Abstract
Tissue development is orchestrated by the coordinated activities of both chemical and physical regulators. While much attention has been given to the role that chemical regulators play in driving development, researchers have recently begun to elucidate the important role that the mechanical properties of the extracellular environment play. For instance, the stiffness of the extracellular environment has a role in orienting cell division, maintaining tissue boundaries, directing cell migration, and driving differentiation. In addition, extracellular matrix stiffness is important for maintaining normal tissue homeostasis, and when matrix mechanics become imbalanced, disease progression may ensue. In this article, we will review the important role that matrix stiffness plays in dictating cell behavior during development, tissue homeostasis, and disease progression.
Abbreviations
ECM | = | Extracellular matrix |
EPC | = | Endothelial progenitor cell |
FA | = | Focal adhesion |
FAK | = | Focal adhesion kinase |
LOX | = | Lysyl oxidase |
MKL1 | = | Megakaryoblastic leukemia factor-1 |
MMP | = | Matrix metalloproteinase |
MSC | = | Mesenchymal stem cell |
ROCK | = | Rho-associated protein kinase |
VSMC | = | Vascular smooth muscle cell |
Introduction
Tissue engineering approaches aim to restore normal physiologic function to damaged or diseased tissues through a specifically tailored combination of cells, extracellular matrices (ECM), soluble factors, and/or mechanical stimulation. Historically, there has been a significant emphasis placed on the role of chemical regulators (ie. growth factors, bioactive ECM moieties, etc.) within the extracellular environment, and further, numerous investigations have confirmed that surface chemistry is a critical parameter contributing to the clinical success of implanted biomaterials and devices. However, only recently have researchers begun to unravel the important role that the mechanical properties of the ECM plays.
In vivo, cells reside in a complex yet highly organized environment containing a diverse collection of soluble and insoluble proteins, sugars, and other cells, and the exact composition and spatial orientation of a cell's microenvironment dictates the local mechanical environment that a cell is exposed to. The mechanical environment is composed of endogenous forces generated by the cells themselves, as well as exogenous forces that are applied to cells by the surrounding microenvironment. Endogenous forces are largely produced by cytoskeletal contractility within cells,Citation1,2 whereas exogenous forces exist in a variety of forms, including gravity, shear stress, and tensile and compressive forces. Cells receive these exogenous forces through interaction with the ECM, and the local stiffness of the ECM is an important mechanical effector of cell behavior.
Interestingly, external physical forces can shape the mechanical environment and make it a continually evolving and dynamic microenvironment for cells. Physical forces have long been known to contribute to tissue development and homeostasis through clinical observations of brain morphology and bone remodeling. For example, external loading is important for tissues like cartilage and bone to maintain the appropriate ECM content and composition. For cartilage and bone, impaired mobility and thus reduced mechanical stimulation leads to a decrease in proteoglycan content and bone mineral density, respectively; both can be increased with exercise. Further, studies probing the effect of weightlessness on the musculoskeletal system during spaceflight have found that without the physical forces imposed by gravity, bone density is greatly reduced. Without gravity, osteoblasts—the bone-producing cells—do not receive the necessary stimulation that instructs them to synthesize new matrix, and there is thus a net loss of bone mass. The osteoblasts are subsequently exposed to an ECM with altered mechanical properties, illustrating the reciprocal nature of tissue homeostasis. These examples illustrate the fact that external forces greatly impact tissue homeostasis. However, it is important to keep in mind that these clinical observations are the product of reciprocal actions between cells and their ECM. On the one hand, cells are constantly synthesizing, breaking down, or otherwise rearranging ECM components to change ECM composition and topography. On the other hand, the ECM has a fundamentally important role in dictating cell behavior, and as such, any changes in ECM dynamics will influence adjacent cells and influence their cellular activities. By maintaining this feedback loop between cells and their ECM, tissues can become very adaptive to the physical forces imposed on it. However, when tissue homeostasis becomes imbalanced, tissue function generally becomes impaired as a result of aberrant cellular behavior. Thus, it is critical to understand how cells respond to their mechanical environment for the treatment and prevention of a variety of diseases.
