTM D3822, with a cell load capacity of 5 kgf. The tensile strengths of the pure and core-embedded abaca fibers were studied. shows that the stress-strain curves of pure abaca fibers indicate an average tensile strength of 410.63 MPa. The results show that the percentage strain is in the range of 1.7–2.7%. The tensile strengths of pure abaca, VE-CN, MEKP, and self-healed fiber showed an average strength of 410.63, 486.81, 290.80, and 100.71 MPa, respectively. The tensile strength of abaca fibers also depends on the growth of the fibers. Besides, the modulus of elasticity of pure abaca fiber with VE-CN core, abaca fiber with MEKP core, and self-healed abaca fibers showed 41.25, 43.17, 37.14, and 21.35 GPa, respectively. The abaca fibers’ tensile strength and modulus strongly depend on the growth, cellulose content, and microfibril angle (MFA). However, the stress-strain curves of unidirectional abaca fibers show abilinear-type elastic-plastic behavior. This characteristic is most common among many plant-based fibers, mainly due to the reorientation of cellulose microfibrils along the fiber axis during tensile loading (Chokshi and Gohil Citation2022, Baley Citation2002, Selvan et al. Citation2022).
Figure 7. (a) Stress-strain curves of pure abaca fibers, (b) VE-CN core-infused abaca fibers, and (c) MEKP core-infused abaca fibers. (d) Specimen preparation with sandpaper as a gripper and tensile test setup.
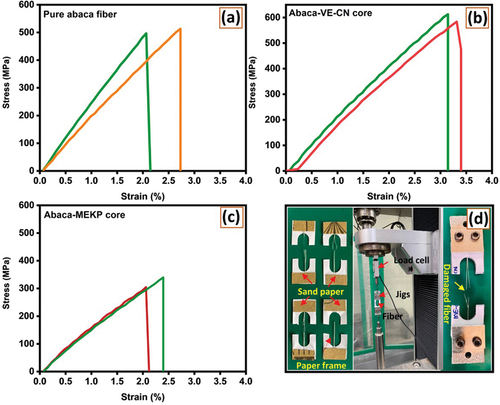
shows that the VE-CN-embedded fibers have an improved tensile strength of 486.81 MPa. The viscous resin inside the lumens increases strength in a bi-directional tensile test by reducing crack propagation between adjacent lumens. The VE-CN-embedded fibers show that the percentage of strain ranges between 3 and 3.5%, which is better than the pure (1.7–2.7%) and MEKP-core-embedded fibers (1.2–2.4%). The core embedded VE-CN abaca fibers show improved tensile strength, 18.55%, compared to pure abaca fiber. On the other hand, shows the tensile stress-strain curves of the MEKP-embedded abaca fibers and from the results presented, it is noticed that the stress-strain curves lie between percentages of strain that are in the range from 1.2 to 2.4%, which is less than that of pure abaca fibers. The fibers embedded with MEKP show an average tensile strength of 290.80 MPa. The lower strength could be due to the rapid release of MEKP at the initial fiber rupture, leading to total fiber damage and failure. clearly shows abaca fibers’ preparation and experimental testing under tensile mode with a 5 kgf cell load.
Fracture morphology of fibers
shows the SEM images of the fractured fiber surface after the single-fiber tensile test. From , it can be observed that the pure abaca fibers experience a bi-directional tension force, which results in the damage of the fibers, showing a ductile failure in the form of ribbons and threads (Ming et al. Citation2016). The fractured surface clearly shows that the split fibers exhibit ductile failure because the fibrils plastically deform before the final failure. On the other hand, the VE-CN-embedded fibers show a different fracture trend compared with pure abaca fibers. From it is noticed that the failure of the fibrils under a tension force creates a compressive force on the fiber surface, which leads to the reduction of the fiber diameter due to the healing resin being released from the lumens. The dispersion of healing resin from the lumens can be seen in , and it is noticed that the resin flows out of the damaged fibrils, and the fibrils are split into threads after their final failure. As shown in , the failure of the MEKP-core-embedded fibers is similar to that of the VE-CN-core-embedded fibers. Owing to tensile failure, the embedded healing liquids come out and remain on the surface of the broken fibrils; however, the healing phenomena is only promoted in the VE-CN-embedded fibers. Finally, it is observed from all three kinds of fibers that when the failure of fibrils begins, the fracture propagates only at the surface of the fiber cell wall. The failure of the cell walls also supports the release of healing resins from the lumen.
