Abstract
Diesel engine exhaust (DE) consists of a complex mixture of gases and aerosols, originating from sources such as engines, turbines, and power generators. It is composed of a wide range of toxic compounds ranging from constituents that are irritating to those that are carcinogenic. The purposes of this work were to characterize DE originating from different engine types on a ship operating offshore and to quantify the potential exposure of workers on the ship’s helicopter deck to select DE compounds. Sampling was conducted on a Norwegian Nansen-class frigate that included helicopter operations. Frigate engines and generators were fueled by marine diesel oil, while the helicopter engine was fueled by high flash point kerosene-type aviation fuel. Exhaust samples were collected directly from the stack of the diesel engine and one of the diesel generator exhaust stacks, inside a gas turbine exhaust stack, and at the exhaust outlet of the helicopter. To characterize the different exhaust sources, non-targeted screening of volatile and semi-volatile organic compounds was performed for multiple chemical classes. Some of the compounds detected at the sources are known irritants, such as phthalic anhydride, 2,5-dyphenyl-p-benzoquinone, styrene, cinnoline, and phenyl maleic anhydride. The exhaust from the diesel engine and diesel generator was found to contain the highest amounts of particulate matter and gaseous compounds, while the gas turbine had the lowest emissions. Personal exposure samples were collected outdoors in the breathing zone of a helicopter deck operator over nine working shifts, simultaneously with stationary measurements on the helicopter deck. Elemental carbon, nitrogen dioxide, and several volatile organic compounds are known to be present in DE, such as formaldehyde, acrolein, and phenol were specifically targeted. Measured DE exposures of the crew on the helicopter deck were variable, but less than the current European occupational exposure limits for all compounds, except elemental carbon, in which concentration varied between 0.5 and 37 µg/m3 over nine work shifts. These findings are among the first published for this type of working environment.
Introduction
Diesel engine exhaust (DE) consists of solid, condensed, and gas phases. The solid phase includes organic carbon (OC) and elemental carbon (EC) particles and minor amounts of metals (U.S. EPA Citation2002), while the condensed phase is composed of low volatility organics adsorbed on the solid particles (McDonald et al. Citation2004) as the exhaust cools after leaving the engine. Gases formed by the combustion of diesel consist of mainly carbon dioxide and water vapor (Moldanová et al. Citation2009). A smaller proportion of the gas compounds in DE are carbon monoxide (CO), nitrogen oxides (NOx), and sulfur dioxide (SO2) together with a complex mixture of volatile organic compounds (VOCs) (Taxell and Santonen Citation2017). VOCs in DE include several functional classes such as large aliphatic hydrocarbons; alcohols; reactive hydrocarbons including ethylene (Steiner et al. Citation2016); carbonyl compounds containing formaldehyde, acetaldehyde, and acrolein (Reda et al. Citation2015); polycyclic aromatic hydrocarbons (PAHs) encompassing naphthalene, benzo[a]pyrene, anthracene; as well as aromatic compounds such as benzene, toluene, ethylbenzenes, and xylenes (BTEX) (Moldanová et al. Citation2009; IARC Citation2012; Perrone et al. Citation2014). Some of these PAHs and VOCs have known toxic effects depending on dose and duration of exposure, including genotoxicity and carcinogenicity (IARC Citation2012), and can cause nausea and dizziness upon acute exposure (Steiner et al. Citation2016; Joshi Citation2019). Gaseous compounds in DE are well known for their odor, and nitrogen dioxide (NO2), sulfur dioxide (SO2), formaldehyde, acrolein, and phenol are irritants (Cernansky Citation1983). Chemicals adsorbed on the carbon matrix of diesel particles can interact with respiratory epitheliums and may elicit a multitude of health effects ranging from cardiovascular and metabolic diseases (Holme et al. Citation2019; Brinchmann et al. Citation2023) to carcinogenesis (Steiner et al. Citation2016).
In occupational settings where diesel engines are used, EC is commonly regarded as the marker for DE particulate matter (PM) (Birch and Cary Citation1996; Birch Citation2002). Nitrogen dioxide and CO have been widely used as markers for the gas phase, even though these are nonspecific compounds present in many occupational settings (Pronk et al. Citation2009; Hedmer et al. Citation2017). The composition of DE depends on several parameters such as fuel type, engine type and load, combustion temperatures, and type and efficiency of aftertreatment (Zhang et al. Citation2021). Aftertreatment of DE in the marine sector is mainly based on selective catalytic reduction technology, which reduces NOx emissions (Zhu et al. Citation2022). The two conventional diesel fuel types used for marine operations are heavy fuel oil (HFO) and marine diesel oil (MDO) (Chu Van et al. Citation2019), the main difference being sulfur content. Diesel engines running at optimal design capacity typically have the lowest emissions of unburnt fuel (OC). When idling or operating at low engine loads, diesel engines typically have higher OC, CO, and VOC emissions (Cooper Citation2001; Citation2003).
Since 2012, DE has been classified as carcinogenic to humans (Group 1) by the International Agency for Research on Cancer (IARC) on the basis that, depending on duration and dose, exposure to DE increases the risk of lung cancer (IARC Citation2012). Other adverse health effects such as exacerbation of asthma, irritation of the eyes and upper respiratory tract, cough, pneumonia, and cardiovascular disease have also been reported following DE exposure (Lloyd and Cackette Citation2001; Ris Citation2007; Taxell and Santonen Citation2017). There has been much focus on characterizing emissions associated with diesel-powered ships concerning the environment, e.g., regional, and worldwide air quality and climate research (Moldanová et al. Citation2013; Zhang et al. Citation2021), but the literature regarding exposure to DE in seafarers is scarce. Seafarers are often exposed to DE over long working hours, with typical shipboard work durations ranging from days to months in an environment where exposures have not been systematically studied. One study assessed the indoor air quality on board an icebreaker operating on two types of fuel (HFO and MDO) (Langer et al. Citation2020). Diesel engine exhaust exposures in port or dock workers have been reported (Methner and Boudreau Citation2006; Debia et al. Citation2016), and exposure to benzene in DE among fishermen was studied (Kirrane et al. Citation2007). Personal exposure to NO2 has been studied in a submarine (Bondi et al. Citation1983), and an assessment of EC and OC concentrations in the engine room of a submarine have been suggested as estimates of occupational and environmental exposure in this marine environment (Gan et al. Citation2010).