Stiffness, or rigidity, of a material is defined as the extent to which a material resists deformation in response to an applied force. Stiffness is used to indicate whether a material is compliant (soft) or rigid (hard). In biology, stiffness has been used to collectively represent mechanical properties of a biological substrate. Our tissues, which are composed of a variety of different ECM molecules, feature a wide range of elastic moduli (), and each tissue/organ has specific stiffness for fulfilling physiological needs. For example, bone is much stiffer than other tissues, for its primary function is to provide structure and protect our internal organs. In this article, we review the role of ECM stiffness on cellular function. We will begin by discussing the important role of ECM mechanics during tissue development and homeostasis, and then expand to discuss the changes that occur to ECM mechanics during the progression of fibrotic diseases and tumor development.
Figure 1. Distinct modulus of human tissues suggesting tissue-specific stiffness. Different tissues with their specific elastic modulus in the body are correlated with their functions (adapted from Cox et al.).Citation98
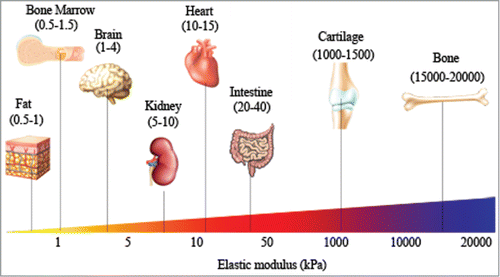
Molecular Regulation by Mechanical Stimulation
The actin cytoskeleton functions to maintain the structural integrity of a cell and contributes to their mechanical properties. It is also involved in cell signaling transduction, thus controlling a variety of cellular responses.Citation3–5 ECM stiffness has been shown to induce actin cytoskeletal reorganization and contractility, thereby controlling adhesion, migration, cell cycle progression, and differentiation.Citation6–8 The translation of ECM stiffness into intracellular signals to change cell behavior is mainly mediated by transmembrane receptors, known as integrins. Integrins are heterodimers consisting of α− and β−subunits with the β-subunit tail linking to the actin cytoskeleton through several different adaptor proteins. There are 18α−subunits and 8 β−subunits identified to date, and they form 24 different αβ heterodimers, with each of them having specificity for different ECM ligands.Citation9 After binding to ECM ligands, integrins cluster to initiate the assembly of adaptor molecules, such as focal adhesion kinase (FAK), vinculin, paxillin, tensin, and talin, for forming focal adhesions between a cell and ECM. With focal adhesions established, actin filaments can then organize into stress fibers to mediate cytoskeletal tension in a cell. In addition, assembling of the focal adhesion complex is able to activate a number of signaling pathways to regulate cell activities.Citation10,11
Stiff matrices increase the number of focal adhesions (FAs) and traction force generated between FAs and the ECM compared to soft ones. A FA complex in a cell functions as a mechanosensor that generates a fluctuating force to pull the ECM, thereby directing cells to migrate to an area with greater ECM stiffness.Citation12 These findings suggest that ECM stiffness is able to regulate FAK activity to trigger subsequent activation of signal molecules to regulate cell activities. For example, Shih et al. demonstrated that stiff matrix enhances FAK activity to increase activation of the downstream molecule ERK1/2, promoting osteogenic differentiation of mesenchymal stem cells (MSCs).Citation13
The Rho/ROCK signaling pathway also plays a crucial role in the regulation of cell-matrix mechanotransduction. It has been shown that Rho activity is regulated by ECM stiffness, with stiff matrices leading to increased RhoA activation, contractility of actin cytoskeleton, and formation of actin stress fibers compared to compliant ones. The Rho family of GTPases consists of more than 20 proteins that induce specific effects to the actin cytoskeleton.Citation14,15 Of these, RhoA, -B, and -C comprise the Rho subgroup of Rho GTPases, which have redundant functions and from here on will be discussed simply with respect to findings regarding RhoA. Other well studied Rho GTPases include Rac1 and Cdc42.
