ABSTRACT
Anterior cruciate ligament (ACL) rupture is one of the most common knee injuries. Due to the limited self-healing capacity, complex structure, and mechanical environment, the regeneration of ACL remains a challenge. To achieve fast recovery and long-term ACL regeneration, biodegradable scaffolds with excellent initial mechanical support and cell recruitment ability have gained increasing research interest. The aptamer-functionalized silk fiber was fabricated by embedding silk fiber into morpholinoethanesulfonic/1-ethyl-3-[3-dimethylaminopropyl] carbodiimide hydrochloride, N-hydroxysuccinimide buffer with aptamer 19s (Apt19s) and characterized in vitro. The clinically size-matched silk ligament was braided utilizing commercial methodology (polyethylene terephthalate ligament), and the tensile strength was analyzed. A total of 18 rabbits were used to test the early mesenchymal stem cell (MSC) recruitment of Apt19s-functionalized silk ligaments. Macroscopic analysis and hematoxylin and eosin and immunohistochemistry stainings were applied at 3, 5, and 7 days post-implantation. The ultimate strength of silk fiber was lower than that of PET fibers. However, the prepared silk ligament had comparable mechanical strength to the native ACL. In vitro, the Apt19s-functionalized fiber recruited bone marrow-derived MSCs. In vivo, Apt19s-functionalized silk ligament enhanced early MSCs recruitment after implantation in comparison with the aptamer-free ligament. The Apt19s-functionalized scaffold silk ligament exhibited comparable mechanical strength with native ACL and enhanced endogenous MSC homing.
摘要
前交叉韧带(ACL)断裂是最常见的膝关节损伤之一. 由于自我修复能力有限、结构复杂和机械环境,ACL的再生仍然是一个挑战. 为了实现ACL的快速恢复和长期再生,具有良好初始机械支撑和细胞募集能力的生物可降解支架已引起越来越多的研究兴趣. 通过将丝纤维嵌入MES(吗啉乙磺酸)/EDC(1-乙基-3-[3-二甲基氨基丙基]碳二亚胺盐酸盐)、NHS(N-羟基琥珀酰亚胺)缓冲液(含Apt19s)中,制备了适配体功能化丝纤维,并在体外进行了表征. 使用商业方法(PET韧带)编织临床尺寸匹配的丝韧带,并分析拉伸强度. 共18只兔子用于测试Apt19s功能化丝韧带的早期间充质干细胞(MSC)募集. 植入后3、5和7天进行大体分析、苏木精和伊红染色和免疫组织化学染色. 蚕丝纤维的极限强度低于PET纤维. 但制备的蚕丝韧带具有与天然ACL相当的机械强度. 在体外,apt19s功能化蚕丝纤维可招募骨髓来源的MSCs. 在体内,与无适配体韧带相比,Apt19s功能化的蚕丝韧带增强了植入后MSCs的早期募集. Apt19s功能化支架蚕丝丝韧带显示出与天然ACL相当的机械强度,并增强了内源性MSC归巢.
Introduction
Anterior cruciate ligament (ACL) is a dense connective tissue responsible for the stability of knee joints (Blaker et al. Citation2021; Frank and Jackson Citation1997). Unlike medial collateral ligament (MCL), ACL has poor self-healing capacity due to its hypocellularity and hypovascularity, and ACL rupture often leads to joint instability, articular cartilage wear, and eventually osteoarthritis (Chalmers et al. Citation2014; Ficek et al. Citation2019). Based on demographic analysis, there are at least 30 million tendon injuries annually, which costs more than $30 billion per year (Chen et al. Citation2021). Autograft, allograft, and artificial ligaments are the main grafts used for ACL reconstruction in clinics (Herzog et al. Citation2018; Schindler Citation2012). However, donor site damage in autograft, risk of immune rejection and synovitis, or graft rupture in polyethylene terephthalate (PET) ligament applications have severely limited the clinical outcome in these patients (Batty et al. Citation2015; Li et al. Citation2012). Studies of tissue engineering ligaments based on biodegradable materials (such as collagen and chitosan) aim to overcome the drawbacks facing these three challenges (Nau Citation2015).
Silk is a Food and Drug Administration (FDA)-approved natural biomaterial with good biocompatibility, excellent mechanical properties, and biodegradability (Fazal and Latief Citation2018; Yodmuang et al. Citation2015). Recently, silk fiber and silk fibroin have been applied to fabricate artificial ligaments in competition with other synthetic fibers. Kaplan et al. prepared silkworm fiber matrices that matched the mechanical requirements of native human ACL, including adequate fatigue performance (Altman et al. Citation2002). To further enhance bioactivity and potential for tissue regeneration based on current mechanical property, basic fibroblast growth factor–releasing poly lactic-co-glycolic acid fibers were knitted into microfibrous silk scaffolds (Sahoo, Toh, and Goh Citation2010). In a year-long controlled laboratory study, silk fiber-based scaffold stimulated ACL regeneration in large animal models, and additional cell seeding further enhanced tissue regeneration at 6 months (Teuschl et al. Citation2016). In addition, Chen et al. coated silk fibroin and hydroxyapatite with PET ligament to improve its ligamentization and osseointegration processes (Cai et al. Citation2018). One of the most recent studies produced silk protein/laponite hybrid fibers by wet-spinning process and proved its great potential for artificial ligament application (Dong et al. Citation2020).
In addition to mechanical support of artificial ligament in the early stage of reconstruction, cells including native somatic cells or recruited mesenchymal stem cells (MSCs) involved in the later tissue remodeling are another critical point during ligament maturation (Vavken and Murray Citation2010). MSCs are the most widely used cell source in cell-based ligament tissue engineering. However, allogeneic MSCs may cause immune rejection and pathophoresis, and in vitro expansion of autologous MSCs has the risks of contamination and phenotype loss (Andia and Maffulli Citation2017). Thus, recruiting endogenous MSCs to participate in ligament repair has been given much attention (Embree et al. Citation2016). Aptamers are short stretches of nucleotides or amino acid residues, which bind to specific targets, such as small chemical groups, large proteins, and even cells. (Zhu and Chen Citation2018) We previously successfully applied an aptamer named aptamer 19s (Apt19s) to enhance cartilage repair by improving stem cell recruitment ability of a silk fibroin bioscaffold (Wang et al. Citation2019). Hence, we hypothesized that Apt19s could be conjugated to silk fibers and endow silk ligament MSCs recruiting ability to facilitate ACL reconstruction.