Bendtsen et al. (Citation2021) reported that the health effects of exposure to gas turbine aircraft engine emissions are similar to those caused by exposure to DE (Bendtsen et al. Citation2021). A range of kerosene-based aviation fuels are in use throughout the world and are overall similar in chemical composition (Lighty et al. Citation2000; Mazaheri et al. Citation2011). Kerosene lies between the distillated crude oil fractions of gasoline (gasoline combustion exhaust is classified as IARC Group 2B—possibly carcinogenic to humans) and diesel (diesel combustion exhaust is classified as IARC Group 1—carcinogenic to humans), thus kerosene-type aircraft fuel combustion products could have carcinogenic potential, given the reported similarities to DE particles (Bendtsen et al. Citation2021).
This study aims to characterize emissions from different engine types on a ship, quantify the personal exposure of helicopter deck operators on board, and describe the variability of the potential exposures estimated from stationary measurements over several days. Indoor measurements were not the focus of the study because the ship is equipped with an effective air filtering system, and initial assessment of exposure concluded that the helicopter deck was the only work area with potentially significant exhaust exposures. The combination of personal measurements, in-situ quantification, as well as source characterization of EC, NO2, and several volatile and semi-volatile components will contribute to increasing knowledge and understanding of exposures to DE and helicopter engine exhaust in offshore environments.
Methods
Engines
The ship is a Royal Norwegian Navy Nansen-class frigate, which has combined diesel and gas turbine propulsion consisting of two cruising-speed 4.5 MW Bazan-Bravo diesel engines and a 21.5 MW General Electric LM 2500 gas turbine for high-speed operation. In addition, the ship has four MTU 12V396 diesel generators. The four different engine types potentially contributing to DE emissions on board are the main diesel engines (2), gas turbine engine operating 9 out of 12 days, diesel power generators (4), and the gas turbine engine of the NH90 helicopter operating 9 out of 12 days on the helicopter deck. The ship engines, including the generators and the gas turbine, were operated on MDO, while the helicopter engine was operated on high flash point kerosene-type aviation fuel.
Sampling
Except for the helicopter engine exhaust, all samples were collected on board while navigating, over a two-week sampling campaign in October 2020. For chemical characterization of the DE sources, samples were collected directly at the stack of one of the generator exhaust outlets, and approximately 1 meter downwind from the main engine exhaust outlets. For safety reasons, samples from the gas turbine engine were collected inside the exhaust stack. The helicopter engine exhaust was sampled on shore, downstream of the exhaust pipe at a safe distance of approximately 3 meters during engine idling. Personal samples for exposure assessment were collected in the breathing zone of a helicopter deck operator during the working shift including breaks, which represents the helicopter deck crew (typically at least two persons). Helicopter deck operators supervise the work on the helicopter deck during operations and are the communications contact between the ship and the helicopter crew. Helicopter deck crew members typically worked shifts between 6 and 12 hr duration. Personal samples were collected from helicopter deck operators that worked for 9 days during helicopter operations. Simultaneously with personal samples, stationary samples were collected on the helicopter deck at approximately 2 m high. The helicopter deck was situated at the stern of the ship. details the samplers and airflow used for both characterization and exposure measurements. In addition to the filter samples, real-time Dräger PAC 7000 loggers for CO and NO2 (Drägerwerk AG & Co, Lübeck, Germany) were attached to the sampling station and in the breathing zone of the worker, logging concentrations every 10 sec. The helicopter deck operator also carried a real-time Dräger PAC 7000 logger for SO2 (Drägerwerk AG & Co, Lübeck, Germany).
Table 1. Components sampled for characterization of diesel engine exhaust and exposure assessment, with sampler type, flow, and time.
Analyses
Elemental carbon was quantified using an organic carbon – elemental carbon (OC-EC) dual-optical analyzer (Sunset Laboratory Inc, Tigard, OR, USA) as described in the National Institute for Occupational Safety and Health, NIOSH method 5040 (Birch Citation2002; NIOSH Citation2016c). Nitrogen dioxide was determined using spectrophotometry, details are given in the electronic supplement (ES) document. Phenol collected on XAD-7 tubes was analyzed based on the Occupational Safety and Health Administration (OSHA) method 32 (2001) (OSHA Citation2021), see ES for further details. The quantification of carbonyl compounds (formaldehyde, acetaldehyde, acetone, acrolein, and propanal) collected on Sep-pack cartridges was performed with HPLC-UV as described by Noskov et al. (Citation2019). The determination of VOCs sampled on charcoal adsorbent tubes was based on NIOSH 1500 method (NIOSH Citation2016a) for the determination of hydrocarbons, and on NIOSH 1501 method (NIOSH Citation2016b) for aromatic hydrocarbons, respectively. Further details are found in the ES. A non-targeted screening of VOCs sampled on Tenax TA – filled TD-tubes was performed using a thermal desorption system (TD100-xr, Markes Int., Ltd., Llantrisant, RCT, UK) equipped with an Agilent 7890B GC and an Agilent 5977B MS detector (Agilent Technologies, Santa Clara, CA, USA). Further details are described in the ES. Particles filtered onto TEM grids were analyzed in a Hitachi SU6600 field emission scanning electron microscope (Hitachi, Tokyo, Japan) using 20 keV at a working distance of 10 mm. The morphology of the particles was investigated in secondary electron imaging mode. Backscatter imaging mode was applied to search for bright spots, which may be inclusions of heavier elements such as lead. The laboratory that performed these determinations follows the ISO 17025:2017 standard recommendations (ISO/IEC Citation2017).