The primary action of RhoA is to induce the formation of actin stress fibers, thick bundles of anti-parallel actin filaments crosslinked by myosin. Rho activities are elicited through downstream activation of specific effector proteins. The two primary downstream effectors of RhoA are Rho-associated protein kinase (ROCK) and mDia. ROCK is a serine/threonine kinase that can phosphorylate a variety of substrates, including the myosin-binding subunit of myosin phosphatase and myosin regulatory light chain, to stimulate crosslinking of actin by myosin and enhance actomyosin contractility,Citation16 whereas mDia catalyzes actin nucleation and polymerization to produce long, straight actin filaments.Citation17 ROCK also phosphorylates and activates LIM-kinase, which in turn phosphorylates and inactivates cofilin—an actin depolymerizing factor—to stabilize existing actin filaments.Citation18 Together, the combined actions of ROCK and mDia downstream of Rho act to induce actomyosin bundle formation within cells. It has been shown that matrix stiffness regulates myofibroblast differentiation via the RhoA/ROCK signaling pathway with matrix stiffening increasing the RhoA activation and ROCK activity and promoting the interaction between RhoA and ROCK.Citation19 Treatment with a RhoA/ROCK inhibitor changes the actin cytoskeleton dynamics and megakaryoblastic leukemia factor-1 (MKL1) nuclear translocation, thus inhibiting myofibroblast differentiation.Citation19
Another member of the Rho family, Rac1, is also involved in the cellular regulation by ECM stiffness. Recently, a study by Bae et al. has shown that the signaling pathway involving FAK, the adaptor protein p130Cas, and Rac plays a critical role in regulating the cell cycle of mouse vascular smooth muscle cells (VSMCs) in response to different matrix stiffness.Citation8 When VSMCs are cultured on a stiff substrate, the Rac1-mediated signaling pathway is activated to enhance the expression of cyclin D and promote entry into the S phase of the cell cycle. In addition, Chaudhuri et al. have demonstrated that in mammary epithelium, mechanical signals induced by ECM stiffness are mediated by the β4 integrin-Rac1-PI3K pathway, and malignant phenotypes of cells are induced when ECM stiffness is increased.Citation20 Together, these studies demonstrate that cells respond to ECM stiffness by reorganizing their actin cytoskeleton through activation of integrins, FA-associated molecules, and the Rho family ().
Figure 2. Molecular regulation by ECM stiffness. Integrins respond to ECM stiffness by clustering together to activate adaptor proteins of focal adhesion and downstream signaling molecules to regulate cell behavior. Previous findings of the regulatory pathways underlying the molecular mechanism induced by ECM stiffness are illustrated as representative examples.
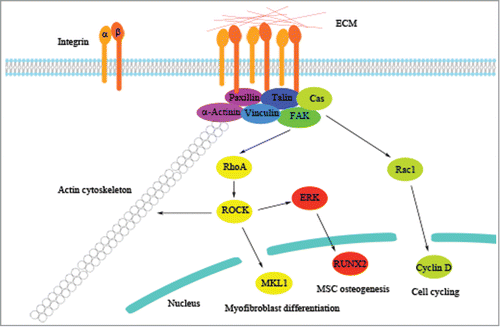
ECM Stiffness Regulates Cellular Behavior
Cell behavior is dictated, in part, by mechanical forces exerted upon the cell, and cells respond to these forces through interaction with the ECM. A cell is in a physical continuum with its surrounding ECM. Using quick freeze, deep-etch electron microscopy—a rapid, chemical fixation-free method that preserves native structure with high fidelity—ECM fibers were shown to radiate orthogonally from the plasma membrane surface and exist as an interconnected unit with the cytoplasmic cytoskeleton through adhesion receptors.Citation21 Thus, external physical forces can be transmitted to cells through the ECM, and ECM stiffness modulates the amplitude of force received. In large part, cells sense the stiffness of their environment by pulling against the ECM using intracellular actomyosin contractility. Together, a model has emerged whereby a cell pulls against the surrounding ECM using Rho-ROCK-mediated actomyosin contraction. If the ECM is stiff and the cell is met with resistance, the forces generated result in tension across the adhesion receptors. This, in turn, results in a strengthening of these adhesions, recruitment of signaling molecules into the focal complexes, and enhanced mechanotransduction. Moreover, a stiff matrix provides enough traction for cell spreading, proliferation, and migration. If the matrix is compliant, the cells will instead deform the matrix and round up, resulting in different signaling and phenotypic outcomes.Citation22 Thus, tissue stiffness has dramatic effects on cellular activities, and this has been observed both during development and throughout the lifespan of adult organisms.