Self-healing properties of abaca fiber carriers
shows the stress-strain curves of the abaca fibers after self-healing. A single-fiber tensile test was conducted to examine the healing effect of core-embedded abaca fibers, in which the damaged core-embedded (VE-CN and MEKP) fibers were preferred, and the half VE-CN fiber was overlapped on the MEKP fiber and cured for 24 h at room temperature. The damaged fibers release healing agents from the lumens and have molecular interactions to promote the curing process. The cured fibers were carefully attached to the paper frame using sandpaper as a gripper, and the strength was estimated after the healing process. The average tensile strength of the self-healed fibers is 100.71 MPa. The reported strength is related to the fibrils interacting with VE-CN to the MEKP fibrils and further subsidized to heal. However, the strength is attributed to the polymerized resin of the catalyst. Owingto weakfibril interactions, the percentage strain varies between 0.8 and 1.25% for self-healed fibers. The stress-strain curves also show a jerk at different strain rates, indicating the breakage of individual self-healed fibrils.
Figure 9. (a) Tensile stress-strain curves of self-healed abaca fibers, and (b) tensile strength of pure fibers, core-infused fibers, and self-healed fibers. (c) Tensile-fractured surface of self-healed abaca fiber.
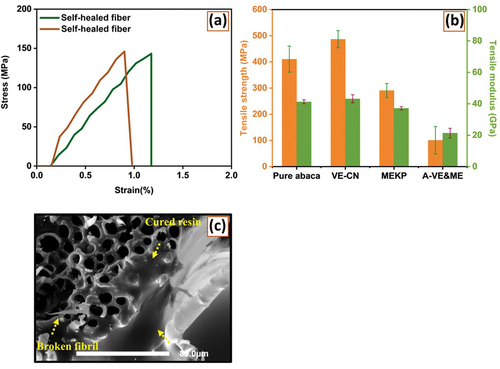
shows the single-fiber tensile strengths of the individual fibers. The tensile strengths of pure abaca, VE-CN, MEKP, and self-healed fibers show average strengths of 410.63, 486.81, 290.80, and 100.71 MPa, respectively. The achieved self-healing property with abaca vascular lumen is 34.5% more than the carbon composites reinforced with glass vascular having a hybrid resin under a low-velocity impact test (Mansingh et al. Citation2021). shows the fractured surface of the self-healed fibers after healing. It should be noted that the resin is released from the lumens after damage, prior to the healing process. The healing resin comes from the lumens and surrounds the fiber-damaged zone, interacting with the hardener for curing. Additionally, the fibers are split and appear as threads as a result of the tensile force. It is important to note that the broken surface of the self-healed abaca fiber suggests that using abaca fiber lumen systems could be essential in self-healing applications.
Conclusions
The fiber lumens were embedded with healing materials (VE-CN and MEKP) using VARIM, with an additional arrangement at the inlet. Physical parameters, were measured such as cross-sectional view, lumen structure, and cell wall. The healing core inside the lumens was confirmed using SEM, FESEM-EDX, and TGA. The mechanical and self-healing properties of the pure and core-embedded abaca fibers were tested using a single-fiber tensile test. The VE-CN core-embedded abaca fibers showed an improved strength of 76.18 MPa. However, the hardener core-embedded fiber shows a decreased strength of 119.83 MPa. This can be explained by the distribution of the healing material inside the lumens and the viscosity of the healing resins. However, the percentage range of strain for the healing core (VE-CN) embedded fibers was high (3.0–3.5%) in the tensile direction. As a result, the self-healed abaca shows a healing efficiency of 100.71 MPa after 24 hr of curing interval. Because of the self-healing characteristic of abaca fibers and their interactions, abaca fibers and their composites can be used selectively for desired attributes. After initial damage, the fibers were healed at room temperature for 24 h. The spilled fibrils were healed by releasing a healing resin in the damaged area and showed a healing efficiency of 100.71 MPa. Because of the self-healing characteristics of abaca fibers and their interactions, abaca fibers and composites can be used selectively for their desired attributes. Tensile fracture analysis allowed the release of the healing core at the fiber failure site. This is a viable option for obtaining a composite with green vascular abaca fiber lumens, which are strong and suited for several self-healing cycles in the composite.
Highlights
Introduced natural fiber lumens (NFLs) as potential self-healing carriers
Resin infusion technique is adopted to load resin/hardener into the fiber lumens
SEM, TGA, and Single fiber tensile test were confirmed the infused resin/hardener
The effective healing efficiency of about 100.71% is achieved
Ethical approval
The authors declare that they have conducted entire research with integrity, fidelity and honesty. We did not intentionally engage in or participate in any form of malicious harm to another person or animal. In addition, have no known competing financial interests or personal relationships that could have appeared to influence the work reported in this paper.
Acknowledgement
This study was supported by the Basic Science Research Program through the National Research Foundation of Korea (NRF), funded by the Ministry of Science Education (2018R1A6A1A03024509 and 2021R1A2B5B03002355).
Disclosure statement
No potential conflict of interest was reported by the author(s).
