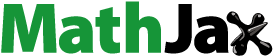
ABSTRACT
Bamboo leaves are a resource of cellulose fibers that can be further developed into value-added products. In this research, cellulose nanofibers were obtained from Tinwa bamboo leaves, and then chemically modified with (i) hexadecyltrimethoxysilane (HDTMS) and (ii) perfluorooctyltriethoxysilane (POTS). The modified nanofibers were then used as a hydrophobic biopolymer coating on paper, as an alternative to commonly used fossil-based coatings. Hydrophobicity in terms of surface and bulk properties of the coated paperboards was investigated. The results showed that the hydrophobic coatings obtained provided a high contact angle up to 141° and a decrease of water penetration speed during immersion up to 94% when using ultrasonic technique. The coated paperboards exhibited color difference values compared with an uncoated paperboard in the range of 0.4 to <2 which is hardly distinguishable from the human eye. These results suggest that a biopolymer obtained from modified bamboo leaves does in fact provide a suitable replacement for hydrophobic coating on paper.
摘要
竹叶是纤维素纤维的一种资源,可以进一步开发成增值产品. 在这项研究中,从廷瓦竹叶获得纤维素纳米纤维,然后用(i)十六烷基三甲氧基硅烷(HDTMS)和(ii)全氟辛基三乙氧基硅(POTS)进行化学改性. 然后,改性纳米纤维被用作纸张上的疏水生物聚合物涂层,作为常用化石涂层的替代品. 研究了涂布纸板表面和体积财产的疏水性. 结果表明,当使用超声技术时,获得的疏水涂层提供了高达141o的高接触角,并且在浸没期间水渗透速度降低了94%. 与未涂布纸板相比,涂布纸板的色差值在0.4至<2的范围内. 这些结果表明,从改性竹叶中获得的生物聚合物事实上确实为纸张上的疏水涂层提供了合适的替代品.
Introduction
Bamboo is an industrial crop with a high economic impact in many countries. According to data provided by cultivators, bamboo leaves are typically perceived as agricultural waste. There are plenty of fallen bamboo leaves that accumulate on the ground every day and most of them are only used as fertilizer. Applications of bamboo leaves have not been widely developed even though there are plenty of organic substances and minerals in those leaves. According to our literature review, there has been only limited research to utilize bamboo leaves for producing new products. For example, Yuan et al. (Citation2021) deposited copper-aluminum composite oxide on a carbon precursor prepared from bamboo leaf fibers for the adsorption of ammonia nitrogen in wastewater. This carbon precursor provided good stability as a carrier of the oxide layer, leading to a higher content of ammonia nitrogen adsorption from sewage when compared with commercially activated carbon (Yuan et al. Citation2021). Olawale (Citation2020) extracted silica from bamboo leaves as a raw material for ceramic production, in which amorphous nanosilica with a spherical shape and high specific area (915.33 m2/g BET) was found under a suitable calcination process of the bamboo leaves (Olawale Citation2020). Other researchers extracted bamboo leaves to acquire bioactive and antimicrobial compounds used in food, personal care, and the pharmaceutical industries. Water-soluble polysaccharides extracted from bamboo (Phyllostachys pubescens Mazel) leaves exhibited high antioxidant activity and also had antimicrobial activity against E. coli, S. aureus, and B. subtilis (Xiao et al. Citation2020). The antioxidants mostly found in bamboo leaves were phenol compounds, selenium, zinc, copper, manganese, iron, vitamin C, and vitamin E (Nirmala et al. Citation2018). In addition to the above-mentioned substances, bamboo leaves are a resource of cellulose, a kind of biopolymer, which can be further developed into products that require cellulose as a raw material, such as paper, textile, and biodegradable plastics. Therefore, exploring cellulose from bamboo leaves for a novel product, such as surface coatings, is worth of consideration.
Nanocellulose is a raw material extracted from plants that has been widely studied. Nanofibrillated cellulose (NFC) or cellulose nanofibers (CNF) are a kind of nanocellulose presenting a long-fiber form. CNF has many strong points matching the requirements of polymers for coatings (Milanovic et al. Citation2020), such as film-forming ability, good wetting on substrates, high thermal stability, and printability. However, it has a hydrophilic behavior, mainly due to the presence of hydroxyl groups (−OH) in its chemical structure. This can be improved using a variety of chemical modifications, such as silane treatment that works via covalent bonding at the positions of those hydroxyl groups (Vijay et al. Citation2021). For example, cellulosic materials modified with a silane containing a long alkyl chain, dodecyltriethoxysilane, and silica nanoparticles presented a water contact angle up to 137° (Le et al. Citation2017) and up to 151° when using hexadecyltrimethoxysilane together with tetraethylorthosilicate (Ahuja et al. Citation2021). A similar result was found when using 1 H,1 H,2 H,2 H-perfluorooctyltriethoxysilane on paper, where the water contact angle was improved up to 146° (Yu et al. Citation2019). These results of improved water contact angle were attributed to the decreased surface energy of cellulosic materials (Wei et al. Citation2020).