Cellular Response to ECM Stiffness During Embryonic Development
Mechanical properties of the ECM play a vital role throughout the entire course of embryonic development. Even from the earliest stages of development, mechanical forces shape the developing embryo. For instance, upon fertilization of the egg, the orientation of the spindle axis, and thus the orientation of cell divisions, is governed by the spatial distribution of ECM adhesions and thus cytoskeletal tension imposed upon the cells.Citation23 In developing Xenopus embryos, a temporal and spatial distribution of mechanical stiffness directs early patterning and differentiation events, with cells creating these gradients of stiffness through actomyosin contraction.Citation24 Zhou and coworkers found that profound tissue stiffening occurs from the gastrula to neurula stages, and this stiffening had an impact on neural tube formation. By treating tissue explants with latrunculin B, an actin-depolymerizing drug used to reduce actomyosin contraction, the researchers were able to reduce tissue stiffness by more than 50%, suggesting that cell-mediated actomyosin contractility is responsible for much of the tissue stiffening that occurs during this stage of development. Together, the spatial distribution of ECM stiffness is critical for directing early tissue patterning and differentiation events, and cells themselves modulate ECM stiffness through intracellular contractility during early embryogenesis.
Actomyosin-based contractility is similarly important for maintaining boundaries between distinct tissues throughout embryogenesis. In early Drosophila embryos, actomyosin-based contraction results in a stiffening of cell layers that physically restricts mitotic cells from intermixing with neighboring compartments.Citation25 Similarly, patterning of the anteroposterior axis is stabilized by the generation of actomyosin-based tension, which acts to organize and stiffen the ECM in the paraxial somatic mesoderm.Citation24 Tight junctions and adherens junctions mediate the forces exerted between cells to compartmentalize these developing tissues. Additionally, myosin II is enriched at sites of tension application on the surface of intercalating cells,Citation26 suggesting that anisotropy in the micromechanical environment contributes significantly to pattern formation. Later during gastrulation, tissue folding is initiated by a subset of cells that physically constrict their apical membranes and expand their basal domains along the ventral midline of the embryo, resulting in tissue invagination. This apical constriction is regulated by the local accumulation of myosin II at the apical surface of invaginating cells.Citation27 Together, actomyonsin-based contraction plays an important role in tissue compartmentalization, patterning, and invagination.