In this study, we compared the mechanical properties of degummed silk fibers with PET fibers (raw material of LARS® ligament)-based artificial ligaments. The silk ligament was fabricated with human ACL specification. The biocompatibility and MSC recruitability of the Apt19s conjugated silk fibers and silk ligaments were detected in vitro and in vivo. The study design and experimental scheme is shown in . Our data demonstrated that silk fiber-braided ligaments could match the basic need of ACL in mechanical support; in addition, the functionalization of Apt19s in silk ligament was biocompatible and enhanced early MSCs recruitment after implantation.
Figure 1. Study design and experimental scheme for the aptamer-functionalized silk ligamentThe carboxyl groups of silk fibers were activated by adding 1-ethyl-3-(3-dimethylaminopropyl) carbodiimide hydrochloride and N-hydroxysuccinimide. The aptamer-functionalized silk fibers was prepared by conjugation of amino-modified aptamer 19s in phosphate buffer solution solution. Fibers with linear density of 1000 D which consisted of three silk fiber bundles were used to fabricate silk ligaments. EDC, 1-ethyl-3-[3-dimethylaminopropyl] carbodiimide hydrochloride; MES, morpholinoethanesulfonic acid; NHS, N-hydroxysuccinimide; Rb BMSCs, rabbit bone marrow mesenchymal stem cells.
![Figure 1. Study design and experimental scheme for the aptamer-functionalized silk ligamentThe carboxyl groups of silk fibers were activated by adding 1-ethyl-3-(3-dimethylaminopropyl) carbodiimide hydrochloride and N-hydroxysuccinimide. The aptamer-functionalized silk fibers was prepared by conjugation of amino-modified aptamer 19s in phosphate buffer solution solution. Fibers with linear density of 1000 D which consisted of three silk fiber bundles were used to fabricate silk ligaments. EDC, 1-ethyl-3-[3-dimethylaminopropyl] carbodiimide hydrochloride; MES, morpholinoethanesulfonic acid; NHS, N-hydroxysuccinimide; Rb BMSCs, rabbit bone marrow mesenchymal stem cells.](/cms/asset/ad8bfa20-e313-4639-af96-9f20edc43a41/wjnf_a_2153194_f0001_oc.jpg)
Methods
Micromorphology of silk and PET fibers. Degummed Bombyx mori silk fibers (kindly provided by the Institute of Biotechnology at Southwest University) and PET fibers (kindly provided by Kinetic Co., Ltd.) of 110dtex were freeze-dried and sputter-coated with gold. The morphologies of the fibers were viewed using HitachiS-3400N scanning electron microscope (SEM) operated at 15.0 kV accelerating voltage.
Preparation of aptamer-conjugated silk fibers. The binding of aptamer (amino-modified Apt19s: 5“-NH2-(A)9-AGGTCAGATGAGGAGGGGGACTTAGGACTGGGTTTATGACCTATGCGTG-3,” synthesized by Sangon Biotech) to silk fibers was performed according to our previous study (Wang et al. Citation2019). Briefly, 5 mg silk fibers were immersed in 2 mL of morpholinoethanesulfonic acid buffer (MES, 0.1 M, pH = 6, Macklin). The carboxyl groups of silk fibers were activated by adding 8 mg of 1-ethyl-3-(3-dimethylaminopropyl) carbodiimide hydrochloride (EDC, J&K) and 12 mg of N-hydroxysuccinimide (NHS, J&K) for 15 min at room temperature. Then, the activation buffer was removed, and the silk fibers were washed twice with phosphate buffer solution (PBS). For the conjugation of aptamer, 4 nmol of amino-modified Apt19s in 2 mL PBS was added and reacted for 12 h in a reciprocating oscillator. Finally, the aptamer-functionalized silk fibers were washed twice with PBS.
Preparation of silk ligament and mechanical testing. Degummed silk strands with a linear density of 1000 D which consisted of three silk fiber bundles (kindly provided by the Institute of Biotechnology at Southwest University) were used to fabricate silk ligaments. Clinically size-matched silk ligaments were braided by Kinetic Co., Ltd (Shanghai, China) utilizing PET ligament production method. The 2-mm diameter silk ligaments for rabbit ACL reconstruction were fabricated based on previously description (Altman et al. Citation2002; Cai et al. Citation2013). Briefly, three silk strands were knitted into a three-ply braid, and the braids were twisted into a diameter of ~2 mm ligament. Finally, the tensile strength of the fibers and knitted silk ligaments with known size were measured using Instron 5969 testing frame (Instron Instruments) with a pre-strain force of 0.1 N and a constant stretching speed of 30 mm/min until rupture or 100% strain.
Isolation and cell culture of rabbit bone marrow-derived MSCs (BMSCs). Rabbit BMSCs were harvested from 3-month-old New Zealand White rabbits according to our previous procedure (Wang et al. Citation2019). Cells were cultured in Dulbecco’s modified Eagle’s medium (DMEM, Invitrogen) containing 10% fetal bovine serum (FBS, Gibco) and 1% penicillin/streptomycin (Beyotime). Medium was changed every 2 or 3 days. MSCs at passage 3 were used in the following experiments.
Aptamer-binding assay. Rabbit MSCs at 70% confluence were washed with warm PBS and incubated with 10 nMFAM-labeled aptamer Apt19s (5“-AGGTCAGATGAGGAGGGGGACTTAGGACTGGGTTTATGACCTATGCGTG-FAM-3,” synthesized by Sangon Biotech) for 3 h. Cells were washed twice with PBS and imaged under fluorescence microscope (IX71, Olympus). The binding efficiency was determined as the percentage of fluorescent cells in three random views of the images. To verify the specific binding of aptamer to MSCs, rabbit chondrocytes served as negative control.