Nowadays, paper plays an essential role in producing biodegradable packaging. Several techniques have been implemented to improve hydrophobicity during paper production, e.g., internal sizing, surface sizing, paper coating, and barrier coating on the finished paper (Rastogi and Samyn Citation2015; Sakare et al. Citation2021). Our study aims to develop CNF from bamboo leaves as a hydrophobic biopolymer coating for paper. Firstly, we studied the cellulose content in bamboo leaves from five available varieties in Thailand and the variety with the highest cellulose content was selected as the prime candidate for the preparation of CNF. Secondly, the CNF obtained was chemically modified to improve its hydrophobic properties and then applied on paperboard. Then, characterizations and property tests were performed to evaluate the application as a hydrophobic coating. An illustration of the overall study is shown in .
Materials and Methods
Materials
Bamboo leaves were supplied by a local bamboo farm located in the northeastern part of Thailand. Siam Kraft Industry Co., Ltd. (Thailand) provided the white back duplex paperboard that was used as a substrate in this study. Sodium hydroxide (NaOH, 99.9% purity) and glacial acetic acid (CH3COOH, 99% purity) were supplied from QReC Chemical Co., Ltd. (Auckland, New Zealand). Sodium chlorite (NaClO2, 80% purity) was purchased from Loba Chemie Pvt., Ltd. (Mumbai, India). Hexadecyltrimethoxysilane (HDTMS) and 1 H,1 H,2 H,2 H-perfluorooctyltriethoxysilane (POTS) were supplied by BRB International b.v. (Ittervoort, the Netherlands) and Tokyo Chemical Industry Co., Ltd. (Tokyo, Japan), respectively. All chemicals were used directly as received.
Methods
Purification of bamboo leaf pulp
Dried bamboo leaves were ground and treated with 6%w/v NaOH solution (solid: liquid ratio, 1:12) at 80°C for 3 h. This treatment was performed three times before passing to a bleaching step. To bleach the pulp, it was boiled with 1.8%w/v NaClO2 solution (solid: liquid ratio, 1:12) at 85°C for 3 h in which diluted acetic acid was added to adjust the pH to 3.5–4 for bleaching efficacy. The bleaching process was performed three times to obtain a high whiteness of the pulp.
Preparation of bamboo leaf nanofibers
The never-dried pulp was carefully diluted with water to a concentration of 0.5%w/v solid content and then mechanically stirred in a high-speed blender (BUO −121280, Buono, Taiwan) at 38,000 rpm for 8 min. The suspension obtained was further defibrillated through a high-pressure homogenizer (LM20 Microfluidizer, Microfluidics, Westwood, MA) equipped with a Z-type interaction chamber (87 µm channel). The defibrillation condition was conducted at an operating pressure of 1,380 bar and a cooling temperature of 20°C. In this process, 200 mL of cellulose slurry was continuously circulated at a fixed defibrillation time of 60 min. After that, a well-dispersed aqueous suspension containing 0.5%w/v of bamboo leaf nanofibers (BLN) was stored in a refrigerator (8–10°C) for further use.
Hydrophobic modification of BLN suspension
Two silane solutions were prepared prior to modification of BLN by a silylation reaction: 5 wt% HDTMS was dissolved in absolute ethanol, and 5 wt% POTS was dissolved in a mixed solvent (95:5 v/v of water:ethanol). Before silylation, the pH of the BLN suspension was adjusted to 4.5 by diluted acetic acid because an acidic pH can catalyze the hydrolysis reaction of the silanes. Silylation was then conducted by dropwise method in which the silane solution was dropped into the acidic BLN under continuous stirring and then kept stirring for 3 h at 25 ± 1°C. After that, the mixture was aged for 18 h to promote the kinetic of the silylation. In this research, different silane concentrations, in the range of 0.2–1.5 wt% based on the BLN suspension weight, were studied. The abbreviations of each silane-modified sample were HD1–HD4 and PO1–PO4 representing the BLN suspension modified with HDTMS and POTS at 0.2 (HD1 and PO1), 0.5 (HD2 and PO2), 1 (HD3 and PO3), and 1.5 (HD4 and PO4) wt% based on the suspension weight, respectively.
Coating on paperboard
The modified-BLN suspension was centrifuged at 5,000 rpm for 45 min to remove water and obtain a concentrated paste (2%w/v solid content of BLN). This paste was used to coat a substrate of sized paper without pigment coating (i.e., the backside of the white back duplex paperboard). A four-sided applicator with 300 μm of wet film thickness was employed for coating so that the coat weight could be calculated from the applied volume – approx. 6 g/m2 due to 2%w/v solid content of BLN. The coating film was dried by a hair crimper at 140°C to simulate the drying process under restraint used in industrial production that reduces the shrinkage of dried paper. After that, the coated paperboard was further cured at 120°C in an oven for 1 h. Figure S1 (see Supplemental materials) shows photographs of uncoated paperboard and coated paperboard with HD-3 and PO-3.
