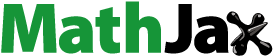
ABSTRACT
The cellulose/polyvinyl alcohol (PVA)/expanded graphite (EG) 3D porous foam, which has wide application prospects in cost-effective dye removal, was prepared by physical crosslinking and foaming technology. The prepared foam material has an obvious 3D network and porous structure, exhibiting excellent removal efficiency for methylene blue (MB) in an aqueous solution. The largest MB adsorption capacity of the foam is 110.81 mg/g. The adsorption process follows the pseudo-2nd order kinetics and the Freundlich isotherm model, indicating that the adsorption process is controlled by active surface sites and the physical adsorption process. Thermodynamic studies have shown that the adsorption process is a spontaneous and exothermic reaction. After five cycles of adsorption experiments, the composite material still exhibited a more than 70% dye removal rate. Results show that cellulose/PVA/EG 3D porous foam is an effective, promising, and recyclable adsorbent, which can be used to remove MB from aqueous solutions.
摘要
采用物理交联和发泡技术制备了纤维素/聚乙烯醇(PVA)/膨胀石墨(EG)3D多孔泡沫,该泡沫在经济高效的染料去除中具有广泛的应用前景. 制备的泡沫材料具有明显的3D网络和多孔结构,对水溶液中的亚甲基蓝(MB)表现出优异的去除效率. 泡沫的最大MB吸附量为110.81 mg/g. 吸附过程遵循伪二级动力学和Freundlich等温线模型,表明吸附过程由活性表面位点和物理吸附过程控制. 热力学研究表明,吸附过程是一个自发的放热反应. 在五个循环的吸附实验之后,复合材料仍然显示出超过70%的染料去除率. 结果表明,纤维素/PVA/EG 3D多孔泡沫是一种有效的、有前途的、可回收的吸附剂,可用于从水溶液中去除MB.
Introduction
Methylene blue (MB) is a cationic dye widely used in dyeing, chemical indicator, and biomedicine. However, it causes a lot of pollution and may lead to some diseases or adverse reactions, such as meningitis, neuronal cell apoptosis, nausea, and vomiting (Kai Ling et al. Citation2021). Many methods have already been applied in solving wastewater pollution, such as membrane filtration, flocculation, co-precipitation, chemical redox, and biological treatment (Abd Ali et al. Citation2021; Ismail et al. Citation2022; Peng, Zhu, and Mathew Citation2019; Usmani et al. Citation2021). However, these methods have some restricting factors in their application, such as high cost and secondary pollution by nano-powder adsorbents, which is hard to recycle. Adsorption is a popular method to remove organic dyes from wastewater (Karim et al. Citation2022). Compared with other methods, it shows low cost, high efficiency, easy recovery, and high selectivity (Bhatti et al. Citation2018).
As porous materials have been developed rapidly in recent decades, related research is focused on aerogels and hydrogels. 3D network materials with open porous and microporous structures show great potential in wastewater adsorption (Franco et al. Citation2021). Tang et al. (Citation2019) developed an amphiphilic graphene aerogel for the adsorption of malachite green, Rhodamine B, MB, and Methyl orange. Wang et al. (Citation2019) prepared a silk fibroin-modified graphene oxide-based aerogel, which showed excellent adsorption capacity (1322 mg/g) in MB. However, some problems of the studied porous materials include expensive raw materials, costly manufacturing, and complicated drying process (Asim et al. Citation2019; Karami Citation2018).
Foam materials also have an open porous structure, convenient preparation process, and extremely low production cost for wider use (Beili et al. Citation2021). The trimethylammonium grafted cellulose foam prepared by Feng et al. (Citation2020) can selectively adsorb anionic dyes and obtain better adsorption performance. Hong et al. (Citation2021) developed a flexible cellulose foam to obtain 116 mg/g adsorption capacity.