Statistics
Normality for EC, NO2, formaldehyde, and methylcyclohexane from the exposure samples were assessed using the Shapiro-Wilk normality test. Associations were assessed with least square regression analysis and Pearson’s correlation coefficient was calculated as the measure of the association. All statistical calculations were performed in Rstudio version [4.2.1] (R Foundation for Statistical Computing, Vienna, Austria). Geometric mean and standard deviation were calculated for EC, NO2, and formaldehyde using CRAN package “EnvStats” (Millard Citation2013). However, we do not report the geometric mean and standard deviation for other compounds because the data was highly censored. Figures were made using the same software, with additional CRAN packages: “ggplot2” (Wickham Citation2009), “ggpubr” version 0.4.0, “ggsci” version 2.9, and “openair” (Carslaw and Ropkins Citation2012).
Results
Chemical characterization of engine exhaust sources
The carbonyls acetaldehyde, acetone, acrolein, and propanal collected on Sep-pack cartridges were all below the respective detection limit of 0.02 ppm (acetaldehyde) and 0.01 ppm (others). Formaldehyde, on the other hand, was found in the exhaust of the diesel generator (0.94 ppm), diesel engine (0.16 ppm), and gas turbine (0.02 ppm) but was below the limit of detection (LOD) in the helicopter engine exhaust (<0.02 ppm). Phenol was not detected above the LOD (<0.10 ppm) in XAD adsorbent tubes; however, it was identified in all four exhaust sources with the analysis of TD tubes, due to the higher sensitivity of the latter method.
Concentrations of EC and NO2 differed among the diesel engine and generator. The concentration of EC in exhaust from the ship’s main engine was 0.6 mg/m3 and 1.2 mg/m3 in the exhaust from the diesel generator, while EC was below the detection limit in both the helicopter and gas turbine exhausts. NO2 was detected among all exhausts with concentrations of 0.5 mg/m3 for the diesel generator exhaust and 1.5 mg/m3 in the diesel engine exhaust. It was also detected in the helicopter engine exhaust and the gas turbine exhaust at a concentration of 0.03 and 0.02 mg/m3, respectively. displays the concentration ratio of selected compounds in the diesel engine, or generator, to the gas turbine.
Figure 1. Selected volatile and semi-volatile organic compounds, nitrogen dioxide, and elemental carbon found in exhaust from the diesel engine and the diesel generator, shown as a concentration ratio to the gas turbine, in logarithmic scale.
*Concentration in the gas turbine exhaust were below the limit of detection (LOD), therefore the ratio is calculated against the LOD for the compounds.
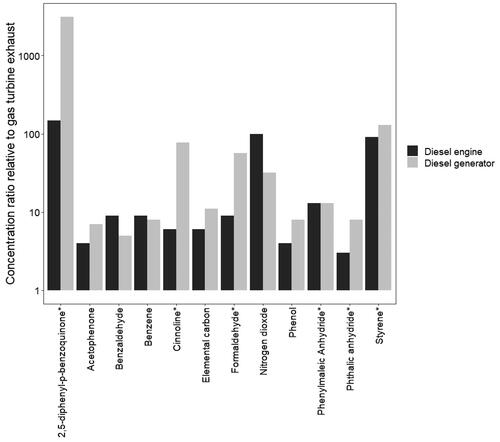
A wide range of compounds of several functional classes was detected in the four different exhausts with a non-targeted screening of VOCs by thermal desorption tubes. An overview of these compounds is presented in . Some of the VOCs detected in the exhaust are known irritants, these include phthalic anhydride (CAS 85-44-9) and phenyl maleic anhydride (CAS 36122-35-7), the quinone 2,5-dyphenyl-p-benzoquinone (CAS 844-51-9), styrene (CAS 100-42-5), and cinnoline (CAS 253-66-7), a nitrogen-containing diazine analogous to naphthalene. Phthalic anhydride was detected in all sources and is a known eye, respiratory tract, and skin irritant (PubChem Citation2022c). The 2,5-dyphenyl-p-benzoquinone was present in exhaust samples from both the diesel engine and diesel generator and is known to cause breathing difficulties (European Chemicals Agency Citation2022b). This compound is, however, not volatile, and its presence in the TD tubes is likely associated with DE PM, where other analogous quinones have been identified (Cho et al. Citation2004). The identification of quinones in this study is uncertain due to the TD tubes and GC-MS method used being less suitable for its high boiling point (458 ± 45 °C). Styrene and cinnoline were detected in the diesel engine and generator exhaust. Styrene is an acute eye and mucous membrane irritant which has been shown to have neurotoxic effects in animal studies (PubChem Citation2022a), whereas cinnoline is a known skin and eyes irritant (PubChem Citation2022b). Several PAHs including naphthalene and phenanthrene, among others, were detected in the diesel engine and generator exhausts as well as in the helicopter engine exhaust. Similarly, to other semi-volatile organic compounds (SVOC), PAHs in the exhausts are likely associated with soot particles or the condensed phase.
Table 2. Non-targeted volatile and semi-volatile organic compound screening of engine exhaust from diesel engine, generator, gas turbine, and helicopter.
Freshly produced soot particles collected from the chimney of the main diesel engine are shown in . An overview of particles collected onto a TEM grid is presented in , and a magnification of a soot agglomerate is shown in . No other particles like carbon nanotubes were observed or inclusions of metals, neither in secondary electron nor in backscatter imaging mode.