Characterizations of bamboo leaf pulp and BLN coating film
Chemical composition analysis. The chemical compositions of bamboo leaves from different varieties in Thailand were determined by a method proposed by Goering and Van Soest in 1970 (Goering and Van Soest Citation1970). Three replicates were evaluated in each variety.
Morphology. The suspension samples were carefully dropped onto microscope glass slides and dried in a desiccator. They were sputter-coated with gold and then investigated by a scanning electron microscope, SEM (JSM-IT500HR, JEOL, Peabody, MA), with 1.0 kV accelerating voltage.
Crystallinity index by X-ray diffraction technique (XRD). XRD (Bruker, AXS-D8, Billerica, MA) under Cu Kα radiation with a wavelength of 0.154 nm at 40 kV and 40 mA was applied. The samples in powder form were prepared and properly filled into sample holders. The scanning angle (2θ) was ranged from 5° to 40° with a step size of 0.005° 2θ and a scan speed of 1 sec per step. The crystallinity indices were analyzed using the Rietveld refinement method with Topas Academic v. 2.1 software, and the percentages of the amorphous and crystalline structures were calculated from the area-based fitting method (French Citation2020).
Chemical structure. Fourier transform infrared spectroscopy (FTIR) with an integrated iD7 ATR accessory (Nicolet iS5, Thermo Scientific Waltham, MA, USA) at 64 scans with a spectra resolution of 8 cm−1 was used to analyze functional groups of samples. The samples of BLN coated film were obtained from the coated paperboards, where the coated side was tested.
Property tests of uncoated and coated paperboards
Hydrophobic property and surface energy by contact angle. The sessile drop method with 3 µl droplet of distilled water from a contact angle meter (OCA-20, DataPhysics Instruments, Filderstadt, Germany) was applied following ASTM D7334–08. A contact angle was read at 10 sec after contacting the sample surface. At least ten replicates were measured in each sample, and an average contact angle value was reported based on five selected values.
In addition, the contact angles of diiodomethane, N,N-dimethylformamide, and ethylene glycol were measured using the same method in order to determine surface energies by I. Fowkes model (Fowkes Citation1963) and II. Owens – Wendt model (sometimes referred to as the OWRK model) (Kaelble and Cirlin Citation1971; Owens and Wendt Citation1969; Rabel Citation1971). The Fowkes model dissociates a liquid surface tension or solid surface energy into its polar and dispersive (nonpolar) components in which the primary equation is as follows:
where is the surface tension of a liquid.
,
,
, and
are the liquid dispersive, liquid polar, solid dispersive, and solid polar components, respectively. The average contact angles (
) of water and diiodomethane in the solid sample were used for the calculation of
and
using a system of two equations. The total surface energy of the solid sample is the sum of
and
as shown in Eq. (2):
For Owens – Wendt model (extended Fowkes model), the surface energy is determined by plotting a linear line () of data points obtained from the average contact angles of different liquids following Eq. 3:
where-axis is
and
-axis is
. A slope (
) and
-intercept (
) allow for the determination of the total surface energy of the solid sample using Eq. 2.
In our work, four different liquids, i.e., water, diiodomethane, N,N-dimethylformamide, and ethylene glycol were used for the calculation of the data points. The determination methods of these two models are shown in the supplemental materials (Table S1-S3).
Surface roughness by atomic force microscopy (AFM). AFM (XE-120, Park Systems, Korea) was used under a non-contact mode using an ACTA probe with a performed area of 5 × 5 µm2 at a scan rate of 0.25 Hz and a resonance frequency at 350 kHz. Three replicates were measured at different positions on each specimen.
Penetration dynamic analysis (PDA) of water. A PDA instrument (PDA.C02 MST, Emtec, Germany) was employed under 2 MHz frequency, and a sensor area of 35-mm diameter. The duration of water immersion with ultrasonic measurement was 120 s. Three replicates were done in each sample, and the average value was then recorded.
CIE L*a*b* color difference. The colorimetric parameters of the uncoated and coated paperboards were analyzed by a portable spectrophotometer (CM-700d, Konica Minolta, Japan) at a standard illuminant D65. The average value of each parameter was obtained from at least four measurements.
Results and Discussion
Chemical composition analysis of raw bamboo leaves from different varieties
According to the chemical composition of raw bamboo leaves from seven different varieties, as summarized in , it can be seen that two varieties exhibited higher cellulose content than others: (i) Phyllostachys edulis (Carrière) J. Houz. (common name: Moso bamboo) and (ii) Schizostachyum pergracile (Munro) R. B. Majumdar (common name: Tinwa bamboo). Their cellulose contents were 29.49 and 29.87%w/w, respectively. According to our survey of bamboo cultivation in Thailand, Tinwa bamboo is widely cultivated and provides a much higher productivity than Moso bamboo. Therefore, the bamboo leaves from Tinwa bamboo were selected for this study. Tinwa bamboo leaves were chemically purified by removing hemicellulose, lignin, and other extractives. After purification, the cellulose content of Tinwa bamboo pulp was increased from 29.87 to 77.8%w/w. Meanwhile, the amount of hemicellulose, lignin, and other extractives was significantly decreased (see and Figure S2). This purified pulp was later transformed into CNF using mechanical defibrillation.