In this work, we prepared the cellulose/PVA/EG 3D porous foam by developing a simple and feasible strategy for the removal of MB. After simple foaming and solidification, the prepared foam has a stable 3D and open porous structure, which can efficiently remove MB and has broad application in MB adsorption.
Experiment
Materials
Microcrystalline cellulose (MCC, 98%, 20 μm), PVA (molecular weight 72,000), sodium dodecyl sulfate (SDS, 99%), sodium hydroxide (NaOH), polyethylene glycol 4000 (PEG), MB, and Ethanol were supplied by Sigma Aldrich (ST. Louis, USA). EG was provided by the Technical University of Liberec. All the solutions used distilled water with 18 MΩ cm electrical resistivity.
Preparation of cellulose solution in PEG+ NaOH System
The procedure of cellulose solution was carried out according to the method reported by Yan and Cernencu et al. (Cernencu et al. Citation2017; Yan Zhijuan Gao). At first, 2 wt% PEG and 9 wt% NaOH were dissolved in 40 ml of distilled water. Then, 2 wt% MCC was added to this solution, continuously stirring for 3 h. Finally, the suspension was frozen at −20°C for 12 h and then thawed at room temperature for better dissolution. This step was repeated three times.
Preparation of 3D porous foam material
At first, 10 wt% PVA and 5 wt% EG were added to the above cellulose solution with stirring at 600 rpm at 85°C for 3 h. Then, 2 wt% SDS was added with stirring for 30 min to emulsify and foam the suspension. At last, the suspension was frozen for solidification at −20°C for 72 h. After thawing, the porous foam material was obtained ( and ). Herein, samples were named according to the composition of the added chemicals, such as PVA+SDS (PS), MCC+PVA (CP), MCC+PVA+SDS (CPS), and MCC+PVA+SDS+EG (CPSG).
Table 1. Composition of substances in solution.
FTIR and Raman test
The changes in the functional group of samples were chemically investigated by the FTIR spectrometer (Nicolet IZ10). Spectrum was obtained in the spectral region between 400 cm−1 and 4000 cm−1 with 2 cm−1 resolution. The average number of scans is 32.
Morphology test of different composites
The surface morphology of porous foam material was observed by scanning electron microscope (SEM) (VEGA TESCAN Inc., USA) (Tan et al. Citation2022).
Adsorption experiment of MB
For the adsorption experiment, MB was chosen as a model dye to check the adsorption properties of porous foam materials. In short, different concentration solutions of MB were prepared by dilution for construction of the calibration curve (Fig. S1). Then, we put 0.06 g sample into 30 ml different concentration solutions of MB (25 mg/L, 50 mg/L, 100 mg/L, 150 mg/L, 200 mg/L, 250 mg/L). Also, different factors were also examined, such as pH (1, 4, 7, 10, 13), temperature (25°C, 50°C, 75°C). Adsorption kinetics and isotherms were studied by batch adsorption experiments, which were performed with different concentrations of dye solutions at 25°C, and the samples were tested every 30 min. For detailed kinetic and isotherm models, please refer to Table S1 and S2. Thermodynamics was tested by batch adsorption experiments at different temperature (25°C, 50°C, 75°C). The UV-spectrophotometer (7415, Cole-Parmer Ltd.) was utilized to determine the concentration of residual MB dye in the solution after adsorption at 665 nm wavelength. The dye removal efficiency and adsorption capacity were calculated according to Eq. 1 and Eq. 2 (Wang et al. Citation2018).
where ,
(mg/L) are the concentrations of MB at zero time and time t, respectively.
is the MB solution volume (L) and
(g) is the mass of the sample.
Thermodynamic of adsorption
Adsorption thermodynamic is used to survey the trend, degree, and driving force of the adsorption process and plays an important role in explaining the characteristics, laws, and mechanism of adsorption. The changes in the parameters of thermodynamics of the adsorption process are generally calculated by equations of Gibbs and Vant’ Hoffs equations (Lakouraj, Norouzian, and Balo Citation2015):
where (kJ/mol) is Gibbs free energy, R and T are gas constant (8.314 J/mol/K) and absolute temperature (K),
(L/mol) is the equilibrium constant of adsorption, and
and
are entropy and enthalpy, respectively.