Changes in cytoskeletal tension and variations in ECM compliance also alter the direction in which cells move and grow, which are crucial cell fate decisions driving tissue patterning during development. In vitro, cells have been shown to migrate preferentially toward stiffer substrates.Citation28 Similarly, gradients of stiffness are generated in vivo to drive tissue patterning. For instance, the basement membrane at the tips of growing epithelial buds in the lung is thinner than the surrounding ECM, creating a gradient in stiffness. In response, the actin cytoskeleton of resident mesenchymal cells shows greater alignment beneath these sites, suggesting that stiffness gradients alter cytoskeletal organization and cellular traction forces in these regions to drive directional budding morphogenesis. Inhibition of cytoskeletal tension using Rho/ROCK antagonists blocks basement membrane thinning at the tips and thus prevents both epithelial budding and branching angiogenesis in the developing lungs,Citation29 suggesting that cells are responsible for creating these stiffness gradients. In the developing mammary gland, branching morphogenesis is likewise characterized by gradients in stiffness. The terminal end buds of branching mammary ducts consist of a basement membrane devoid of collagen type I, whereas collagen deposition is abundant in the flanking regions, creating reduced stiffness in the terminal end buds.Citation30 This stiffness gradient drives proliferation and subsequent ductal elongation, and interestingly, implantation of a pellet containing exogenous TGFβ at the tip of growing ducts induces collagen deposition and inhibits elongation.Citation31 Thus, the ability to generate spatial variations in mechanical tension between endothelial cells and the underlying basement membrane plays an important role in the establishment of local migration tracks and tissue patterning events during branching morphogenesis.Citation32
Several other studies have demonstrated that simply by disrupting ECM structure or composition, tissue development can be greatly affected. For example, ECM mechanics and geometry have been shown to be critical for epithelial cell differentiation,Citation33 and proper ECM composition and elasticity are required for maintenance of the differentiated state of mammary epithelial cells, as ECM stiffening and loss of laminin signaling were found to reduce mammary epithelial cell-specific gene expression.Citation34 Further, a complete loss or disruption of ECM structure leads to mammary gland regressionCitation35 and the involution of capillary blood vessels,Citation36 demonstrating the importance of ECM structure for cellular differentiation and tissue development. Some tissues have unique mechanisms for altering their mechanics throughout morphogenesis. For example, the heart alters its stiffness throughout tissue development by changing the isoform ratios of the cytoskeleton protein, titin, which possesses elastic properties that influence the stiffness of heart tissue.Citation37 Mechanical strain induces the unfolding of the C-terminal kinase domain of titin, which leads to exposure of an ATP-binding site for autophosphorylation. In so doing, titinis believed to work as a strain-sensing molecule for force adaptation in muscle.Citation38 Thus, embryonic organs appear to adapt their material properties in response to changes in the physical forces that occur during each developmental stage.
Similarly, exogenous mechanical forces like hydrostatic pressure and shear stress are important regulators of embryogenesis and have been shown to play a critical role in the development and patterning of many organs. In fact, cells residing in virtually all developing organs, including muscle,Citation39 brain,Citation40,41 kidneys,Citation42,43 and the haematopoietic system,Citation44,45 are sensitive to exogenous mechanical cues. For example, the heart starts pumping blood in Xenopus embryos using a hydrostatic impedance pumping mechanism in which waves of contraction and elastic deformation of the heart chamber create dynamic suction forces that drive blood flow even before valve formation,Citation46 and disruption of myosin-based tension generation during the initial stages of heart looping disrupts morphogenesis,Citation47 demonstrating the important interplay between these exogenous mechanical forces and actomyosin contractility in developing organs. Subsequently, shear stress generated by blood flow from the heart is critical for blood vessel cell fate determination, as evidenced by the activation of arterial markers in endothelial cells of the chick yolk sac.Citation48 Similarly, increased amniotic fluid pressure accelerates lung maturation in rats,Citation49 but if cytoskeletal tension is too high in cells of the developing lung, lung expansion is disrupted,Citation29 further demonstrating the important interplay between exogenous mechanical forces and actomyosin contractility during morphogenesis.
Cellular Response to ECM Stiffness During Tissue Homeostasis
In adult tissues, normal physiology is maintained, in part, through continual tissue remodeling, a process called homeostasis. Tissue homeostasis involves the replacement of older cells with more youthful cells by stem cells and is regulated by the physical and chemical properties of the matrix microenvironment. For instance, it has long been known that bone undergoes continual resorption and formation, and these 2 opposing processes are finely balanced to control normal bone density. Bone formation from MSCs is affected by matrix stiffness: MSC osteogenesis is enhanced with matrix stiffness. Further, previous studies have shown that the MAPK/ERK signaling pathway is increasingly activated to enhance the expression of the bone-associated transcription factor, RUNX2, by stiff matrix compared to that by soft matrix.Citation50,51
The ability of bone to change its structure and adapt to mechanical loads implies that mechanical forces can regulate the behavior of osteoblasts and osteoclasts, the cells responsible for forming and breaking down bone, respectively. Specifically, increased loads have been shown to increase formation and decrease resorption, whereas immobilization has been shown to have the opposite effect.Citation52 Of course, these loads are received by cells through interaction with the bone matrix. Similarly, chondrocytes interact with a highly ECM-rich environment in cartilage to achieve mechanical and metabolic homeostasis even under large compression loads.Citation53 Thus, normal ECM elasticity invariably plays an important role in tissue homeostasis and function by transducing the mechanical load to the cells.