Cell migration assay. The ability of aptamer-conjugated silk fibers to recruit MSCs was assessed by cell migration assay in a transwell model (Millipore, 8 μm). Briefly, 10 × 104 MSCs in 100 μL of DMEM were placed in the upper chamber, and silk fibers (with or without Apt19s) were put in the lower chamber in the presence of 1 mL DMEM containing 10% FBS. After 48 h, the cells were fixed with 4% paraformaldehyde and stained with crystal violet. The MSCs recruitment capacity of Apt19s-conjugated silk fibers was evaluated by the number of cells migrated to the other side of the membrane.
Biocompatibility of aptamer-conjugated silk fibers. To measure the cytotoxicity of Apt19s-functionalized silk fibers, the extracted liquid was first prepared by soaking 50 mg silk fibers in 10 mL DMEM at 37°C for 24 h, and sterilized with 0.22 μm filter (Millipore). Then, MSCs were seeded on 96-well plates (5 × 103 cells/well) 12 h before adding the extracted liquid (10 µL/100 µL DMEM). Finally, after 24 h, 48 h, and 72 h, co-culture of silk fiber extracts with cells, the Cell Counting Kit-8 (CCK-8, Biosharp) was used to evaluate the cytotoxicity. An equal amount of culture medium was used as control.
The adhesion ability of MSCs to the silk fibers (with or without Apt19s) was tested by co-culturing 20 silk fibers with 5 × 104 MSCs suspension in six-well culture plate for 2 h at 37°C, 5% CO2. The difference of MSCs adhesion was visualized with a microscope (Olympus X71).
Animal and surgical procedure. All procedures were in accordance with the Guide for the Care and Use of Laboratory Animals and were approved by the Institutional Animal Care and Use Committee. Eighteen New Zealand White rabbits (female, 2.5-3.0 kg) randomly divided into experiment group (Apt-silk ligament, n = 9) and control group (pure silk ligament, n = 9) were used for the study. Under anesthesia with pentobarbital sodium (50 mg/kg), the ACL was cutoff and the residual ACL attachment points at tibial and femoral were reserved. 2.0-mm tunnels were drilled following the anatomical ACL footprints according to the attachment points. A Kirschner wire was used to guide the scaffold through the tunnel, and either end of the scaffold was suture fixed to the periosteum. The animals were fed separately in a unique cage, according to the grouping information. At 3, 5, and 7 days post-implantation, the rabbits were sacrificed, and the samples (n = 3/time point/group) were collected for histologic observation.
Histologic analysis. Specimens were fixed in 10% formaldehyde, decalcified in ethylene diamine tetraacetic acid for 3–4 weeks, dehydrated in a graded ethanol series, and embedded in paraffin. Samples were cut in the sagittal plane into 4-µm-thick sections. Paraffin sections were subjected to hematoxylin and eosin (HE) and immunohistochemical staining of MSC marker CD90/CD105.
Statistical analysis. All data were presented as mean ± standard deviation. Statistical analysis was performed using SPSS software (version 21.0; IBM). Normal analysis was performed using Quantile–Quantile plots. One-way analysis of variance and Student’s t-test were used to calculate the differences between groups. P < .05 was considered statistically significant.
Results
Morphology and mechanical properties of silk fibers and PET fibers
Due to the immunogenicity of sericin (Altman et al. Citation2003), SEM was carried out to make sure sericin was completely removed from the surface of silk fiber. As shown in , the surface of the degummed silk fibers was smooth without sericin residual. The diameter of silk fibers (5-10um) was close to that of PET fibers. Mechanical properties of both single silk and PET fibers were characterized by the Instron testing frame. As shown in , the break load of PET fibers was 4.2 cN/dtex, which is five times higher than that of silk fibers.
Figure 2. Gross appearance and mechanical properties of the braided ligaments. (A) Gross appearance and scanning electron microscope images of degummed silk fibers (a) and PET fibers. (B) Machine-braided silk ligament and PET ligament with φ = 7 mm. (C) Maximum load of silk-braided ligament. (D) Gross appearance of the silk ligament for animal study with φ = 2 mm. (E) Stress strain curves and quantitative mechanical properties of the silk ligaments with φ = 2 mm.
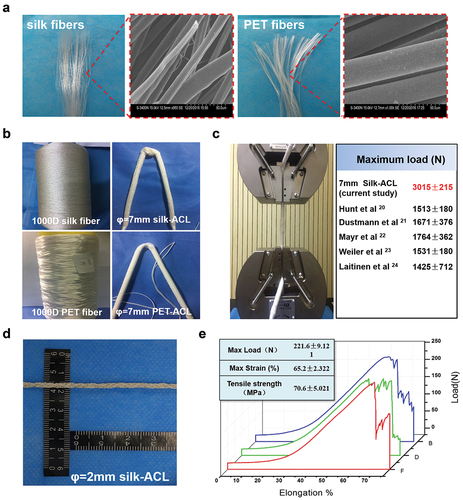
Table 1. Mechanical properties of 110 dtex silk and PET fiber.
Preparation of silk ligament according to artificial ligament method and mechanical properties of silk ligament for rabbit ACL reconstruction
To verify the feasibility of silk-based artificial ligaments with our prepared silk fibers, silk ligament with 7 mm diameter was braided using 1000 D silk strands based on the existing artificial ligament method (). Mechanical test revealed that the ultimate tensile strength of the silk ligament was 3000 N higher than that of native human ligaments () (Dustmann et al. Citation2008; Hunt et al. Citation2005; Laitinen et al. Citation1993; Mayr et al. Citation2012; Weiler et al. Citation2002).
For animal study applications, as shown in , silk and PET ligaments with diameter of 2 mm based on the size of rabbit ACL (Han et al. Citation2019) were braided using the same methodology. Mechanical test results () revealed that the maximum load was 221.6 ± 9.121 N, which was higher than that of native rabbit ACL ligament (Bascuñán et al. Citation2019). Based on these data, we speculated that silk fibers could meet the primary needs of providing mechanical support as raw material for artificial ligaments.