Recycling test
The sample containing adsorbed MB was placed into absolute ethanol solution for 72 h at room temperature. Then, the adsorption experiment was repeated after most MB was desorbed from the samples. This test was performed at pH 13 and 25°C with 0.06 g adsorbent to 30 ml 150 mg/L MB concentration.
Results
Composition analysis
As can be seen in , the broad peak at 3282 cm−1 is attributed to the O-H stretching vibrations of cellulose and PVA. The peak at 2908 cm−1 is a saturated C-H stretching vibration, which may be caused by PVA, PEG, or SDS. The weak peak at 1652 cm−1 may be due to the C=C stretching vibration. The peaks at 1413 cm−1 and 1323 cm−1 may be due to the CH2 stretching vibration on PVA. The sharp peak at 1090 cm−1 is attributed to the C – O stretching vibrations of cellulose and PVA. It is worth noting that no characteristic peaks of SDS were found in CPSG, such as the peaks around 1250 cm−1and 900 cm−1in the SDS curve representing its stretching vibrations of CH3 and S=O, respectively. It shows that in the subsequent washing process, SDS is completely washed away. In addition, no new characteristic peaks appeared in CPSG other than the above peaks, suggesting that cellulose/PVA/EG may be a physical blend rather than a chemical association.
Figure 2. Results of FTIR and Raman test. (a: FTIR test; b: Raman test for PEG; c: Raman test for CPSG).
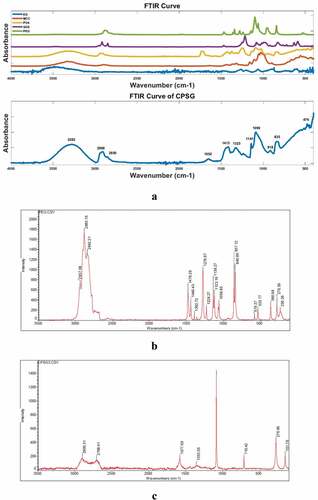
PEG is a condensation polymer of ethylene oxide and water with the general formula H (OCH2 CH2)n OH. It can be seen from the Raman spectrum that CPSG does not find obvious symmetrical stretching vibrations of PEG methylene groups at 2842 cm−1 and 2883 cm−1. In addition, the skeletal vibrations specific to PEG were not found at 840 cm−1 and 857 cm−1 in CPSG. It is shown that the CPSG samples do not contain PEG.
Surface morphology of different structures
As we can see from , it can be clearly observed that CPS and CPSG have a 3D porous structure. In contrast, PS only forms a 2D film, and CP forms a 3D structure without porous structure. At first, as a water-soluble, soft, and long-chain polymer, PVA has been widely applied in the preparation of permeable membrane, aerogel, and hydrogel due to its excellent film-forming and excellent chemical properties (Sonker et al. Citation2018). However, even if we added any foaming agent in this research, there was still no porous structure. In the subsequent thawing process, its 3D structure collapsed. In addition, bubbles generated by the foaming agent could not form porous structures in the final solidification due to the collapse of the overall structure.
Figure 3. Surface morphology. (a-: photo of PS; a: SEM of PS; b-: photo of CP b: SEM of CP; c-: photo of CPS; c: SEM of CPS; d-: photo of CPSG; d: SEM of CPSG).