Additional information
Funding
References
- Baley, C. 2002. Analysis of the flax fibres tensile behaviour and analysis of the tensile stiffness increase. Composites Part A, Applied Science and Manufacturing 33 7:939–15. doi:10.1016/S1359-835X(02)00040-4.
- Bernal-Chávez, S. A., S. Alcalá-Alcalá, M. González-Torres, M. Luisa Del Prado-Audelo, and G. Leyva-Gómez. 2022. PG-150 distearate-PVA self-healing hydrogel: Potential application in tissue engineering. Materials Letters 308 131176. doi:10.1016/j.matlet.2021.131176.
- Chalapathi, K., M. N. P. Venkata, and J. Il Song. 2022. Impact of surface treatments and hybrid flame retardants on flammability, and thermal performance of Bamboo Paper Composites. Journal of Natural Fibers 19 6:2152–62. doi:10.1080/15440478.2020.1807436.
- Chokshi, S., and P. Gohil. 2022. Experimental investigation and mathematical modeling of longitudinally placed natural fiber reinforced polymeric composites including interphase volume fraction. Fibers and Polymers 23 2:488–501. doi:10.1007/s12221-021-2087-2.
- Chuanwei, L., Z. Ling, C. Wang, J. Wang, Q. Yong, and F. Chu. 2022. Multiple hydrogen bonding interactions toward rapidly self-healing, photothermal conversion elastomer composites. Composites Part B: Engineering 228 109428. doi:10.1016/j.compositesb.2021.109428.
- Fidelis, M. E. A., T. Vitorino Castro Pereira, and R. Dias Toledo Filho. 2013. The effect of fiber morphology on the tensile strength of natural fibers. Journal of Materials Research and Technology 2 2:149–57. doi:10.1016/j.jmrt.2013.02.003.
- Garg, A., and S. Jain. 2022. 8 - different types of self-repairing of composite materials: An overview. In Woodhead Publishing Series in Composites Science and Engineering, ed., D. Verma, M. Sharma, K. L. Goh, S. Jain, and B. T. Himani. Sustainable Biopolymer Composites Sharma 173–82. Amsterdam: Woodhead Publishing.
- Heng, A., Y. Yang, Z. Zhou, Y. Bo, Y. Wang, Y. He, D. Wang, and J. Qin. 2021. Pectin-based injectable and biodegradable self-healing hydrogels for enhanced synergistic anticancer therapy. Acta Biomaterialia 131 149–61. doi:10.1016/j.actbio.2021.06.029.
- Hu, P., S. Zhou, Y. Wang, J. Xu, S. Zhang, and J. Fu. 2022. Printable, room-temperature self-healing and full-color-tunable emissive composites for transparent panchromatic display and flexible high-level anti-counterfeiting. Chemical Engineering Journal 431 133728. doi:10.1016/j.cej.2021.133728.
- Jun-Feng, S., X.-L. Zhang, Y.-D. Guo, X.-F. Wang, L. Feng-Ling, Y. Fang, Z. Ding, and N.-X. Han. 2019. Experimental observation of the vascular self-healing hollow fibers containing rejuvenator states in bitumen. Construction and Building Materials 201 715–27. doi:10.1016/j.conbuildmat.2019.01.001.
- Kukde, S., B. Ketan Sarangi, and H. Purohit. 2019. Chapter six - antioxidant role of nanoparticles for enhancing ecological performance of plant system. In Engineered nanomaterials and phytonanotechnology: Challenges for plant sustainability, ed., S. K. Verma and K. B. T. Ashok. Comprehensive Analytical Chemistry DasVol. 87 159–87. Amsterdan: Elsevier.
- Mansingh, B. B., J. S. Binoj, N. Prem Sai, S. Abu Hassan, M. R. S. Suchart Siengchin, Y. C. Liu, and Y. C. Liu. 2021. Sustainable development in utilization of Tamarindus indica L. and its by-products in industries: A review. Current Research in Green and Sustainable Chemistry 4 100207. doi:10.1016/j.crgsc.2021.100207.
- Ming, C., H. Takagi, A. N. Nakagaito, Y. Li, and I. N. W. Geoffrey. 2016. Effect of alkali treatment on interfacial bonding in abaca fiber-reinforced composites. Composites Part A, Applied Science and Manufacturing 90 589–97. doi:10.1016/j.compositesa.2016.08.025.
- Moreira, H. R., and A. P. Marques. 2022. Vascularization in skin wound healing: where do we stand and where do we go? Current Opinion in Biotechnology 73 253. doi:10.1016/j.copbio.2021.08.019.
- Naga Kumar, C., M. N. Prabhakar, and J. Song. 2020. Result of vascular tube design on the curative and mechanical performance of modified carbon fibers/hybrid resin self‐healing composites. Polymer Composites 41 5:1913–24. doi:10.1002/pc.25507.