Binding specification of Apt19s and MSC recruitment of aptamer functionalized silk fibers
The binding specificity of Apt19s toward MSCs was confirmed by incubating FAM-conjugated Apt19s with rabbit BMSCs and with rabbit chondrocytes as control. After addition of FAM-conjugated Apt19s to culture medium for 3 h, strong green fluorescence was detected in most MSCs, while hardly any fluorescence was observed in chondrocytes (). On average of three different areas, the percentage of FAM-labeled BMSCs was 93.70% ± 5.93%, while that of FAM-labeled chondrocytes was only 11.03% ± 5.22% (P = .005) ().
Figure 3. Recruitment of MSCs with Apt19s in vitro (A) Apt19s binding specificity assay: fluorescent images of the binding of FAM (5-carboxyfluorescein)-labeled Apt19s with rabbit BMSCs and rabbit chondrocytes. (B) Quantitative data of aptamer binding specificity assay. (C) Schematic diagram of the cell migration assay via the Transwell model, and the migrated MSCs toward the aptamer-free/functionalized (Apt-/apt+) silk fiber were dyed with crystal violet. (D) Quantitative data of MSC migration.
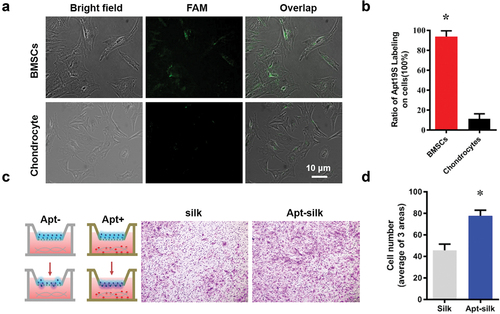
The transwell system was used to confirm MSC recruitment of Apt19s-functionalized silk fibers in vitro (). Compared with the aptamer-free silk fibers, the Apt19s-functionalized silk fibers attracted more BMSCs to migrate to the other side of the membrane. On average of three views, there were 78 BMSCs migrating toward the Apt19s-functionalized silk fibers but only 46 MSCs to the Apt19s-free silk fibers (P = .002) ().
Biocompatibility and BMSCs adhesion of aptamer-functionalized silk fibers
For cytotoxicity of the Apt19s-functionalized silk fibers, the CCK-8 assay result () showed that the cell number in the extracted liquid group was higher than the culture medium group at 24 h, but no difference at 48 h and 72 h, indicating the Apt19s-functionalized silk fibers had no side effect on the growth of BMSCs. shows typical attachment images of BMSCs to the Apt19s-functionalized silk fibers and pure silk fibers. After co-culture of the two fibers with BMSCs suspension for 2 h, we found more MSCs attached to the Apt19s-functionalized silk fibers than pure silk fibers, indicating that Apt19s helped recruit BMSCs.
Gross and histologic evaluation
We aimed to evaluate the MSCs recruitment ability of our Apt19s-functionalized silk fiber design. No rabbit was infected or died throughout the experiment; articular joint samples including implanted ACL were harvested at 3, 5, and 7 days after surgery for gross and histologic evaluation. shows our ACL reconstruction processes in rabbit models. There is no evident sign of infection or inflammatory response in any of the rabbits (). The articular cavities were clean without fester or hydrops which proved our silk ligaments were biocompatible and nonimmunogenicity.
Figure 5. Rabbit ACL reconstruction processes and sample collection. (A) Diagram of our rabbit ACL reconstruction experiment with Apt-silk ligaments. (1) Anesthesia and sterilize, (2) ACL cutoff, (3) anterior drawer test, (4) tibial tunnel creation, (5) ligament implantation, (6) anterior drawer test. (B) Gross appearance of articular cavity 3, 5, 7 days post-operation, white arrow indicates silk ligament.
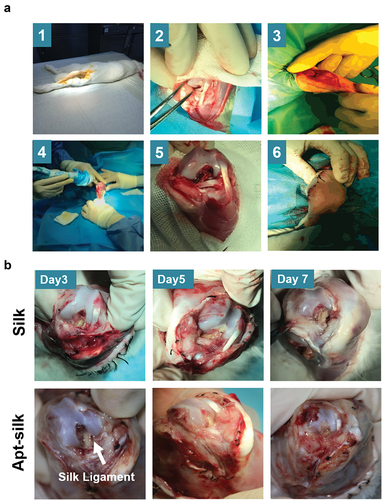
Histologic analysis of cell infiltration into artificial ligaments was carried out via hematoxylin and eosin staining. The number of infiltrated cells increased in both pure silk ligament group and Apt19s-functionalized silk ligament group post-operatively (). Compared to the two groups at the same time point, there is more cell ingrowth in Apt19s-functionalized silk ligament than pure silk group. At day 7 post-operatively, the number of infiltrated cells was obviously higher in Apt19s-functionalized silk fibers than pure silk fibers. Immunohistochemical staining of MSC marker CD90 and CD105 were carried out to further confirm cell phenotype (Dominici et al. Citation2006). The results () showed that there were more CD90/CD105 positive cells in Apt19s-functionalized silk ligament than in pure silk ligament group.
Figure 6. Histologic analysis of cell infiltration into the artificial ligaments. (A) Representative HE staining images of the rabbit joint samples with silk ligaments. Black arrow indicates infiltrated cells. (B) Immunohistochemistry of mesenchymal stem cell marker CD90/CD105 of the recruited cells at Day 7. The silk ligament-implanted interface with bone tunnel is outlined with a red rectangle and magnified in close-up views.
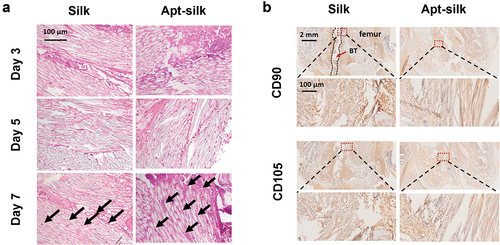
Discussion
In this study, silk ligament with MSC recruitment ability was fabricated by conjugating Apt19s to silk fibers, followed by clinically applicable size ligament preparation with an established braiding method. The prepared silk ligament had comparable mechanical strength with human native ACL. In the rabbit ACL reconstruction model, the Apt19s-functionalized silk ligament demonstrated significantly stronger MSCs recruitment at 3, 5, and 7 days postreconstruction, proving the effective design of the present ACL scaffold.