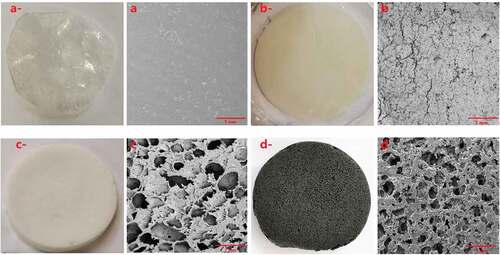
In contrast to CP, CPS and CPSG formed a good 3D structure. The main reason is the addition of cellulose. It is well known that cellulose consists of a well-organized crystalline region that contributes to the strength, high stiffness, and amorphous region, which makes fiber more flexible (Lavoine et al. Citation2012). Cellulose is added to the system as a reinforcing component filler, forming a uniform network and stable 3D structure in the mixed solution with PVA through hydrogen bonding and supramolecular interaction.
In this research, cellulose/PVA/EG 3D porous foam was physically cross-linked. Then, the PEG and SDS were washed away in the subsequent washing process and initially utilized SDS as a foaming agent and surfactant to prepare foam material. Compared with CPS and CPSG, SDS is the key to porous forming. Foam is a complex gas/liquid dispersion system. Due to the effect of high shear force, massive bubbles are generated and accumulated in a trace amount of surfactant solution to form a liquid foam slurry. As shown in , those irregular bubbles fix the cellulose and EG particles between the gas and liquid phases. In addition, the liquid foam can be prepared by rearranging SDS molecules at the surface of air bubbles entrapped in a suspension. The hydrophobic area of SDS extends out of the bubble surface, and the hydrophilic area combines with water to form a surfactant layer. The negative charge of cellulose and EG caused electrostatic repulsion with SDS. So, they are repelled between the bubbles to form a water layer containing cellulose and EG. Although it has the same charge as cellulose and EG, they still adsorb to hydrophobic sites to form aggregates as SDS is a strong surfactant. In conclusion, cellulose is a strong reinforcing material that guarantees the porous structure formed by SDS.
Influence of different factors on adsorption performance
At first, transmission resistance is affected by many factors, including the type and structure of the adsorbent. We investigated the adsorption performance of different samples at relatively lower dye concentrations (25 mg/L). As seen in , CPSG has the highest removal rate of dye (96.21%) and adsorption capacity (12.03 mg/g) compared to CP (16.51%, 2.06 mg/g) and CPS (65.02%, 8.13 mg/g). This is mainly due to the porous structure of CPSG, and MB molecules easily diffuse from the external surface to the internal surface. Cellulose provides support for forming a stable foam structure and increases the contact between cellulose and MB. At the same time, the negative charge of cellulose has a certain degree of electrostatic adsorption to MB. Moreover, EG provides more active sites and significantly improves the adsorption performance. At the same time, the negative charges provided by the cellulose and EG improve MB adsorption.
Figure 5. A: The influence of CP, CPS, and CPSG on adsorption, b: The effect of different initial concentrations of dyes on adsorption, the influence of different pH values on adsorption, d: The effect of temperature on adsorption.
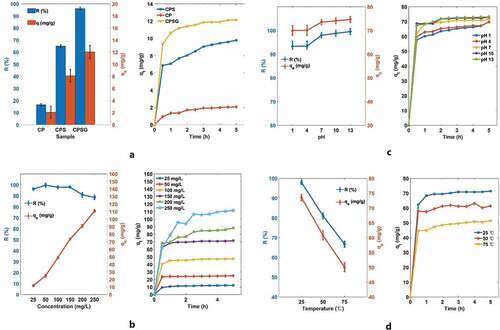
In addition, the effect of different initial dye concentrations (25–250 mg/L) on the adsorption performance was investigated. It can be seen from that as the initial dye concentration increases, the adsorption capacity increases significantly from 12.03 mg/g to 110.81 mg/g, which is possibly caused by concentration polarization. At higher dye concentration, concentration polarization accelerates the diffusion rate of MB molecules on the surface of the adsorbent, and more dye molecules are adsorbed.
The pH value is also one of the important factors influencing adsorption performance. The research results show that the removal rate and adsorption capacity of MB increase with the increase in pH value, with results from similar studies () (Abd-Elhamid et al. Citation2019; Eltaweil et al. Citation2020). Cellulose is easily protonated in a low pH environment. This will gradually convert the surface of the material to a positive charge, causing repulsion with MB and reducing the adsorption efficiency.