Endothelial progenitor cells (EPCs) are a key cell type for vascular repair and neovascularization.Citation54 During the repair process, EPCs escape from their niche by producing matrix metalloproteinases (MMPs) that digest the ECM and migrate to a target site to rebuild the tissue. Therefore, EPC behavior is greatly regulated by chemical and physical properties of their surrounding ECM.Citation55 Hanjaya-Putra et al. have demonstrated that soft matrix reduces the MMP production by EPCs while increasing cell elongation and extending intracellular vacuoles to open lumen compartments through a signaling pathway involving the activation of the Rho GTPase Cdc42.Citation56
Not surprisingly, when normal tissue homeostasis becomes imbalanced, as can occur during tissue injury, tissue function can become impaired. Interestingly, a prominent feature of many injured tissues is altered tissue mechanics. During the normal wound healing process, it is very common for the ECM of damaged tissues to be replaced by fibrous tissue that is stiffer than the original ECM.Citation57 The newly formed tissue of increased stiffness is generally referred to as scar tissue. Matrix stiffening in scar tissue tends to be the product of abnormal and/or increased ECM deposition, increased ECM crosslinking, and reduced or abnormal matrix degradation. In some areas of the body, such as the skin, scar formation does not typically impair normal tissue function. However, changes in matrix stiffness upon wound healing often perturb normal cellular behavior in other parts of the body. For instance, scarring of the central nervous system can severely impair tissue function.Citation58 Within the brain and spinal cord, tissue injury leads to glial scar formation, which acts as a mechanical barrier and inhibits signal transduction.Citation59 Glial scar maturation has been shown to be associated with increased fibronectin deposition, which inhibited axonal growth and healing.Citation60 Concomitantly, increased fibronectin deposition promoted astrocyte attachment, which played a role in physically separating the injury site from surrounding tissues.Citation61 Thus, tissue mechanics are critical for normal cellular activities, and when disrupted, tissue function is often impaired.
Moreover, aging is another factor that may disrupt tissue homeostasis to alter tissue mechanical properties. For example, aging reduces stiffness of the soft tissue around the hip region, which results in increased risk of hip fracture in the elderly.Citation62 Another example is that the ECM structure of muscles in older people becomes stiffer due to increased density of highly crosslinked collagen than that in younger people.Citation63 Aging also affects stiffness of the blood vessel wall. A previous study by Choi et al. has shown that using hydrogels to mimic the stiffness of young and aging intima demonstrates that the aging process increases the permeability of an endothelial cell monolayer and disrupts cell-cell junctions by activating the Rho-dependent signaling pathway to upregulate cell contractility.Citation64
Cellular Response to ECM Stiffness During Disease Progression
Over time, homeostatic mechanisms are typically able to restore normal tissue mechanics and thus restore proper tissue function. However, prolonged changes in ECM mechanics can result in the development and progression of disease state.Citation49,50 The ECM is essential for normal wound healing processes, but excessive matrix deposition, as is observed with fibrotic diseases, can lead to organ dysfunction. Fibrotic diseases, which include pulmonary fibrosis, systemic sclerosis, liver cirrhosis, and cardiovascular disease, are typically characterized by the hyperproliferation of fibroblasts, their differentiation into myofibroblasts, and excessive ECM synthesis and secretion. Constitutive activation of collagen-secreting myofibroblast-like cells leads to increases in both collagen amount and concentration, which throws off normal tissue homeostasis to more heavily favor production and leads to tissue stiffening. This imbalance is often exacerbated by deregulated expression of MMPs—which act to degrade ECM—and TIMPs—which inhibit the activity of MMPs. Specifically, MMP expression is often downregulated and TIMP expression becomes upregulated, resulting in incomplete matrix remodeling and irreversible fibrosis.Citation65 This imbalance, in turn, compromises normal tissue function and drives disease progression. In addition, variations in ECM composition likewise have a profound effect on the biomechanical properties of a tissue.Citation66 For instance, collagen and elastin fibers are the main structural components in pulmonary connective tissue, but their elastic properties are substantially different. The elastic modulus of collagen fibrils is in the range of 1200 MPa, whereas the elastic modulus of elastin is closer to 1 MPa.Citation67,68 In the lung, they form a continuous network that allows for passive recoil during expiration. However, during pulmonary fibrosis, an increase in collagen content leads to a stiffening of the tissue, which subsequently results in progressive dyspnea (ie. shortness of breath).Citation69 Thus, increased matrix deposition and altered composition results in altered tissue mechanics that alter cellular behavior and impair tissue function.