Table 1. Chemical composition of bamboo leaves from different varieties.
Morphology of BLNs
The purification process could transform the shape of ground bamboo leaves from non-uniform chips to fibers with diameters in the range of 5–10 µm and an aspect ratio >100. Moreover, the fibers’ surfaces became much smoother than the chips’ surfaces since non-cellulosic constituents, i.e., lignin, hemicellulose, and other extractives, were mainly removed during the alkaline delignification and bleaching processes (Figure S3 in Supplemental materials).
shows SEM micrographs of BLN before and after modification. Our results suggest that unmodified BLN exhibits web-like networks, with open spaces arising from intermolecular hydrogen bonding. The width is approximately 10–80 nm, and the length is up to several micrometers, indicating maintaining a high aspect ratio of the fiber shape. The shape obtained was due to our preparation methods such that the defibrillation of fibers occurred preferentially along the longitudinal direction by shear and impact forces. After surface modification, both HDTMS and POTS modified BLN displayed a highly dense and co-continuous structure having thick-coated layers of the spent silanes, thus confirming the achievement of surface modification via silylation. Interestingly, the coated layers were responsible for hydrophobicity, resulting in a hydrophobized surface on the paperboard.
Crystallinity index (CI) of raw bamboo leaves and BLNs
shows XRD patterns of raw bamboo leaves, the unmodified BLN, the BLN modified with HDTMS (HD-3) and the BLN modified with POTS (PO-3). After peak deconvolution, the results show that all sample types visibly display the main peaks around 15ο, 16ο, 22ο, and 35ο 2θ. These peaks represent (1–10), (110), (200), and (004) crystallographic planes, respectively, of an Iβ crystal structure in cellulose type I. They are evidence of the preservation of the crystalline structure of cellulose after surface modification by silylation. In addition, the HD-3 sample also displays two new main peaks at 21.4ο and 6.5ο 2θ which represent the lateral crystallization from the attachment of long alkyl chains onto cellulose chains and the increase in interlayer space between cellulose chains formed by the intercalation of long alkyl chains in the HDTMS structure, respectively (Guo et al. Citation2017; Jiang and Yi Citation2020). Based on the determined CI ( and Table S4 in Supplemental materials), the CI values of both HD-3 and PO-3 decreased to 39.3% and 46.9%, respectively. The decrease of CI implies a reduction of intermolecular orientation due to the steric hindrance of hexadecyl and perfluorooctyl side-chains in HD-3 and PO-3, especially in HD-3 where long alkyl chains provide a significant increase in amorphous structure. These side-chains link with anhydroglucose units of cellulose via the silylation reaction, resulting in increased free volume and molecular mobility and leading to less order of intermolecular chain packing.
Chemical structure of unmodified and modified BLNs
The FTIR spectra of all samples () show three prominent peaks located at 3320, 2880, and 1020 cm−1, corresponding to molecular vibrations of O-H stretching, C-H stretching, and C-O-C stretching of anhydroglucose units, respectively. These results help in understanding the remaining structure of cellulose type I after silylation. There is a minor peak at 1630 cm−1, representing O-H bending. In BLN samples, this peak was more notable compared to uncoated paperboards due to the significant increase in surface area containing hydroxyl groups after transformation into CNF. HD-3 and HD-4 samples () display new doublet peaks at 2915 and 2850 cm−1 and a minor peak at 1470 cm−1, arising mainly from C-H stretching and scissoring of hexadecyl chain (-C16H33) in HDTMS. In addition, minor peaks at 1110 and 713 cm−1 appeared in PO-3 and PO-4 samples that are associated with C-F3 and C-F bending, respectively, of perfluorooctyl group in POTS (Wu et al. Citation2018). When considering evidence of silylation, the change of peaks in the range of 1200–750 cm−1 would be typically focused due to the representatives of Si-O-C and Si-O-Si bonds, although they overlap with other peaks. The BLN modified with HDTMS and POTS also demonstrated three characteristic changes that were noted in previous works (Cabrera et al. Citation2020; Gogoi and Tyagi Citation2021; Pacaphol and Aht-Ong Citation2017). The first was a minor change at the shoulder of the peak at 1200–1100 cm−1 (symmetric stretching of Si-O-C). The second was a peak overlapping with the blunt peak of C-O in the anhydroglucose units at 1030 cm−1 (symmetric stretching of Si-O-Si) tand the transformation to a sharp end peak was noticed after silylation. The last was a new minor peak at 860 cm−1 (Si-O bending) associated with siloxane bonds (see . Our observations indicate that the silylation of CNF was achieved after modification with HDTMS and POTS, where a new covalent bond of Si-O-C is an evidence of condensation reaction between -Si-OH in silanes and -C-OH in the anhydroglucose ring of cellulose. In addition, a new siloxane bond (Si-O-Si) indicated self-crosslinking between silane molecules, which was also found on the surface of silylated samples.