Occupational exposure
Carbon monoxide and NO2 real-time concentrations measured in the breathing zone of the helicopter deck operator with electrochemical sensors showed generally low concentrations (below 10 parts per million by volume, ppm, for CO and below 1 ppm for NO2, respectively), similar to the measured concentration at the stationary sampling station. Concentrations of SO2 were below LOD during the whole sampling campaign.
Pollution roses showing the frequency of wind direction relative to the ship position and the concentration of NO2 and CO measured at the stationary position are presented in . The wind direction relative to the ship was mainly headwind, at speeds below 10 m/s approximately 70% of the time and between 10 and 15 m/s for approximately 30% of the time. These results show that DE from the ship’s main engine, generator, or gas turbine were the main contributors to NO2 during the campaign since the sampling position at the helicopter deck is situated at the stern of the ship. In this investigation, the concentration of CO on the helicopter deck appeared to be less associated with the wind direction compared to that of NO2, possibly due to the dilution of engine exhaust constituents due to mixing with ambient air.
Figure 3. Concentrations (ppm) of carbon monoxide (CO, left) and of nitrogen dioxide (NO2, right) measured stationary on the helicopter deck at the stern. Wind direction relative to the ship position (center of the plot) during the sampling campaign.
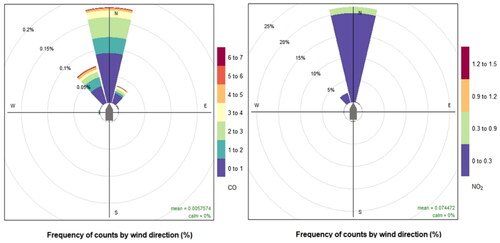
The concentrations of EC collected onto filters, and of NO2 and formaldehyde over 9 (personal) and 12 (stationary) work shifts are presented in . As expected, the concentration varies greatly between the different days, and the pattern of variation is similar between personal and stationary samples.
Figure 4. Concentration (µg/m3) of different compounds sampled during a work shift over 9 to 12 consecutive days. EC = elemental carbon.
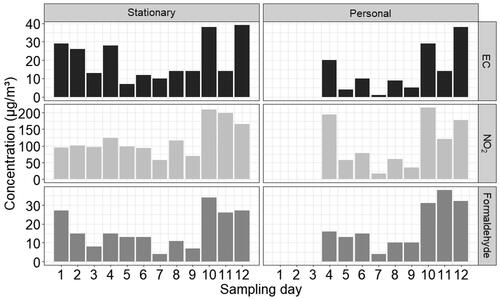
The co-variation of EC, NO2, and formaldehyde within both the personal and stationary data sets indicate a similar main source or sources. These associations were tested, yielding Pearson’s correlation coefficients for EC to NO2 of r = 0.74, p-value = 0.0001, and r = 0.69, p-value = 0.0006 for EC to formaldehyde, respectively. The association between NO2 and formaldehyde had a Pearson’s correlation coefficient of r = 0.75, p-value = 0.0001. Further investigation on the potential health effects of these additive exposures is outside the scope of this manuscript.
Methylcyclohexane was detected in the characterization samples of diesel engine and helicopter exhaust (). Although the correlations are weak, a significant association between methylcyclohexane and EC (r = 0.55, p-value = 0.01) as well as to NO2 (r = 0.55, p-value = 0.009) was found. The alkanes detected in the characterization samples taken from the diesel engine, generator, as well as from the helicopter exhaust, did, on the other hand, not correlate significantly to either EC or NO2.
A summary of the concentrations measured in the breathing zone of the helicopter deck operator and at the stationary sampling site on the helicopter deck during the campaign is presented in . A relatively high number of samples were below LOD for different VOCs, e.g., BTEX, thus the table only presents the compounds above LOD.
Table 3. A summary of the exposure to and concentration of compounds measured on helicopter deck during working hours over 9 to 12 days (n).
Discussion
The characterization performed in this study shows that DE from the main engine and diesel generators differed from that of the helicopter and gas turbine engine emissions in terms of their concentration of EC, NO2, formaldehyde, and VOCs. Gas turbines are the lower-emitting source due to their inherent dilution of exhaust technology (Pavri and Moore Citation2001). One interesting finding is that the diesel main engine emits a higher concentration of NO2, whereas the generator emits the highest concentration of formaldehyde. Our study shows that the exhaust includes both volatiles and semi-volatile organic compounds which are known irritants, in addition to several PAHs which are reported from previous studies of ships emission (Moldanová et al. Citation2009). Further research with analytical methods specifically targeted to SVOCs is needed to confirm our findings.
The potential exposure to DE varied over the sampling campaign. In general, the concentrations, measured in the breathing zones of the helicopter deck operators and at the stationary sampling site on the helicopter deck were low compared to the current European OEL for all measured compounds, except for EC. In some of the work shifts, EC concentrations were close to the current European OEL (50 µg/m3) indicating significant worker exposure on the helicopter deck. A significant correlation was found between EC and NO2, indicating a similar source or sources of exposure. CO is known to be present in the atmosphere in trace concentrations up to 0.1 ppm (Badr and Probert Citation1994; Park and Rhee Citation2016), and the concentrations measured stationary at the stern of the ship reflect the influence of environmental background sources, besides the engine exhaust.
Characterization of exhaust by engine type
Characterization of DE from the four different types of engines demonstrates that the exhaust from the main diesel engines had the highest concentration of NO2, about three times higher than the sampled diesel generator, while the gas turbine and helicopter exhausts only contained negligible amounts. Conversely, the concentration of EC in the samples from diesel generator exhaust is about double of the ship engine exhaust, while EC was not detected in exhaust samples from the gas turbine and helicopter. Overall, our findings indicate that exhaust from the main diesel engines is potentially the main gaseous contributor, while the generator might be the predominant contributor of PM. In contrast, the gas turbine and helicopter contributed with very little NO2, EC, and VOCs.