Pronounced changes in ECM remodeling, which in many ways mimics what is observed in fibrotic diseases, play a crucial role in tumor progression, as well. Tumor development is a dynamic and progressive process that involves both cellular and environmental cues. This is most evident in the fact that the tumor microenvironment is continuously remodeled, with increased stiffness being one of the hallmark features associated with tumor development. In fact, breast cancer tissue can be up to 10-fold stiffer than normal breast tissue (1.5 kPa and 150 Pa, respectively).Citation70,71 Further, malignant breast tumors tend to appear stiffer than benign tumors based on elastography, with a halo of stiffer tissue frequently observed at the tumor margin of invasive tumors. Increased matrix stiffness and ECM remodeling were observed in pre-malignant tissue, as well, and this increase was shown to contribute to malignant transformation in the breast,Citation72 suggesting that changes in ECM stiffness occur early and act to promote future disease progression.
The majority of increased stiffness that arises in the tumor microenvironment occurs as a result of increased ECM deposition—termed desmoplasia—and altered ECM composition, with collagen type I and fibronectin being the most abundant ECM components deposited in cancer.Citation73 Fibronectin, in particular, possesses several physical attributes that enable it to have significant effects on local ECM stiffness. Fibronectin fibrils incorporate directly into the ECM through specific heparin, fibrin, and collagen binding domains, and it has been shown that in vivo fibrillar collagen formation is dependent on binding and subsequent conformational changes in fibronectin.Citation74 This increases the percentage of linear fibers, which leads to a reduction in elasticity at higher fibronectin concentrations.Citation75 Thus, fibronectin directly modifies the mechanical and structural properties of collagen fibers. In addition to collagen type I, collagens type II, III, V, and IX all show increased deposition during tumor formation.Citation76 Increased collagen type V deposition, in particular, is mechanically significant, as it changes the structural organization of collagen type I fibrils.Citation77 Further, heparan sulfate proteoglycans are frequently overexpressed in cancer.Citation78,79 Overall, this results in the formation of a dense, fibrous tissue surrounding malignant, but generally not benign, tumors.Citation71 Concomitantly, this increased stiffness results in altered cellular behavior. For instance, mammary cells in a stiff matrix are more proliferative,Citation80 upregulating proliferation-associated genes like cyclin D1,Citation81 and human breast carcinoma cells show an upregulation of an entire set of genes related to proliferation.Citation82 In contrast, mammary cells within compliant matrices demonstrate growth control, organization of glandular architecture, and express proteins signifying a more differentiated phenotype.Citation34,83,84 Together, matrix stiffening induces a shift in cell fate decision from differentiation to proliferation, which ultimately could lead to activation of oncogenes and tumor progression.
Increased collagen content and tumor stiffness may also be a product of reduced remodeling. MMPs are well established as having a major role in degrading ECM components during normal tissue remodeling and wound healing. However, their expression is deregulated in many tumors. Indeed, their expression is often highly upregulated, and the sustained presence of these proteases, coupled with increased ECM synthesis, leads to a progressive destruction of normal ECM and replacement by a stiffer tumor-derived ECM. The tumor-derived ECM, in turn, has been shown to stimulate proliferation and apoptotic mechanisms, which is believed to lead to the selection of apoptosis-resistant tumor cells with enhanced invasiveness.Citation85–87 Further, MMP-mediated degradation of the ECM facilitates cell migration and also generates numerous bioactive cleaved peptides and releases growth factors and chemokines contained within the ECM,Citation88 which further promotes metastatic progression.