Moreover, the influence of different temperatures on the adsorption performance was investigated. It can be seen from that as the temperature increases, the dye removal rate and adsorption capacity of the adsorbent decrease significantly, indicating that temperature is also an important influencing factor of the adsorption performance. As the temperature increases, the thermal mobility of dye molecules increases, which may accelerate their transport to the surface of the adsorbent. However, at the same time, it may also accelerate the desorption of dye molecules from the surface of the adsorbent.
Adsorption kinetic of adsorbent in MB
There are two possible interactions between adsorbate and adsorbent, namely chemical and physical interactions. It is very important to figure out whether an adsorption phenomenon is a chemical or physical adsorption, which can help us understand its adsorption mechanism. Adsorption kinetics reveals the relationship between adsorption efficiency and time and is a good tool to analyze the adsorption rate. This research chooses the three most commonly used kinetic models (Pseudo-1st order, Pseudo-2nd order, and Intraparticle diffusion (IPD) model) to study the adsorption rate. The equation of Linearized form is shown in Table S1.
The adsorption kinetics curves and parameters are shown in and . It is obvious that the Pseudo-2nd order is more suitable than the Pseudo-1st order. The largest theoretical MB adsorption capacity of the foam is 123 mg/g. It reveals that the adsorption process depends on the number of active sites. However, it has been reported in the literature that the kinetic data near or in equilibrium may have serious deviations if a Pseudo-2nd order kinetic model is applied (Simonin Citation2016). Therefore, other models are needed for further study on the adsorption mechanism.
Table 2. Parameters of adsorption kinetic.
The IPD model is used to determine the rate-limiting steps of the entire adsorption process. As shown in and , indicates changes in diffusion rate, revealing that the adsorption process mainly follows three steps: (1) The first stage is the diffusion of MB molecules from the body to the external surface of the adsorbent; (2) Then, MB molecules diffuse through the pores and diffuse into the internal surface; (3) The last stage is near equilibrium stage. In this stage, a balance between adsorption and desorption is reached. This model is in excellent correspondence with the results of comparison between adsorption capacity and time in the previous section. At the initial stage of the adsorption process, MB is adsorbed on the surface of the adsorbent at a very fast rate, and then slowly diffuses to the interior, finally reaching equilibrium.
Adsorption isotherm of adsorbent in MB
The adsorption isotherm is to study the relationship between the concentration of adsorbate in the liquid phase and the adsorbent at a specific temperature at equilibrium. In this study, several models (Langmuir, Freundlich, and Temkin) were selected to analyze the adsorption mechanism (Table S2).
It can be seen from and that the Freundlich model (R2 = 0.954, 0.991, 0.979) is more suitable than Langmuir (R2 = 0.983, 0.863, 0.771) and Temkin model (R2 = 0.935, 0.871, 0.919) for describing the adsorption of MB molecules on cellulose/PVA/EG porous materials. It reveals the multilayer adsorption of MB on heterogeneous surfaces. Multilayer adsorption is due to mutual attraction between molecules, and additional molecules are superimposed on the first adsorption layer to form multilayer adsorption, which is essentially physical adsorption. In addition, represents the adsorption strength of the adsorbent. When
, it indicates that the adsorption behavior is favorable (Eltaweil et al. Citation2020). At the same time, the porous adsorbent has a greater adsorption strength at 25°C as the lowest temperature in the test, indicating that high temperature is unfavorable for the adsorption behavior, which further supports its physical adsorption behavior.
Table 3. The key parameters of adsorption isotherm model.
Thermodynamic of adsorption
As shown in , the free energy is negative, indicating that the porous material adsorbs MB as a spontaneous process. As the temperature increases, the absolute value of
decreases, indicating that the spontaneous tendency of adsorption decreases, which is not conducive to adsorption. The negative value of the adsorption enthalpy
reveals that the adsorption process is an exothermic reaction. The negative value of the adsorption entropy
indicates that the adsorption reduces the degree of freedom of the adsorbate molecules.