Non-targeted screening analysis of the four different exhaust sources points to the generator being the main contributor of the irritants 2,5-difenyl-p-benzoquinone, cinnoline, formaldehyde, and phthalic anhydride. Previous reports of quinones in diesel vehicular emissions are published (Cho et al. Citation2004; Jakober et al. Citation2007), however, the irritant properties of these compounds point to the need for further research on exposure to seafarers. The diesel engine and generator are the dominant sources of formaldehyde and phenol on board, with the generator emitting formaldehyde at a six times higher factor than the ship engine, and almost double the amount of phenol. Only small amounts of these compounds were detected in the helicopter and gas turbine exhausts. Moreover, phenol, a common irritant from fossil fuel combustion, was also detected with TD-GC-MS in all the exhausts except from the gas turbine, with the diesel generator being the principal emitter. However, deviations between the sampling points of the gas turbine and the helicopter and the effects of dilution among the different engine technologies might have affected the concentration of the exhausts, and thus the detection of compounds.
A quantification of VOCs in the different DE is outside the scope of this work but has been vastly covered in the published literature for HFO (Moldanová et al. Citation2009; Sippula et al. Citation2014) and MDO (Moldanová et al. Citation2009; Chu Van et al. Citation2019; McCaffery et al. Citation2021). Still, characterization of DE performed in this work confirmed the presence of several PAH compounds, BTEX, and heterocyclic hydrocarbons as previously reported by others (Langer et al. Citation2020; Zhang et al. Citation2021).
The main morphology reported from DE is soot particles, which are agglomerates or aggregates of particles with an onion shell-like structure (Rana et al. Citation2022). This is consistent with what we have observed in the characterized DE. Some authors have reported carbon nanotubes found in DE particles (Uchida et al. Citation2006; Jung et al. Citation2013) however, no carbon nanotubes were observed among the particles collected from the four different diesel engines in this study.
Exposure assessment
The measured exposure during work on the helicopter deck was below European OEL for the DE gases (European Chemicals Agency Citation2022a), and well below existing European OELs for NO2 and the VOCs. Nevertheless, the median concentration of NO2 was much higher in this study (personal: 0.08 and stationary: 0.1 mg/m3) compared to what was measured over one week on the bow of an icebreaker tested with two different fuel types (HFO: 7.4 µg/m3, MDO: 1.4 µg/m3) (Langer et al. Citation2020). On the other hand, when operated on HFO diesel, concentrations of formaldehyde on the deck of the icebreaker were higher than the concentrations measured in this study Concentrations of EC measured on the helicopter deck were, for some days, significant with values close to the European OEL of 50 µg/m3 but above the State of California OEL of 20 µg/m3a as a time-weighted average (TWA) (California Department of Public Health Citation2002). The EC concentrations (geometric mean GM: 8.5 µg/m3) were higher on the helicopter deck than what was reported of gate controllers at the port of Montreal (GM: 1.6 µg/m3) (Debia et al. Citation2016), and on deck by Langer and coworkers (2020), independent from the fuel type used in their study (Langer et al. Citation2020). However, direct comparison with the Langer et al. (Citation2020) study is limited by the fact that the sampling strategy was very different, e.g., compounds were sampled on two opposite sides of the ships, as well as at different heights. Generally, higher concentrations of all compounds except EC were identified inside the icebreaker, the engine room, and personal spaces. Indoor measurements were not the focus of this study.
The correlation between EC and NO2 was strong and significant in this study (Pearson’s correlation coefficients of r = 0.74, p-value = 0.0001). This finding is consistent with reports in underground mining and road tunnel finishing, in which pollutants originate from diesel-powered engines (Berlinger et al. Citation2019). Although there are many offshore scenarios where several multiple of DE are present (e.g., when supply ships are near oil rigs, in highly trafficked waters, or close to land), a significant correlation between EC and NO2 may not occur in these situations.
Study limitations
The non-targeted screening analysis performed in this study detected several compounds present in the different exhausts. Sampling with Tenax packed TD tubes is, however, not the optimal method for each of the identified compounds. These tubes were nevertheless chosen for sampling VOCs for the non-targeted analysis since these allow for screening of a broad range of VOCs with boiling points between 60 and 250 °C. Further research is needed to validate the presence of some of the VOCs/SVOCs detected in the exhaust, such as 2,5-difenyl-p-benzoquinon, cinnoline, phthalic anhydride, and phenyl maleic anhydride. Validation and quantification of these compounds are important because these are known irritants, and an additive effect may therefore be of concern.
Due to the different engine technologies, combustion exhaust emissions at the stacks were subjected to variable degrees of dilution, which was not accounted for in this study. Therefore, future studies characterizing the exhausts at multiple sources should consider including additional measurements of, for instance, CO and CO2 (Fleming et al. Citation1965), allowing for the assessment of differences in dilution between sampling points.
The exposure assessment in this study is limited to relatively few personal measurements. Still, the results show that the personal exposure and stationary samples were comparable, implying that the concentrations measured on the helicopter deck may represent workers’ exposures. One aim of this study was to understand daily variations, as this is often not included in occupational hygiene measurements offshore. Our results show that the occupational exposures on the helicopter deck were highly variable (e.g., ranging from ECmin = 0.5 to ECmax = 37 µg/m3). This variability is an important consideration when conducting offshore exposure assessments. Further research is needed to elucidate the extent of DE exposure to seafarers.