ACL rupture is one of the most common knee injuries in clinical practice. Due to the limited healing capacity, complex structure, and mechanical environment, the regeneration of ACL remains a challenge (Leong, Petrigliano, and McAllister Citation2014). In order to achieve fast recovery and quick return to sports, there is growing interest in tissue engineering ligament applications. Within this arena, PET ligament exhibited excellent mechanical properties and achieved good clinical outcomes. However, PET suffers from drawbacks such as the nondegradability, increased risk of synovitis, graft rupture, and bone tunnel enlargement (Batty et al. Citation2015; Li et al. Citation2012). On the other hand, most biodegradable ligaments provide poor initial mechanical support (Caruso and Dunn Citation2005; Koob et al. Citation2001; Nau Citation2015) and rapid degradation in vivo (Bellincampi et al. Citation1998; Dunn et al. Citation1995). Therefore, efforts have still been made to develop biodegradable artificial ligament which is mechanically strong but also biocompatible.
As the FDA-proved biomaterial, in vivo studies have proven the possibility of clinical application of silk-based scaffolds (Holland et al. Citation2019; Thurber, Omenetto, and Kaplan Citation2015; Zhang et al. Citation2017). Recently, silk has been considered as an attractive candidate for artificial ligament due to its good biocompatibility, mechanical properties, and ideal in vivo degradation rate. Although the ultimate strength of silk fiber was lower than that of PET fibers in our study, followed-up tests indicated that the silk fiber base ligament with clinically applicable size was comparable with native human ACL in tensile strength. Furthermore, it has been suggested that slow in vivo degradation rate of silk fibers provided ideal time frame for autologous ACL tissue regeneration while providing mechanical support. The main component of silk fiber is silk fibroin which consists of light (26 kDa) and heavy chain (325 kDa) of a glycoprotein in a ratio of 1:1, linked by a disulfide bond (Valluzzi et al. Citation2002). The repetitive element Gly-Ala-Gly-Ala-Gly-Ser in the heavy chain of silk fibroin helps the hydrophobic crystalline β-sheets formation, making silk hard to be hydrolyzed or degraded by proteases (Li, Ogiso, and Minoura Citation2003). In animal studies, comparison analysis was performed among absorbable and nondegradable sutures. The results indicated 55% and 16% lost in tensile strength and elastic modulus of silk fibers at 6 weeks post-subcutaneously implantation, respectively (Greenwald et al. Citation1994). While another study using the same animal model proposed 73% loss of tensile strength in silk fibers at 30 days post-implantation (Bucknall, Teare, and Ellis Citation1983), it indicated faster degradation. Despite the differences in methodology used for mechanical analyses, the rate of degradation is dependent upon the implantation site, microenvironment, and subtype of silk fibers (Salthouse, Matlaga, and Wykoff Citation1977; Soong and Kenyon Citation1984). Common to all implants, inflammation is the main mechanism for degradation. In the present study, sericin was removed during silk ligament fabrication to minimize foreign body response (Kurosaki et al. Citation1999). Based on the histological data during 1-week post-ACL reconstruction, we found no evident signs of infection or inflammatory response in knee joint. However, the extended observation time period, as well as the reduction in mechanical property of implanted silk ligaments were not included in the present study. In order to explore the balance between tissue regeneration and scaffold degradation, further studies are required.
Noticing the important role of cell sources in ACL regeneration, efforts have been made to increase cellularity in ACL scaffolds. Recent study using silk fiber-based scaffolds in sheep ACL resection model indicated that additional stem cell seeding increased the regenerative activity and decreased silk fiber content (Teuschl et al. Citation2016). In addition, stem cell recruitment strategy involving chemokine has also been proven to be promising for ACL regeneration (Hu et al. Citation2018). In the present study, we conjugated Apt19s onto silk fibers to enhance the recruitment of endogenous MSCs from bone tunnel. The same method has been proven effective in directing the homing of joint-resident MSCs in our previous study (Wang et al. Citation2019). Comparing with other MSC recruit factors including antibodies, growth factors, and surface modification, aptamers are much easier to prepare and scale up nonimmunogenic and could be conjugated with dyes or functional groups. The Apt19s we used in this study was first selected by Hou et al. (Hou et al. Citation2015), and later applied to cartilage repair by directing the homing of endogenous MSC by Quan Yuan et al. (Hu et al. Citation2017) and our group. In the present study, both in vitro and in vivo data verified that Apt19s is promising targeting ligand for recruiting native MSC onto silk ligament for ACL repair. However, the survival and proliferation of migrated MSCs and its participation in ACL tissue remodeling still need long-term observation.
Apart from MSC infiltration and neoligament regeneration, tendon–bone healing is also important for osteointegration at the tendon–bone interface after ACL reconstruction. Hydroxyapatite modification at both ends of a silk-collagen ligament was found to promote osteointegration at the tendon–bone interface (Bi et al. Citation2021). The addition of laponite into artificial ligament which is made of wet-spinning produced silk fibers could significantly enhance the graft osseointegration process and also improve the corresponding biomechanical properties of the artificial ligament (Dong et al. Citation2020). Silk fibroin tubular grafts containing ZnSr-doped-tricalcium phosphate particles used as bone tunnel fillers were capable of connecting to the ACL tissue while stimulating bone tissue regeneration for a faster osteointegration (Ribeiro et al. Citation2022). We did not observe the tendonbone healing results in this study, and relative modification methods could be introduced to the present aptamer-conjugated silk ligament to enhance its osteointegration ability in the future study.
In conclusion, we braided an artificial ligament with clinically available silk fibers and functionalized with the nonimmunogenic aptamer Apt19s to obtain the endogenous MSCs recruitment ability. Our results demonstrated that the silk ligament has mechanical properties mimicking native ACL and the aptamer conjugation enhances endogenous stem cell homing. Hence, the aptamer-functionalized silk ligament may offer a new tissue-engineering approach for ligament injury treatment.