Water contact angle and surface energy of uncoated and coated paperboards
Water contact angle (WCA) images and values are summarized in . The uncoated paperboard showed a moderate hydrophilic surface with WCA at 74.3° ± 2.4, which is notably higher than conventional cellulosic materials, due to the sizing process during its paper production (Rastogi and Samyn Citation2015). The WCA of the unmodified-BLN coated paperboard displayed a low angle at 54.7° ± 2.7, which is likely due to hydrophilic behavior from the abundant hydroxyl groups of CNF. After silylation, the WCAs of the BLN coated paperboards increased sharply (), especially the WCAs of HD-3 and PO-3 samples that displayed angles as high as 140.8° ± 1.6 and 140.1° ± 1.6, respectively. These WCA increases were mainly due to the long alkyl chain in HDTMS and the fluoroalkyl chain in POTS, which are typically responsible for hydrophobic behavior. Both long alkyl and fluoroalkyl chains have low polarity. After silylation, hydroxyl groups in CNF were replaced by long alkyl or fluoroalkyl chain, the surface free energy of CNF was reduced, and CNF also lost the ability of hydrogen bond creation between its hydroxyl groups and water, leading to hydrophobicity on the modified CNF surface.
Figure 5. Water contact angle images of uncoated paperboard (a), coated paperboards with unmodified BLN (b) and BLN modified with HD-1 (c), HD-2 (d), HD-3 (e), HD-4 (f), PO-1 (g), PO-2 (h), PO-3 (i), and PO-4 (j).
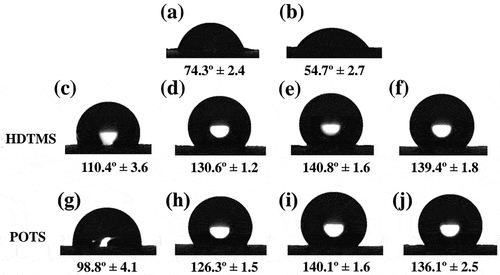
According to the contact angle theory of Wenzel (Wenzel Citation1949), surface hydrophobicity can be enhanced by (i) decreasing the surface energy and (ii) raising the surface roughness of the hydrophobic part, especially at the microscopic scale. Based on the results in (data for the surface energy calculation displayed in Table S1-S3), two models of the surface energy determination were used to estimate a trend of surface energy changes because our results contain a wide range of surface characteristics attributing to before and after silylation. Therefore, one model may not fit to represent all the results. The high surface energies of the uncoated paperboard (43.36 mJ.m−2 by Fowkes and 40.8 mJ.m−2 by Owens – Wendt) and the one coated with unmodified BLN (48.11 mJ.m−2 by Fowkes and 45.01 mJ.m−2 by Owens – Wendt) were found, indicating a highly polar surface. In contrast, the surface energies of paperboard coated with modified BLN are lower, indicating reduced polarity. Even at the lowest concentrations of HDTMS and POTS (HD-1 and PO-1), the samples show a decreasing number of surface energies by 50% and 66% compared to the uncoated sample, respectively (values based on Fowkes model since the coefficients of determination (R2) obtained from Owens-Wendt model in were too low in these two samples). The modification reduced the polarity by reducing the amount of hydroxyl groups. The surface energies tend to decrease with increasing concentration of HDTMS and POTS, where the optimal concentration can be seen in the HD-3 (20.12 mJ.m−2 by Fowkes and 22.71 mJ.m−2 by Owens – Wendt) and PO-4 (10.78 mJ.m−2 by Fowkes and 15.94 mJ.m−2 by Owens – Wendt) samples. Moreover, we found low values of R2 in the coated samples with high roughness (), and most of them are the samples modified with POTS. This result is in agreement with the previous work (Hejda, Solar, and Kousal Citation2010) where the higher roughness of the sample surface caused a loss of the linear trend or a low value of R2 when using Owens – Wendt model.
Figure 6. AFM images and surface roughness of uncoated paperboard (a,g), coated paperboards with unmodified BLN (b,h) and BLN modified with HD-3 (c,i), HD-4 (d,j), PO-3 (e, k), and PO-4 (f, l).
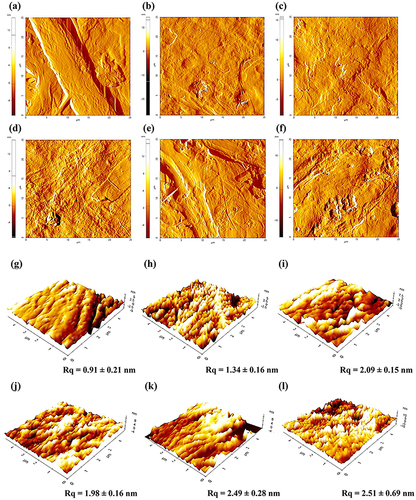
Table 2. Surface energy, water penetration speed, and color of uncoated and coated paperboards.