Conclusions
This study shows that DE from the ship’s main diesel engine and generators were the major contributors to the presence of EC, NO2, and VOCs on the helicopter deck. However, the measured concentration in the breathing zone of the workers to these compounds was low for all, except for EC. For some days, EC concentrations were close to the current occupational exposure limit while almost negligible on other days. The high variation in EC should be considered when planning future studies of DE exposure in offshore operations. Several irritants were detected in the exhausts with non-targeted screening analysis, and are likely to be associated with the presence of diesel PM. More research is needed to quantify these irritants and to elucidate a possible additive effect in this work environment.
uoeh_a_2180150_sm8433.docx
Download MS Word (35.3 KB)Acknowledgments
We are grateful to the crew on board the frigate for assistance with the sampling and logistics. The authors would especially like to thank Anette Borge Malmo and Aksel Sveum from the Royal Norwegian Air Force for their contribution to facilitating the study and assisting with the sampling.
Data availability statement
Data that support the findings of this study are available from the corresponding author, E.Z., upon reasonable request.
Additional information
Funding
References
- Badr O, Probert SD. 1994. Carbon monoxide concentration in the Earth’s atmosphere. Appl Energy. 49(2):99–143. DOI: 10.1016/0306-2619(94)90035-3.
- Bendtsen KM, Bengtsen E, Saber AT, Vogel U. 2021. A review of health effects associated with exposure to jet engine emissions in and around airports. Environ Health. 20(1):10. DOI: 10.1186/s12940-020-00690-y.
- Berlinger B, Ellingsen DG, Romanova N, Friisk G, Daae HL, Weinbruch S, Skaugset NP, Thomassen Y. 2019. Elemental carbon and nitrogen dioxide as markers of exposure to diesel exhaust in selected Norwegian industries. Ann Work Expo Health. 63(3):349–358. DOI: 10.1093/annweh/wxy112.
- Birch ME. 2002. Occupational monitoring of particulate diesel exhaust by NIOSH method 5040. Appl Occup Environ Hyg. 17(6):400–405. DOI: 10.1080/10473220290035390.
- Birch ME, Cary RA. 1996. Elemental carbon-based method for monitoring occupational exposures to particulate diesel exhaust. Aerosol Sci Technol. 25(3):221–241. DOI: 10.1080/02786829608965393.
- Bondi KR, Shea ML, DeBell RM. 1983. Nitrogen dioxide levels aboard nuclear submarines. Am Ind Hyg Assoc J. 44(11):828–832. DOI: 10.1080/15298668391405814.
- Brinchmann BC, Holme JA, Frerker N, Rambøl MH, Karlsen T, Brinchmann JE, Kubátová A, Kukowski K, Skuland T, Øvrevik J. 2023. Effects of organic chemicals from diesel exhaust particles on adipocytes differentiated from human mesenchymal stem cells. Basic Clin Pharmacol Toxicol. 132(1):83–97. DOI: 10.1111/bcpt.13805.
- California Department of Public Health. 2002. Health Hazard Advisory: diesel engine exhaust (PDF). January 2023; [accessed 2023 Feb 3]. https://www.cdph.ca.gov/Programs/CCDPHP/DEODC/OHB/HESIS/CDPH%20Document%20Library/diesel.pdf.
- Carslaw DC, Ropkins K. 2012. Openair – an R package for air quality data analysis. Environ Model Software. 27-28:52–61. DOI: 10.1016/j.envsoft.2011.09.008.
- Cernansky NP. 1983. Diesel exhaust odor and irritants: a review. J Air Pollut Control Assoc. 33(2):97–104. DOI: 10.1080/00022470.1983.10465560.
- Cho AK, Di Stefano E, You Y, Rodriguez CE, Schmitz DA, Kumagai Y, Miguel AH, Eiguren-Fernandez A, Kobayashi T, Avol E, et al. 2004. Determination of four quinones in diesel exhaust particles, SRM 1649a, and atmospheric PM2.5 special issue of Aerosol Science and Technology on findings from the fine particulate matter supersites program. Aerosol Sci Technol. 38(sup1):68–81. DOI: 10.1080/02786820390229471.
- Chu Van T, Ramirez J, Rainey T, Ristovski Z, Brown RJ. 2019. Global impacts of recent IMO regulations on marine fuel oil refining processes and ship emissions. Transp Res D Transp Environ. 70:123–134. DOI: 10.1016/j.trd.2019.04.001.
- Cooper DA. 2001. Exhaust emissions from high speed passenger ferries. Atmos Environ. 35(24):4189–4200. DOI: 10.1016/S1352-2310(01)00192-3.
- Cooper DA. 2003. Exhaust emissions from ships at berth. Atmos Environ. 37(27):3817–3830. DOI: 10.1016/S1352-2310(03)00446-1.
- Debia M, Neesham-Grenon E, Mudaheranwa OC, Ragettli MS. 2016. Diesel exhaust exposures in port workers. J Occup Environ Hyg. 13(7):549–557. DOI: 10.1080/15459624.2016.1153802.
- European Chemicals Agency. 2022a. Diesel fuel combustion products, vapor. Occupational Exposure Limits OELs. ECHA substance information; [accessed 2022 Sept 12]. https://echa.europa.eu/substance-information/-/substanceinfo/100.305.408#REGULATORY_NAMEScontainer.
- European Chemicals Agency. 2022b. Substance Infocard 2,5-diphenyl-p-benzoquinone. ECHA substance information; [accessed 2022 Sept 12]. https://echa.europa.eu/substance-information/-/substanceinfo/100.011.528.
- Fleming RD, Dimitriades B, Hurn RW. 1965. Procedures in sampling and handling auto exhaust. J Air Pollut Control Assoc. 15(8):371–374. DOI: 10.1080/00022470.1965.10468394.