Highlights
Anterior cruciate ligament (ACL) rupture is one of the most common knee injuries in clinics, artificial ligaments, mainly polyethylene terephthalate (PET) ligament is non-degradable and may induce synovitis or graft rupture. In this study, we used silk fiber to fabricate artificial ligament, which is a Food and Drug Administratgion -approved natural biomaterial with good biocompatibility, excellent mechanical properties, and biodegradability.
We were the first to fabricate silk ligaments with human ACL specifications using industrial parameter, and the prepared silk ligament had comparable mechanical strength with human native ACL, proving its potential in large-scale application.
Our data demonstrated that silk fiber braided ligaments could match the basic need of ACL in mechanical support, in addition, the functionalization of Apt19s was biocompatible and enhanced early MSCs recruitment after implantation.
Acknowledgments
We thank the technical support in histologic staining offered by Knorigene Technologies.
Disclosure statement
No potential conflict of interest was reported by the author(s).
Correction Statement
This article has been republished with minor changes. These changes do not impact the academic content of the article.
Additional information
Funding
References
- Altman, G. H., F. Diaz, C. Jakuba, T. Calabro, R. L. Horan, J. Chen, H. Lu, J. Richmond, and D. L. Kaplan. 2003. Silk-based biomaterials. Biomaterials 24 (3):401–14. doi:10.1016/s0142-9612(02)00353-8.
- Altman, G. H., R. L. Horan, H. H. Lu, J. Moreau, I. Martin, J. C. Richmond, and D. L. Kaplan. 2002. Silk matrix for tissue engineered anterior cruciate ligaments. Biomaterials 23 (20):4131–41. doi:10.1016/s0142-9612(02)00156-4.
- Andia, I., and N. Maffulli. 2017. Biological Therapies in Regenerative Sports Medicine. Sports Med 47 (5):807–28. doi:10.1007/s40279-016-0620-z.
- Bascuñán, A. L., A. Biedrzycki, S. A. Banks, D. D. Lewis, and S. E. Kim. 2019. Large Animal Models for Anterior Cruciate Ligament Research. Frontiers in Veterinary Science no. 6 (6):292. doi:10.3389/fvets.2019.00292.
- Batty, L. M., C. J. Norsworthy, N. J. Lash, J. Wasiak, A. K. Richmond, and J. A. Feller. 2015. Synthetic devices for reconstructive surgery of the cruciate ligaments: A systematic review. Arthroscopy: The Journal of Arthroscopic & Related Surgery 31 (5):957–68. doi:10.1016/j.arthro.2014.11.032.
- Bellincampi, L. D., R. F. Closkey, R. Prasad, J. P. Zawadsky, and M. G. Dunn. 1998. Viability of fibroblast-seeded ligament analogs after autogenous implantation. Journal of Orthopaedic Research 16 (4):414–20. doi:10.1002/jor.1100160404.
- Bi, F., Y. Chen, J. Liu, Y. Wang, D. Xu, and K. Tian. 2021. Anterior cruciate ligament reconstruction in a rabbit model using a silk-collagen scaffold modified by hydroxyapatite at both ends: A histological and biomechanical study. Journal of Orthopaedic Surgery and Research 16 (1):139. doi:10.1186/s13018-021-02281-0.
- Blaker, C. L., S. Zaki, C. B. Little, and E. C. Clarke. 2021. Long-term Effect of a Single Subcritical Knee Injury: Increasing the Risk of Anterior Cruciate Ligament Rupture and Osteoarthritis. The American Journal of Sports Medicine 49 (2):391–403. doi:10.1177/0363546520977505.
- Bucknall, T. E., L. Teare, and H. Ellis. 1983. The choice of a suture to close abdominal incisions. European Surgical Research 15 (2):59–66. doi:10.1159/000128334.
- Cai, C., C. Chen, G. Chen, F. Wang, L. Guo, L. Yin, D. Feng, and L. Yang. 2013. Type I collagen and polyvinyl alcohol blend fiber scaffold for anterior cruciate ligament reconstruction. Biomedical Materials 8 (3):035001. doi:10.1088/1748-6041/8/3/035001.
- Cai, J., F. Wan, Q. Dong, J. Jiang, C. Ai, D. Sheng, W. Jin, X. Liu, Y. Zhi, S. Wang, et al. 2018. Silk fibroin and hydroxyapatite segmented coating enhances graft ligamentization and osseointegration processes of the polyethylene terephthalate artificial ligament in vitro and in vivo. Journal of Materials Chemistry B 6 (36):5738–49. doi:10.1039/c8tb01310a.
- Caruso, A. B., and M. G. Dunn. 2005. Changes in mechanical properties and cellularity during long-term culture of collagen fiber ACL reconstruction scaffolds. Journal of Biomedical Materials Research: Part A 73A (4):388–97. doi:10.1002/jbm.a.30233.
- Chalmers, P. N., N. A. Mall, M. Moric, S. L. Sherman, G. P. Paletta, B. J. Cole, and B. R. Bach Jr. 2014. Does ACL reconstruction alter natural history?: A systematic literature review of long-term outcomes. The Journal of Bone & Joint Surgery 96 (4):292–300. doi:10.2106/JBJS.L.01713.
- Chen, J., Q. Mo, R. Sheng, A. Zhu, C. Ling, Y. Luo, A. Zhang, Z. Chen, Q. Yao, Z. Cai, et al. 2021. The application of human periodontal ligament stem cells and biomimetic silk scaffold for in situ tendon regeneration. Stem Cell Research & Therapy 12 (1):596. doi:10.1186/s13287-021-02661-7.
- Dominici, M., K. Le Blanc, I. Mueller, I. Slaper-Cortenbach, F. Marini, D. Krause, R. Deans, A. Keating, D. Prockop, and E. Horwitz. 2006. Minimal criteria for defining multipotent mesenchymal stromal cells. The International Society for Cellular Therapy position statement. Cytotherapy 8 (4):315–17. doi:10.1080/14653240600855905.