Surface roughness of uncoated and coated paperboards
According to the theory of Wenzel (Wenzel Citation1949) in the above-mentioned paragraph, an increase in the fraction of hydrophobic micro- or nanoscale roughness can significantly improve hydrophobicity on the surface. The root mean square roughness (Rq) of the uncoated and coated paperboards determined from surface topography images is summarized in . The unmodified-BLN coated paperboard has a rougher surface (Rq = 1.34 nm) than the uncoated paperboard due to the nanoscale layer coated by CNF. The silane-modified BLN coated paperboard shows further increases in Rq, with a higher concentration of silanes leading to higher Rq values, especially in HD-3 and PO-3 where Rq increases to 2.09 and 2.49 nm, respectively. The Rq increase is due to the nanoscale roughness of silylated molecules on the CNF surface. These changes of Rq agree well with the contact angles and surface energies described in previous sections.
Water penetration of uncoated and coated paperboards
Penetration dynamic analysis, or PDA, is a technique specifically used to interpret the paper–liquid interaction by recording the ultrasound intensity passing through the paper immersed in the liquid as a function of time. In , it can be seen that the ultrasound intensities of the uncoated and the unmodified-BLN coated paperboard quickly decreased from the first few seconds of the immersion time, and their intensities were close to zero within 10 sec. Conversely, the paperboards coated with HD-3 and PO-3 showed a gradual decrease in intensity and still maintained higher than 20% intensity even when the water immersion time had passed for 120 sec. These decreases in the intensity indicated the water penetration ability – the more water penetrated through the paperboard, the more intensity decreased. As water penetrated the fiber structure of the paperboard, air bubbles in the paperboard were compressed as the backside of the paperboard was closed by a sample holder. Those compressed air bubbles flowed back into the swollen structure of fibers and became trapped in the paper pores. The air bubbles resulted in the scattering of the ultrasound, thus decreasing the transmission intensity (see Figure S4 in Supplemental materials). Consequently, the results imply that the HD-3 and PO-3 samples exhibit a much higher hydrophobicity than the uncoated sample, and they also provided hydrophobicity in terms of the bulk property of the paperboard since the entire piece of the coated paperboard was immersed in a water bath for an extended period.
Figure 7. Water penetration curves under ultrasound transmission of uncoated and coated paperboards.
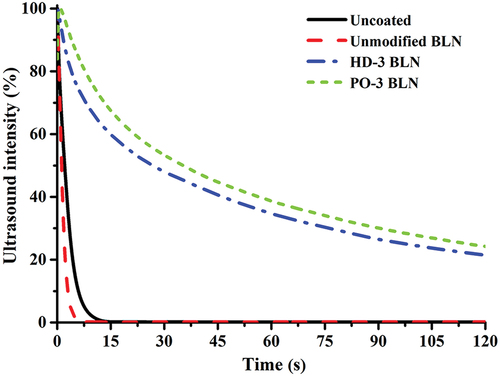
Additionally, the penetration speed of the water can be measured from the initial slope at the linear region of the ultrasound intensity–time curve (Sarah and Ulrich Citation2018). In , the uncoated paperboard had a penetration speed of 39.63 s−1, whereas the unmodified BLN showed a slight increase in penetration speed (42.52 s−1). This increase was due to the hydrophilic groups (−OH) on the enlarged surface area from the size reduction of cellulosic fibers to be CNF. After hydrophobic modification, the coated papers’ penetration speed decreased dramatically compared to the unmodified one. These results were attributed to the layer of hydrophobic BLN coating on the paperboard that effectively resists water-molecule penetration.
Color difference between uncoated and coated paperboards
Color is one of the most important factors that influences the appearance and quality of coated paper. shows the colorimetric parameters (L*, a*, b*) and total color difference (ΔE*) of the uncoated and coated paperboards. The results show that the lightness of all coated paperboards with modified BLN was equivalent to the uncoated paperboard; however, they were slightly greenish-yellowish compared with the uncoated one. When considering ΔE* values between the uncoated and coated paperboards, it was found that the BLN coatings did not noticeably influence the color of the paperboards. The paperboards coated with BLN exhibited ΔE* values in the range of 0.4 to <2 (Figure S1 and ); a color difference that the human naked eye can hardly distinguish according to the ΔE* study described in a previous article (Mokrzycki and Tatol Citation2011).