- Gan TH, Hanhela P, Mazurek W, Gillett R. 2010. Characteristics of submarine engine diesel particulates in the maritime environment. J Aerosol Sci. 41(1):23–35. DOI: 10.1016/j.jaerosci.2009.09.007.
- Hedmer M, Wierzbicka A, Li H, Albin M, Tinnerberg H, Broberg K. 2017. Diesel exhaust exposure assessment among tunnel construction workers – correlations between nitrogen dioxide, respirable elemental carbon, and particle number. Ann Work Expo Health. 61(5):539–553. DOI: 10.1093/annweh/wxx024.
- Holme JA, Brinchmann BC, Refsnes M, Låg M, Øvrevik J. 2019. Potential role of polycyclic aromatic hydrocarbons as mediators of cardiovascular effects from combustion particles. Environ Health. 18(1):74. DOI: 10.1186/s12940-019-0514-2.
- IARC. 2012. Diesel and gasoline engine exhausts and some nitroarenes. Lyon (France): International Agency for Research on Cancer; [accessed 2022 Aug 20]. https://publications.iarc.fr/_publications/media/download/3181/e6bd0692f1a9bb46589d3ca2d8178fa8dcd05ba5.pdf.
- ISO/IEC. 2017. ISO 17025:2017 General requirements for the competence of testing and calibration laboratories. In: ISO/CASCO Committee on conformity assessment, editor: International Organization for Standardization ISO/International Electrotechnical Commission IEC.
- Jakober CA, Riddle SG, Robert MA, Destaillats H, Charles MJ, Green PG, Kleeman MJ. 2007. Quinone emissions from gasoline and diesel motor vehicles. Environ Sci Technol. 41(13):4548–4554. DOI: 10.1021/es062967u.
- Joshi DA. 2019. An overview on common organic solvents and their toxicity. J Pharm Res Int. 28(3):1–18. DOI: 10.9734/jpri/2019/v28i330203.
- Jung HS, Miller A, Park K, Kittelson DB. 2013. Carbon nanotubes among diesel exhaust particles: real samples or contaminants? J Air Waste Manag Assoc. 63(10):1199–1204. DOI: 10.1080/10962247.2013.812048.
- Kirrane E, Loomis D, Egeghy P, Nylander-French L. 2007. Personal exposure to benzene from fuel emissions among commercial fishers: comparison of two-stroke, four-stroke and diesel engines. J Expo Sci Environ Epidemiol. 17(2):151–158. DOI: 10.1038/sj.jes.7500487.
- Langer S, Österman C, Strandberg B, Moldanová J, Fridén H. 2020. Impacts of fuel quality on indoor environment onboard a ship: from policy to practice. Transp Res D Transp Environ. 83:102352. DOI: 10.1016/j.trd.2020.102352.
- Lighty JS, Veranth JM, Sarofim AF. 2000. Combustion aerosols: factors governing their size and composition and implications to human health. J Air Waste Manag Assoc. 50(9):1565–1618. DOI: 10.1080/10473289.2000.10464197.
- Lloyd AC, Cackette TA. 2001. Diesel engines: environmental impact and control. J Air Waste Manag Assoc. 51(6):809–847. DOI: 10.1080/10473289.2001.10464315.
- Mazaheri M, Johnson GR, Morawska L. 2011. An inventory of particle and gaseous emissions from large aircraft thrust engine operations at an airport. Atmos Environ. 45(20):3500–3507. DOI: 10.1016/j.atmosenv.2010.12.012.
- McCaffery C, Zhu H, Karavalakis G, Durbin TD, Miller JW, Johnson KC. 2021. Sources of air pollutants from a Tier 2 ocean-going container vessel: main engine, auxiliary engine, and auxiliary boiler. Atmos Environ. 245:118023. DOI: 10.1016/j.atmosenv.2020.118023.
- McDonald JD, Barr EB, White RK, Chow JC, Schauer JJ, Zielinska B, Grosjean E. 2004. Generation and characterization of four dilutions of diesel engine exhaust for a subchronic inhalation study. Environ Sci Technol. 38(9):2513–2522. DOI: 10.1021/es035024v.
- Methner MM, Boudreau AY. 2006. NIOSH Health Hazard Evaluation Report, No. HETA-2003-0246-3013, Joint Pacific Marine Safety Code Committee. San Francisco (CA): U.S. Department of Health and Human Services, Public Health Service, Centers for Disease Control and Prevention, National Institute for Occupational Safety and Health.
- Millard SP. 2013. EnvStats: an R package for environmental statistics. New York (NY): Springer. https://link.springer.com/book/10.1007/978-1-4614-8456-1.
- Moldanová J, Fridell E, Popovicheva O, Demirdjian B, Tishkova V, Faccinetto A, Focsa C. 2009. Characterisation of particulate matter and gaseous emissions from a large ship diesel engine. Atmos Environ. 43(16):2632–2641. DOI: 10.1016/j.atmosenv.2009.02.008.
- Moldanová J, Fridell E, Winnes H, Holmin-Fridell S, Boman J, Jedynska A, Tishkova V, Demirdjian B, Joulie S, Bladt H, et al. 2013. Physical and chemical characterisation of PM emissions from two ships operating in European emission control areas. Atmos Meas Tech. 6(12):3577–3596. DOI: 10.5194/amt-6-3577-2013.
- NIOSH. 2016a. Method 1500 hydrocarbons, BP 36°-216 °C. National Institute for Occupational Safety and Health; [accessed 2023 Feb 3]. https://www.cdc.gov/niosh/docs/2003-154/method-b.html.
- NIOSH. 2016b. Method 1501 hydrocarbons, aromatic. National Institute for Occupational Safety and Health; [accessed 2023 Feb 3]. https://www.cdc.gov/niosh/docs/2003-154/pdfs/1501.pdf.