- Dong, Q., J. Cai, H. Wang, S. Chen, Y. Liu, J. Yao, Z. Shao, and X. Chen. 2020. Artificial ligament made from silk protein/Laponite hybrid fibers. Acta biomaterialia 106 (106):102–13. doi:10.1016/j.actbio.2020.01.045.
- Dunn, M. G., J. B. Liesch, M. L. Tiku, and J. P. Zawadsky. 1995. Development of fibroblast-seeded ligament analogs for ACL reconstruction. Journal of Biomedical Materials Research 29 (11):1363–71. doi:10.1002/jbm.820291107.
- Dustmann, M., T. Schmidt, I. Gangey, F. N. Unterhauser, A. Weiler, and S. U. Scheffler. 2008. The extracellular remodeling of free-soft-tissue autografts and allografts for reconstruction of the anterior cruciate ligament: A comparison study in a sheep model. Knee Surgery, Sports Traumatology, Arthroscopy 16 (4):360–69. doi:10.1007/s00167-007-0471-0.
- Embree, M. C., M. Chen, S. Pylawka, D. Kong, G. M. Iwaoka, I. Kalajzic, H. Yao, C. Shi, D. Sun, T. J. Sheu, et al. 2016. Exploiting endogenous fibrocartilage stem cells to regenerate cartilage and repair joint injury. Nature communications 7 (1):13073. doi:10.1038/ncomms13073.
- Fazal, N., and N. Latief. 2018. Bombyx mori derived scaffolds and their use in cartilage regeneration: A systematic review. Osteoarthritis and Cartilage 26 (12):1583–94. doi:10.1016/j.joca.2018.07.009.
- Ficek, K., J. Rajca, M. Stolarz, E. Stodolak-Zych, J. Wieczorek, M. Muzalewska, M. Wyleżoł, Z. Wróbel, M. Binkowski, and S. Błażewicz. 2019. Bioresorbable Stent in Anterior Cruciate Ligament Reconstruction. Polymers (Basel) 11 (12):1961. doi:10.3390/polym11121961.
- Frank, C. B., and D. W. Jackson. 1997. Current Concepts Review - the Science of Reconstruction of the Anterior Cruciate Ligament. The Journal of Bone & Joint Surgery 79 (10):1556–76. doi:10.2106/00004623-199710000-00014.
- Greenwald, D., S. Shumway, P. Albear, and L. Gottlieb. 1994. Mechanical comparison of 10 suture materials before and after in vivo incubation. The Journal of Surgical Research 56 (4):372–77. doi:10.1006/jsre.1994.1058.
- Han, F., P. Zhang, T. Chen, C. Lin, X. Wen, and P. Zhao. 2019. A LbL-Assembled Bioactive Coating Modified Nanofibrous Membrane for Rapid Tendon-Bone Healing in ACL Reconstruction. International Journal of Nanomedicine 14 (14):9159–72. doi:10.2147/IJN.S214359.
- Herzog, M. M., S. W. Marshall, J. L. Lund, V. Pate, C. D. Mack, and J. T. Spang. 2018. Trends in Incidence of ACL Reconstruction and Concomitant Procedures Among Commercially Insured Individuals in the United States, 2002-2014. Sports Health 10 (6):523–31. doi:10.1177/1941738118803616.
- Holland, C., K. Numata, J. Rnjak-Kovacina, and F. P. Seib. 2019. The Biomedical Use of Silk: Past, Present, Future. Advanced Healthcare Materials 8 (1):e1800465. doi:10.1002/adhm.201800465.
- Hou, Z., S. Meyer, N. E. Propson, J. Nie, P. Jiang, R. Stewart, and J. A. Thomson. 2015. Characterization and target identification of a DNA aptamer that labels pluripotent stem cells. Cell Research 25 (3):390–93. doi:10.1038/cr.2015.7.
- Hunt, P., S. U. Scheffler, F. N. Unterhauser, and A. Weiler. 2005. A model of soft-tissue graft anterior cruciate ligament reconstruction in sheep. Archives of Orthopaedic and Trauma Surgery 125 (4):238–48. doi:10.1007/s00402-004-0643-z.
- Hu, Y., J. Ran, Z. Zheng, Z. Jin, X. Chen, Z. Yin, C. Tang, Y. Chen, J. Huang, H. Le, et al. 2018. Exogenous stromal derived factor-1 releasing silk scaffold combined with intra-articular injection of progenitor cells promotes bone-ligament-bone regeneration. Acta biomaterialia 71 (71):168–83. doi:10.1016/j.actbio.2018.02.019.
- Hu, X., Y. Wang, Y. Tan, J. Wang, H. Liu, Y. Wang, S. Yang, M. Shi, S. Zhao, Y. Zhang, et al. 2017. A Difunctional Regeneration Scaffold for Knee Repair based on Aptamer-Directed Cell Recruitment. Advanced Materials 29 (15):1605235. doi:10.1002/adma.201605235.
- Koob, T. J., T. A. Willis, Y. S. Qiu, and D. J. Hernandez. 2001. Biocompatibility of NDGA-polymerized collagen fibers. II. Attachment, proliferation, and migration of tendon fibroblasts in vitro. Journal of Biomedical Materials Research: An Official Journal of the Society for Biomaterials, the Japanese Society for Biomaterials, and the Australian Society for Biomaterials and the Korean Society for Biomaterials 56 (1):40–48. doi:10.1002/1097-4636(200107)56:1<40:aid-jbm1066>3.0.co;2-i.
- Kurosaki, S., H. Otsuka, M. Kunitomo, M. Koyama, R. Pawankar, and K. Matumoto. 1999. Fibroin allergy. IgE mediated hypersensitivity to silk suture materials. Nihon Ika Daigaku zasshi 66 (1):41–44. doi:10.1272/jnms.66.41.
- Laitinen, O., T. Pohjonen, P. Tormala, K. Saarelainen, J. Vasenius, P. Rokkanen, and S. Vainionpaa. 1993. Mechanical properties of biodegradable poly-L-lactide ligament augmentation device in experimental anterior cruciate ligament reconstruction. Archives of Orthopaedic and Trauma Surgery 112 (6):270–74. doi:10.1007/BF00452963.