Conclusions
Hydrophobic biopolymer coating was successfully prepared from modified bamboo leaf nanofibers. Cellulose nanofibers (CNF) were produced from the leaves of Schizostachyum pergracile (Munro) R. B. Majumdar (common name: Tinwa bamboo), as its leaves provided higher cellulose than other varieties considered. The hydrophobic modification of CNF was achieved via a silylation reaction using two different silanes, one containing a long alkyl chain (HDTMS) and one containing a perfluoro functional group (POTS). The hydrophobic properties of the CNF coatings were proven by contact angle and water penetration under ultrasound transmission. It was observed that the contact angle increased from 74° on uncoated paperboard, to 141° and 140° on paperboards coated with HDTMS and POTS modified CNF, respectively. Additionally, their ultrasound transmission was maintained to be higher than 20% even when the water immersion was as long as 120 sec. The coated paperboards exhibited ΔE* values in the range of 0.4 to <2, which is hardly distinguishable to the human eye. Based on the results obtained, these findings show the feasibility of using silylated bamboo leaf nanofibers as a hydrophobic coating on paper.
Highlights
Cellulose nanofibers-based hydrophobic coating was successfully produced from bamboo leaves.
Both hydrophobic surface and bulk properties of the paperboard were improved by the coating.
Silylated nanofibers coating provided a high contact angle of up to 141° with reduced surface energy.
Ultrasound transmission is maintained higher than 20% at 120 s of water immersion of the coated paperboard.
Declaration
The authors confirm that this manuscript has not been published and submitted simultaneously elsewhere. All authors have given consent for the publication of identifiable details, which can include photographs and/or videos and/or case history and/or details within the text to be published in this journal (Journal of Natural Fibers).
Supplemental Material
Download Zip (2.1 MB)Acknowledgements
The authors appreciate the Department of Imaging and Printing Technology, the Department of Materials Science, and the Department of Chemistry, Faculty of Science, Chulalongkorn University, Bangkok, Thailand, for laboratory facilities and instruments. We gratefully acknowledge the assistance of Dr. Kraiwit Pakutsah for the pulp purification; Assoc. Prof. Dr. Pichayada Katemake for the advice of statistical analysis; and Asst. Prof. Dr. Supaporn Noppakundilograt for the advice of surface energy models. Finally, special thanks go to Dr. Darren Burford for proofreading and English editing.
Disclosure statement
The authors declare that there are no known competing financial interests or personal relationships that could have appeared to influence the work reported in this paper.
Supplementary material
Supplemental data for this article can be accessed online at https://doi.org/10.1080/15440478.2023.2178581
Additional information
Funding
References
- Ahuja, D., S. Dhiman, G. Rattan, S. Monga, S. Singhal, and A. Kaushik. 2021. Superhydrophobic modification of cellulose sponge fabricated from discarded jute bags for oil water separation. Journal of Environmental Chemical Engineering 9 (2):105063. doi:10.1016/j.jece.2021.105063.
- Cabrera, I. C., S. Berlioz, A. Fahs, G. Louarn, and P. Carriere. 2020. Chemical functionalization of nano fibrillated cellulose by glycidyl silane coupling agents: A grafted silane network characterization study. International Journal of Biological Macromolecules 165:1773–15. doi:10.1016/j.ijbiomac.2020.10.045.
- Fowkes, F. M. 1963. Additivity of intermolecular forces at interfaces. I. Determination of the contribution to surface and interfacial tensions of dispersion forces in various liquids. The Journal of Physical Chemistry 67 (12):2538–41. doi:10.1021/j100806a008.
- French, A. D. 2020. Increment in evolution of cellulose crystallinity analysis. Cellulose 27 (10):5445–48. doi:10.1007/s10570-020-03172-z.
- Goering, H. K., and P. J. Van Soest. 1970. Forage fiber analysis: Apparatus, reagents, procedures, and some applications. In USDA-ARS Agricultural Handbook 379. Washington DC: Agricultural Research Service, U.S. Dept. of Agriculture.
- Gogoi, R., and A. K. Tyagi. 2021. Surface modification of jute fabric by treating with silane coupling agent for reducing its moisture regain characteristics. Journal of Natural Fibers 18 (6):803–12. doi:10.1080/15440478.2019.1658252.
- Guo, J., W. Du, S. Wang, Y. Yin, and Y. Gao. 2017. Cellulose nanocrystals: A layered host candidate for fabricating intercalated nanocomposites. Carbohydrate Polymers 157:79–85. doi:10.1016/j.carbpol.2016.09.065.
- Hejda, F., P. Solar, and J. Kousal. 2010. Surface free energy determination by contact angle measurements – a comparison of various approaches. WDS’10 Proceedings of Contributed Papers, Part III 25–30.
- Jiang, W., and J. Yi. 2020. Surface modification of nanocrystalline cellulose and its application in natural rubber composites. L. Li, C. Wu, and J. Gu Journal of Applied Polymer Science 137(39):49163. doi:10.1002/app.49163.
- Kaelble, D. H., and E. H. Cirlin. 1971. Dispersion and polar contributions to surface tension of poly(methylene oxide) and Na-treated polytetrafluoroethylene. Journal of Polymer Science Part A-2: Polymer Physics 9 (2):363–68. doi:10.1002/pol.1971.160090210.