- NIOSH 2016c. Method 5040, issue 4 diesel particulate matter (as elemental carbon). National Institute for Occupational Safety and Health; [accessed 2023 Feb 3]. https://www.cdc.gov/niosh/docs/2014-151/pdfs/methods/5040.pdf.
- Noskov A, Thomassen Y, Berlinger B, Olsen R, Ervik TK, Weinbruch S, Gilmutdinov A. 2019. Characterization of air contaminants emitted during laser cutting of carbon fiber-reinforced composite materials. Anal Bioanal Chem. 411(2):305–313. DOI: 10.1007/s00216-018-1469-9.
- OSHA. 2021. Method no. 32 phenol and cresol. Occupational Safety and Health Administration, United States Department of Labor; [accessed 2023 Feb 3]. https://www.osha.gov/sites/default/files/methods/osha32.pdf.
- Park K, Rhee TS. 2016. Oceanic source strength of carbon monoxide on the basis of basin-wide observations in the Atlantic. Environ Sci Process Impacts. 18(1):104–114. DOI: 10.1039/C5EM00546A.
- Pavri R, Moore GD. 2001. Gas turbine emissions and control. GE Energy Services. GER-4211 (03/01); [accessed 2023 Feb 3]. https://www.ge.com/content/dam/gepower-new/global/en_US/downloads/gas-new-site/resources/reference/ger-4211-gas-turbine-emissions-and-control.pdf.
- Perrone MG, Carbone C, Faedo D, Ferrero L, Maggioni A, Sangiorgi G, Bolzacchini E. 2014. Exhaust emissions of polycyclic aromatic hydrocarbons, n-alkanes and phenols from vehicles coming within different European classes. Atmos Environ. 82:391–400. DOI: 10.1016/j.atmosenv.2013.10.040.
- Pronk A, Coble J, Stewart PA. 2009. Occupational exposure to diesel engine exhaust: a literature review. J Expo Sci Environ Epidemiol. 19(5):443–457. DOI: 10.1038/jes.2009.21.
- PubChem. 2022a. Compound summary for CID 7501, Styrene. National Center for Biotechnology Information; [accessed 2023 Feb 3]. https://pubchem.ncbi.nlm.nih.gov/compound/7501.
- PubChem. 2022b. Compound summary for CID 9208, Cinnoline; [accessed 2023 Feb 3]. https://pubchem.ncbi.nlm.nih.gov/compound/9208.
- PubChem. 2022c. Phthalic anhydride – laboratory chemical safety summary (LCSS). National Institutes of Health NIH; [accessed 2023 Feb 3]. https://pubchem.ncbi.nlm.nih.gov/compound/Phthalic-anhydride#datasheet=LCSS.
- Rana S, Saxena MR, Maurya RK. 2022. A review on morphology, nanostructure, chemical composition, and number concentration of diesel particulate emissions. Environ Sci Pollut Res Int. 29(11):15432–15489. DOI: 10.1007/s11356-021-15999-5.
- Reda AA, Schnelle-Kreis J, Orasche J, Abbaszade G, Lintelmann J, Arteaga-Salas JM, Stengel B, Rabe R, Harndorf H, Sippula O, et al. 2015. Gas phase carbonyl compounds in ship emissions: differences between diesel fuel and heavy fuel oil operation. Atmos Environ. 112:370–380. DOI: 10.1016/j.atmosenv.2015.03.057.
- Ris C. 2007. U.S. EPA health assessment for diesel engine exhaust: a review. Inhal Toxicol. 19(Suppl 1):229–239. DOI: 10.1080/08958370701497960.
- Sippula O, Stengel B, Sklorz M, Streibel T, Rabe R, Orasche J, Lintelmann J, Michalke B, Abbaszade G, Radischat C, et al. 2014. Particle emissions from a marine engine: chemical composition and aromatic emission profiles under various operating conditions. Environ Sci Technol. 48(19):11721–11729. DOI: 10.1021/es502484z.
- Steiner S, Bisig C, Petri-Fink A, Rothen-Rutishauser B. 2016. Diesel exhaust: current knowledge of adverse effects and underlying cellular mechanisms. Arch Toxicol. 90(7):1541–1553. DOI: 10.1007/s00204-016-1736-5.
- Taxell P, Santonen T. 2017. Diesel engine exhaust: basis for occupational exposure limit value. Toxicol Sci. 158(2):243–251. DOI: 10.1093/toxsci/kfx110.
- U.S. EPA. 2002. Health Assessment Document for diesel engine exhaust (Final 2002); [accessed 2023 Feb 3]. EPA/600/8-90/057F. https://cfpub.epa.gov/ncea/risk/recordisplay.cfm?deid=29060.
- Uchida T, Ohashi O, Kawamoto H, Yoshimura H, Kobayashi K-i, Tanimura M, Fujikawa N, Nishimoto T, Awata K, Tachibana M, et al. 2006. Synthesis of single-wall carbon nanotubes from diesel soot. Jpn J Appl Phys. 45(10A):8027–8029. DOI: 10.1143/jjap.45.8027.
- Wickham H. 2009. Manipulating data. In: Wickham H, editor. ggplot2: elegant graphics for data analysis. New York (NY): Springer; p. 157–175.
- Zhang F, Chen Y, Su P, Cui M, Han Y, Matthias V, Wang G. 2021. Variations and characteristics of carbonaceous substances emitted from a heavy fuel oil ship engine under different operating loads. Environ Pollut. 284:117388. DOI: 10.1016/j.envpol.2021.117388.
- Zhu Y, Zhou W, Xia C, Hou Q. 2022. Application and development of selective catalytic reduction technology for marine low-speed diesel engine: trade-off among high sulfur fuel, high thermal efficiency, and low pollution emission. Atmosphere. 13(5):731. DOI: 10.3390/atmos13050731.