Table 4. Parameters of the thermodynamic model.
Reusability of the adsorbent
For industrial applications, the regeneration and recycling of adsorbents are very important. In this study, the adsorbent after adsorption was immersed in an ethanol solution to regenerate it. The experimental result shows that after five cycles, the MB dye removal rate of the composite material drops from 97.98% to 71.93%, possibly for a combination of chemistry and physics adsorption of cellulose/PVA/EG foam (). As is known to all, chemical adsorption is irreversible, but physical adsorption is reversible. Therefore, the adsorption process was mainly led by physical adsorption in the subsequent cycle. To sum up, the removal rate of adsorbents after regeneration remains high, which is appropriate for the treatment of MB wastewater.
Adsorption mechanism of cellulose/PVA/EG foam in MB
The adsorption mechanism of the adsorbate on the adsorbent is affected by many factors, such as the size and structure of the adsorbent. Based on the above experimental results, the adsorption mechanism scheme lists in . According to the analysis of adsorption kinetics and isotherm, the adsorption process is mainly physical adsorption affected by the number of active sites and adsorbent structure. So, the possible interactions that determine the effect of MB adsorption are electrostatic adsorption, hydrogen bonding, and van der Waals force.
Conclusion
In this study, cellulose/PVA/EG 3D porous foam material was prepared by a simple foaming technique using physical crosslinking. Results show that cellulose strongly supports the 3D network structure of the material, and SDS effectively promotes the formation of the porous structure. The addition of EG significantly improves the removal efficiency of methylene blue. In the adsorption experiment, the dye concentration, pH, and temperature significantly affect the adsorption result of the material. The adsorption capacity is directly proportional to dye concentration and pH, while the adsorption capacity is inversely proportional to temperature. The adsorption process follows the pseudo-2nd order kinetics and the Freundlich isotherm model. Thermodynamic studies have shown that the adsorption process is a spontaneous and exothermic reaction. In addition, after five cycles of adsorption, the repeatability study found that the dye removal rate remained high, although the adsorption capacity of the material decreased slightly. Therefore, the prepared cellulose-based foam material is a stable, environmentally friendly, efficient, and reusable adsorbent for MB removal.
Highlights
Cellulosic/polyvinyl alcohol/expanded graphite 3D porous foam was prepared by a simple low-temperature solidification method.
The adsorption process follows the pseudo-2nd order kinetics and the Freundlich isotherm model and the largest theoretical MB adsorption capacity of the foam is 123 mg/g.
After five cycles of adsorption, the dye removal rate of more than 70% was still maintained.
Ethics approval and consent to participate
Manuscripts reporting studies that do not involve human participants, human data, or human tissue.
Supplemental Material
Download MS Word (196 KB)Acknowledgements
This work was supported by the project ‘Advanced structures for thermal insulation in extreme conditions’ (Reg. No. 21–32510 M) granted by the Czech Science Foundation (GACR) and was supported by the research project of Student Grant Competition of Technical University of Liberec no. 2022-6069.
Disclosure statement
We have no known competing financial interests or personal relationships that could have appeared to influence the work reported in this paper.
Supplementary material
Supplemental data for this article can be accessed online at https://doi.org/10.1080/15440478.2023.2190189
Additional information
Funding
References
- Abd Ali, L. I., H. K. Ismail, H. F. Alesary, and H. Y. Aboul-Enein. 2021. A nanocomposite based on polyaniline, nickel and manganese oxides for dye removal from aqueous solutions. In International Journal of Environmental Science and Technology, Vol. 18 7–15 2031–2050. Springer.