- Le, D., S. Kongparakul, C. Samart, P. Phanthong, S. Karnjanakom, A. Abudula, and G. Guan. 2017. One-pot fabrication of hydrophobic nanocellulose-silica film for water resistant packaging application. Journal of the Japan Institute of Energy 96 (8):261–65. doi:10.3775/jie.96.261.
- Milanovic, Ј., Т. Lazic, I. Zivkovic, М. Vuksanovic, M. Milosevic, and М. Kostic. 2020. The effect of nanofibrillated tempo-oxidized cotton linters on the strength and optical properties of paper. Journal of Natural Fibers 19 (11):3993–4006. doi:10.1080/15440478.2020.1848742.
- Mokrzycki, W., and M. Tatol. 2011. Color difference Delta E - a survey. Machine Graphics and Vision 20 (4):383–411.
- Nirmala, C., M. S. Bisht, H. K. Bajwa, and O. Santosh. 2018. Bamboo: A rich source of natural antioxidants and its applications in the food and pharmaceutical industry. Trends in Food Science & Technology 77:91–99. doi:10.1016/j.tifs.2018.05.003.
- Olawale, O. 2020. Bamboo leaves as an alternative source for silica in ceramics using box Benhken design. Scientific African 8:e00418. doi:10.1016/j.sciaf.2020.e00418.
- Owens, D. K., and R. C. Wendt. 1969. Estimation of the surface free energy of polymers. Journal of Applied Polymer Science 13 (8):1741–47. doi:10.1002/app.1969.070130815.
- Pacaphol, K., and D. Aht-Ong. 2017. The influences of silanes on interfacial adhesion and surface properties of nanocellulose film coating on glass and aluminum substrates. Surface & Coatings Technology 320:70–81. doi:10.1016/j.surfcoat.2017.01.111.
- Rabel, W. 1971. Einige Aspekte der benetzungstheorie und ihre anwendung auf die untersuchung und veränderung der oberflächeneigenschaften von polymeren. Farbe und Lack 77 (10):997–1005.
- Rastogi, V. K., and P. Samyn. 2015. Bio-based coatings for paper applications. Coatings 5 (4):887–930. doi:10.3390/coatings5040887.
- Sakare, P., A. K. Bharimalla, J. Dhakane-Lad, and P. G. Patil. 2021. Development of greaseproof paper from banana pseudostem fiber for packaging of butter. Journal of Natural Fibers 18 (12):1974–82. doi:10.1080/15440478.2019.1710652.
- Sarah, K., and H. Ulrich. 2018. Short timescale wetting and penetration on porous sheets measured with ultrasound, direct absorption and contact angle. RSC Advances 8 (23):12861–69. doi:10.1039/C8RA01434E.
- Vijay, R., S. Manoharan, S. Arjun, A. Vinod, and D. Lenin Singaravelu. 2021. Characterization of silane-treated and untreated natural fibers from stem of leucas aspera. Journal of Natural Fibers 18 (12):1957–73. doi:10.1080/15440478.2019.1710651.
- Wei, D. W., H. Wei, A. C. Gauthier, J. Song, Y. Jin, and H. Xiao. 2020. Superhydrophobic modification of cellulose and cotton textiles: Methodologies and applications. Journal of Bioresources and Bioproducts 5 (1):1–15. doi:10.1016/j.jobab.2020.03.001.
- Wenzel, R. N. 1949. Surface roughness and contact angle. The Journal of Physical and Colloid Chemistry 53 (9):1466–67. doi:10.1021/j150474a015.
- Wu, Y., Z. Qian, Y. Lei, W. Li, X. Wu, X. Luo, Y. Li, B. Li, and S. Liu. 2018. Superhydrophobic modification of cellulose film through light curing polyfluoro resin in situ. Cellulose 25 (3):1617–23. doi:10.1007/s10570-018-1676-8.
- Xiao, Z., Q. Zhang, J. Dai, X. Wang, Q. Yang, C. Cai, J. Mao, and Q. Ge. 2020. Structural characterization, antioxidant and antimicrobial activity of water-soluble polysaccharides from bamboo (Phyllostachys pubescens Mazel) leaves. International Journal of Biological Macromolecules 142:432–42. doi:10.1016/j.ijbiomac.2019.09.115.
- Yu, L., Z. Zhang, H. Tang, and J. Zhou. 2019. Fabrication of hydrophobic cellulosic materials via gas–solid silylation reaction for oil/water separation. Cellulose 26 (6):4021–37. doi:10.1007/s10570-019-02355-7.
- Yuan, J., Y. Zhu, J. Wang, Z. Liu, J. Wu, T. Zhang, P. Li, and F. Qiu. 2021. Agricultural bamboo leaf waste as carbon precursor for the preparation of Cu-Al/biomass fiber adsorption and its application in the removal of ammonia nitrogen pollutants from domestic wastewater. Journal of Wood Chemistry and Technology 41 (4):137–49. doi:10.1080/02773813.2021.1914110.