- Naga Kumar, C., M. N. Prabhakar, and J.-I. Song. 2021. Synthesis of vinyl ester resin-carrying PVDF green nanofibers for self-healing applications. Scientific Reports 11 1:908. doi:10.1038/s41598-020-78706-3.
- Peng, L., Y. Du, G. Liu, P. Huang, and Y. Ding. 2021. Light self-healing of resin matrix composites based on pipe network carrier. Materials Letters 288 129330.doi:10.1016/j.matlet.2021.129330.
- Sajin, J. B., R. Christu Paul, J. S. Binoj, R. S. R. I. Kheng Lim Goh, M. S. Senthil Saravanan, K. L. Goh, R. S. Rimal Isaac, and M. S. Senthil Saravanan. 2022. Impact of fiber length on mechanical, morphological and thermal analysis of chemical treated jute fiber polymer composites for sustainable applications. Current Research in Green and Sustainable Chemistry 5 100241. doi:10.1016/j.crgsc.2021.100241.
- Selvan, M. T. G. A., J. Selvi Binoj, S. Siengchin, M. Rangappa Sanjay, Y. Liu, M. R. Sanjay, and Y. Liu. 2022. Extraction and characterization of natural cellulosic fiber from fragrant screw pine prop roots as potential reinforcement for polymer composites. Polymer Composites 43 1:320–29. doi:10.1002/pc.26376.
- Sinha, A. K., S. Bhattacharya, and H. Kumar. 2021. Abaca fibre reinforced polymer composites: a review. Journal of Materials Science 56 7:4569–87. doi:10.1007/s10853-020-05572-9.
- Swamy, B. Y., M. N. P. Chavidi Venkata Prasad, M. C. S. S. Kashay Chowdoji Rao, I. Chung, M. C. S. Subha, and I. Chung. 2013. Biodegradable chitosan-g-poly (methacrylamide) microspheres for controlled release of hypertensive drug. Journal of Polymers and the Environment 21 4:1128–34. doi:10.1007/s10924-012-0554-y.
- Venkata Chalapathi, K., R. Kumar Cheedarala, and J. Il Song. 2021. Synthesis of restorative microcapsules having vinyl ester healing medicine for hybrid resin/modified carbon fiber self-healing composites. Journal of Applied Polymer Science 138 16:1. doi:10.1002/app.50227.
- Wang, X., Z. Chen, J. Ren, S. Chen, and F. Xing. 2022. Object status identification of x-ray ct images of microcapsule-based self-healing mortar. Cement and Concrete Composites 125 104294. doi:10.1016/j.cemconcomp.2021.104294.
- Wang, H., J. Xu, X. Du, Z. Du, X. Cheng, and H. Wang. 2021. A self-healing polyurethane-based composite coating with high strength and anti-corrosion properties for metal protection. Composites Part B: Engineering 225 109273. doi:10.1016/j.compositesb.2021.109273.
- Yan, L., H. Ma, Y. Shen, Q. Li, and Z. Zheng. 2015. Effects of resin inside fiber lumen on the mechanical properties of sisal fiber reinforced composites. Composites Science and Technology 108 32–40. doi:10.1016/j.compscitech.2015.01.003.
- Yong-Chao, W., J.-L. Shao, and H. Zhan. 2021. Damage and self-healing characteristics of monolayer graphene enhanced cu under ballistic impact. Mechanics of Materials 155 103736. doi:10.1016/j.mechmat.2020.103736.
- Zhang, X., P. Zhu, and Z. Zhong. 2022. A chemo-mechanically coupled continuum damage-healing model for chemical reaction-based self-healing materials. International Journal of Solids and Structures 236–237 111346. doi:10.1016/j.ijsolstr.2021.111346.
- Zhao, J., G. Ji, X. Zhang, R. Hu, and J. Zheng. 2021. Preparation of a high strength, rapid self-healing composite gel and its application in electrochemical capacitor. Polymer 214 123372. doi:10.1016/j.polymer.2020.123372.
- Zhao, X., W. Tu, Q. Chen, and G. Wang. 2021. Progressive modeling of transverse thermal conductivity of unidirectional natural fiber composites. International Journal of Thermal Sciences 162 106782. doi:10.1016/j.ijthermalsci.2020.106782.
- Zhu, J., H. Wang, F. Guo, L. Salmén, and Y. Yu. 2021. Cell wall polymer distribution in bamboo visualized with in situ imaging FTIR. Carbohydrate Polymers 274 118653. doi:10.1016/j.carbpol.2021.118653.
- Zijing, L., L. R. de Souza, C. Litina, A. E. Markaki, and A. Al-Tabbaa. 2020. A novel biomimetic design of a 3d vascular structure for self-healing in cementitious materials using murray’s law. Materials & Design 190 108572. doi:10.1016/j.matdes.2020.108572.