- Abd-Elhamid, A. I., E. A. Kamoun, A. A. El-Shanshory, H. M. A. Soliman, and H. F. Aly. 2019. Evaluation of graphene oxide-activated carbon as effective composite adsorbent toward the removal of cationic dyes: composite preparation, characterization and adsorption parameters. Journal of Molecular Liquids 279 (April):530–39. Elsevier. doi:10.1016/J.MOLLIQ.2019.01.162.
- Asim, N., M. Badiei, M. A. Alghoul, M. Mohammad, A. Fudholi, M. Akhtaruzzaman, N. Amin, and K. Sopian. 2019. Biomass and industrial wastes as resource materials for aerogel preparation: opportunities, challenges, and research directions. In Industrial & Engineering Chemistry Research Vol. 58 (38): 17621–45. American Chemical Society. doi:10.1021/acs.iecr.9b02661
- Beili, L., Q. Lin, Z. Yin, F. Lin, X. Chen, and B. Huang. 2021. Robust and lightweight biofoam based on cellulose nanofibrils for high-efficient methylene blue adsorption. In Cellulose, Vol. 28 1–288 273–288. Springer.
- Bhatti, H. N., J. Hayat, M. Iqbal, S. Noreen, and S. Nawaz. 2018. Biocomposite application for the phosphate ions removal in aqueous medium. Journal of Materials Research and Technology 7 (3):300–07. Elsevier. doi:10.1016/J.JMRT.2017.08.010.
- Cernencu, A. I., A. Lungu, D. Dragusin, A. Serafim, E. Vasile, C. Ionescu, and H. Iovu. 2017. Design of cellulose–alginate films using PEG/NaOH aqueous solution as co-solvent. Cellulose 24 (10):4419–31. doi:10.1007/s10570-017-1412-9.
- Eltaweil, A. S., H. Ali Mohamed, M.A.E.M. Eman, and G. M. El-Subruiti. 2020. Mesoporous magnetic biochar composite for enhanced adsorption of malachite green dye: characterization, adsorption kinetics, thermodynamics and isotherms. Advanced Powder Technology 31 (3):1253–63. Elsevier. doi:10.1016/J.APT.2020.01.005.
- Feng, C., P. Ren, M. Huo, Z. Dai, D. Liang, Y. Jin, and F. Ren. 2020, August. Facile synthesis of trimethylammonium grafted cellulose foams with high capacity for selective adsorption of anionic dyes from water. ( Elsevier) Carbohydrate Polymers 241:116369. doi:10.1016/J.CARBPOL.2020.116369.
- Franco, P., S. Cardea, A. Tabernero, and I. De Marco. 2021. Porous aerogels and adsorption of pollutants from water and air: a review. Molecules 26 (15):4440. doi:10.3390/molecules26154440.
- Hong, M., X. Fei Zhang, Z. Wang, L. Song, and J. Yao. 2021, March. Flexible cellulose foams with a high loading of attapulgite nanorods for Cu2+ Ions removal colloids and surfaces. ( Elsevier) Physicochemical and Engineering Aspects 612:126038. doi: 10.1016/J.COLSURFA.2020.126038.
- Ismail, H. K., L. I. Abd Ali, H. F. Alesary, B. K. Nile, and S. Barton. 2022. Synthesis of a poly (p-aminophenol)/starch/graphene oxide ternary nanocomposite for removal of methylene blue dye from aqueous solution. Journal of Polymer Research 29 (5–22). Springer:1–22.
- Kai Ling, Y., X. Jiat Lee, H. Chyuan Ong, W.H. Chen, J.S. Chang, C.S. Lin, P. Loke Show, and T. Chuan Ling. 2021. Adsorptive removal of cationic methylene blue and anionic congo red dyes using wet-torrefied microalgal biochar: equilibrium, kinetic and mechanism modeling. Environmental Pollution 272:115986. Elsevier. doi:10.1016/j.envpol.2020.115986.
- Karami, D. 2018. A review of aerogel applications in adsorption and catalysis. In Journal of Petroleum Science and Technology, Vol. 8 4 3–15. Research Institute of Petroleum Industry (RIPI).