- Leong, N. L., F. A. Petrigliano, and D. R. McAllister. 2014. Current tissue engineering strategies in anterior cruciate ligament reconstruction. Journal of Biomedical Materials Research: Part A 102 (5):1614–24. doi:10.1002/jbm.a.34820.
- Li, M., M. Ogiso, and N. Minoura. 2003. Enzymatic degradation behavior of porous silk fibroin sheets. Biomaterials 24 (2):357–65. doi:10.1016/s0142-9612(02)00326-5.
- Li, H., Z. Yao, J. Jiang, Y. Hua, J. Chen, Y. Li, K. Gao, and S. Chen. 2012. Biologic failure of a ligament advanced reinforcement system artificial ligament in anterior cruciate ligament reconstruction: A report of serious knee synovitis. Arthroscopy 28 (4):583–86. doi:10.1016/j.arthro.2011.12.008.
- Mayr, H. O., A. Stoehr, M. Dietrich, R. von Eisenhart-Rothe, R. Hube, S. Senger, N. P. Suedkamp, and A. Bernstein. 2012. Graft-dependent differences in the ligamentization process of anterior cruciate ligament grafts in a sheep trial. Knee Surgery, Sports Traumatology, Arthroscopy 20 (5):947–56. doi:10.1007/s00167-011-1678-7.
- Nau, T. 2015. Regeneration of the anterior cruciate ligament: Current strategies in tissue engineering. World Journal of Orthopedics 6 (1):127–36. doi:10.5312/wjo.v6.i1.127.
- Ribeiro, V. P., J. B. Costa, and S. M. Carneiro, S. Pina, A. C. A. Veloso, R. L. Reis, J. M. Oliveira. 2022. Bioinspired Silk Fibroin-Based Composite Grafts as Bone Tunnel Fillers for Anterior Cruciate Ligament Reconstruction. Pharmaceutics 14 (4):697. doi:10.3390/pharmaceutics14040697.
- Sahoo, S., S. L. Toh, and J. C. Goh. 2010. A bFGF-releasing silk/plga-based biohybrid scaffold for ligament/tendon tissue engineering using mesenchymal progenitor cells. Biomaterials 31 (11):2990–98. doi:10.1016/j.biomaterials.2010.01.004.
- Salthouse, T. N., B. F. Matlaga, and M. H. Wykoff. 1977. Comparative tissue response to six suture materials in rabbit cornea, sclera, and ocular muscle. American Journal of Ophthalmology 84 (2):224–33. doi:10.1016/0002-9394(77)90856-x.
- Schindler, O. S. 2012. Surgery for anterior cruciate ligament deficiency: A historical perspective. Knee Surgery, Sports Traumatology, Arthroscopy 20 (1):5–47. doi:10.1007/s00167-011-1756-x.
- Soong, H. K., and K. R. Kenyon. 1984. Adverse reactions to virgin silk sutures in cataract surgery. Ophthalmology 91 (5):479–83. doi:10.1016/s0161-6420(84)34273-7.
- Teuschl, A., P. Heimel, S. Nurnberger, M. van Griensven, H. Redl, and T. Nau. 2016. A Novel Silk Fiber–Based Scaffold for Regeneration of the Anterior Cruciate Ligament. The American Journal of Sports Medicine 44 (6):1547–57. doi:10.1177/0363546516631954.
- Thurber, A. E., F. G. Omenetto, and D. L. Kaplan. 2015. In vivo bioresponses to silk proteins. Biomaterials 71 (71):145–57. doi:10.1016/j.biomaterials.2015.08.039.
- Valluzzi, R., S. Winkler, D. Wilson, D. L. Kaplan, A. J. Bailey, J. Macmillan, P. R. Shrewry, and A. S. Tatham. 2002. Silk: Molecular organization and control of assembly. Philosophical Transactions of the Royal Society of London: Series B, Biological Sciences no. 357 (1418):165–67. doi:10.1098/rstb.2001.1032.
- Vavken, P., and M. M. Murray. 2010. Translational studies in anterior cruciate ligament repair. Tissue Engineering: Part B, Reviews 16 (1):5–11. doi:10.1089/ten.teb.2009.0147.
- Wang, X., X. Song, T. Li, J. Chen, G. Cheng, L. Yang, and C. Chen. 2019. Aptamer-Functionalized Bioscaffold Enhances Cartilage Repair by Improving Stem Cell Recruitment in Osteochondral Defects of Rabbit Knees. The American Journal of Sports Medicine 47 (10):2316–26. doi:10.1177/0363546519856355.
- Weiler, A., R. F. G. Hoffmann, H. J. Bail, O. Rehm, and N. P. Sudkamp. 2002. Tendon healing in a bone tunnel. Part II: Histologic analysis after biodegradable interference fit fixation in a model of anterior cruciate ligament reconstruction in sheep. Arthroscopy: The Journal of Arthroscopic & Related Surgery 18 (2):124–35. doi:10.1053/jars.2002.30657.
- Yodmuang, S., S. L. McNamara, A. B. Nover, B. B. Mandal, M. Agarwal, T.A.N. Kelly, P.H.G. Chao, C. Hung, D. L. Kaplan, and G. Vunjak-Novakovic. 2015. Silk microfiber-reinforced silk hydrogel composites for functional cartilage tissue repair. Acta biomaterialia 11 (11):27–36. doi:10.1016/j.actbio.2014.09.032.
- Zhang, W., L. Chen, J. Chen, L. Wang, X. Gui, J. Ran, G. Xu, H. Zhao, M. Zeng, J. Ji, et al. 2017. Silk Fibroin Biomaterial Shows Safe and Effective Wound Healing in Animal Models and a Randomized Controlled Clinical Trial. Advanced Healthcare Materials 6 (10):1700121. doi:10.1002/adhm.201700121.
- Zhu, G., and X. Chen. 2018. Aptamer-based targeted therapy. Advanced Drug Delivery Reviews 134 (134):65–78. doi:10.1016/j.addr.2018.08.005.