- Karim, S., N. Ahmad, D. Hussain, Y. Sun Mok, and G. Uddin Siddiqui. 2022. Active removal of anionic azo dyes (MO, CR, EBT) from aqueous solution by potential adsorptive capacity of zinc oxide quantum dots. In Journal of Chemical Technology & Biotechnology, Wiley Online Library.
- Lakouraj, M. M., R.S. Norouzian, and S. Balo. 2015. Preparation and cationic dye adsorption of novel Fe3O4 supermagnetic/thiacalix[4]arene tetrasulfonate self-doped/polyaniline nanocomposite: kinetics, isotherms, and thermodynamic study. In Journal of Chemical & Engineering Data Vol. 60 (8): 2262–72. American Chemical Society. doi:10.1021/acs.jced.5b00080
- Lavoine, N., I. Desloges, A. Dufresne, and J. Bras. 2012. Microfibrillated cellulose – Its barrier properties and applications in cellulosic materials: A review. Carbohydrate Polymers 90 (2):735–64. Elsevier Ltd. doi:10.1016/j.carbpol.2012.05.026.
- Peng, L., C. Zhu, and A. P. Mathew. 2019. Mechanically robust high flux graphene oxide - nanocellulose membranes for dye removal from water. Journal of Hazardous Materials 371 (June):484–93. Elsevier. doi:10.1016/J.JHAZMAT.2019.03.009.
- Simonin, J. P. 2016. On the comparison of pseudo-first order and pseudo-second order rate laws in the modeling of adsorption kinetics. Chemical Engineering Journal 300 (September):254–63. Elsevier. doi:10.1016/J.CEJ.2016.04.079.
- Sonker, A. K., K. Rathore, R. Krishna Nagarale, and V. Verma. 2018. Crosslinking of polyvinyl alcohol (PVA) and effect of crosslinker shape (aliphatic and aromatic) thereof. Journal of Polymers and the Environment 26 (5):1782–94. doi:10.1007/s10924-017-1077-3.
- Tang, S., D. Xia, Y. Yao, T. Chen, J. Sun, Y. Yin, W. Shen, and Y. Peng. 2019, October. Dye adsorption by self-recoverable, adjustable amphiphilic graphene aerogel. ( Academic Press) Journal of Colloid and Interface Science 554:682–91. doi:10.1016/J.JCIS.2019.07.041.
- Tan, X., Q. Peng, K. Yang, T. Yang, J. Saskova, J. Wiener, M. Venkataraman, J. Militky, W. Xiong, and X. Jie. 2022. Preparation and characterization of corn husk nanocellulose coating on electrospun polyamide 6. Alexandria Engineering Journal 61 (6):4529–40. Elsevier. doi:10.1016/J.AEJ.2021.10.011.
- Usmani, Z., M. Sharma, A. Kumar Awasthi, N. Sivakumar, T. Lukk, L. Pecoraro, V. Kumar Thakur, D. Roberts, J. Newbold, and V. Kumar Gupta. 2021. Bioprocessing of waste biomass for sustainable product development and minimizing environmental impact. Bioresource Technology 322:124548. doi:10.1016/j.biortech.2020.124548.
- Wang, S., H. Ning, H. Ning, K. Huang, S. Weng, W. Xiaopeng, W. Liangke, J. Liu, Alamusi. 2019. Preparation and characterization of graphene oxide/silk fibroin hybrid aerogel for dye and heavy metal adsorption. Composites Part B: Engineering, 163 (April):716–22. Elsevier. doi:10.1016/J.COMPOSITESB.2018.12.140.
- Wang, Y., L. Zhao, J. Hou, H. Peng, W. Jianning, Z. Liu, and X. Guo. 2018. Kinetic, isotherm, and thermodynamic studies of the adsorption of dyes from aqueous solution by cellulose-based adsorbents. Water Science and Technology 77 (11):2699–708. doi:10.2166/wst.2018.229.