In addition, there is an increase in the prevalence of cross-linking within the tumor stroma.Citation89 The crosslinking of collagen fibers is achieved through the activity of lysyl oxidase (LOX) proteins, and the expression and activity of LOX proteins are elevated in response to increased collagen deposition. Increased crosslinking results in increased stiffnessCitation72 and has been shown to increase the invasiveness of many types of cancer cells.Citation90,91 As a result, elevated LOX expression is significantly correlated with metastasis and decreased survival in cancer patients.Citation92 While the exact mechanisms by which increased ECM stiffness increases cancer cell invasiveness remain to be elucidated, it is thought to involve increased cellular adhesion to the ECM, integrin clustering, and focal adhesion activation.Citation93 Additionally, increased integrin clustering results in enhanced mechanotransduction that ultimately promotes cell migration.Citation94 Interestingly, primary tumor-mediated ECM remodeling has also been observed at future sites of metastasis; these secondary sites of metastasis are referred to as pre-metastatic niches and have been shown to be formed, in part, by primary tumor-secreted LOX proteins.Citation91 LOX-dependent ECM remodeling and matrix stiffening at distant sites act to facilitate tumor cell colonization and growth, thus highlighting the critical role that ECM stiffness plays in encouraging a tumor microenvironment.
In addition to changes in ECM composition, ECM architecture is also fundamentally different within tumors. Rather than the relaxed, non-oriented fibrils found in normal tissue stroma, the collagen fibrils found in breast tissue are often thicker and align perpendicular to the tumor boundary, which is associated with malignant transformation and metastatic progression.Citation72,95 This non-random orientation of ECM fibers is called anisotropy and leads to concentration gradients of fibrils that generate differential tension and migration tracks that can guide cell migration and induce invasivity. The cells themselves assist in the observed alignment of ECM associated with tumor formation, and these activities have been shown to be dependent on Rho activity.Citation82 Additionally, transformed mammary epithelial cells are often found on bundles of linear collagen fibers adjacent to blood vessels,Citation96 further facilitating metastasis. Remodeling of basement membranes is also commonly associated with cancer, and further, with malignancy and metastasis. Disruption of the basement membrane eliminates apicobasal polarity and allows cancer cells to escape the primary tumor. Together, several changes occur to the architecture of ECM found within a tumor microenvironment that alters tissue mechanics and subsequent cell behavior to drive malignant transformation and metastatic progression. Interestingly, tumorigenic cells can become phenotypically normal if the microenvironment is appropriately manipulated,Citation97 exemplifying the importance of tissue stiffness on cell activities.
Conclusion
ECM stiffness plays an important role in dictating several aspects of tissue development, such as orienting cell division, maintaining tissue boundaries, directing cell migration, and driving differentiation. Similarly, ECM stiffness is important for maintaining normal tissue homeostasis, and abnormal ECM stiffness is often a hallmark of several diseases like cancer. As tissue engineering strategies continue to gain popularity as viable options to treat a variety of diseases, an understanding of the role that ECM stiffness plays in dictating cell fate decisions will be critical to the overall success of these approaches.
DISCLOSURE OF POTENTIAL CONFLICTS OF INTEREST
No potential conflicts of interest were disclosed.
REFERENCES
- Burridge K, Chrzanowska-Wodnicka M. Focal adhesions, contractility, and signaling. Annu Rev Cell Dev Biol 1996; 12:463-518; PMID:8970735; http://dx.doi.org/10.1146/annurev.cellbio.12.1.463
- Mege RM, Gavard J, Lambert M. Regulation of cell-cell junctions by the cytoskeleton. Curr Opin Cell Biol 2006; 18:541-8; PMID:16905303; http://dx.doi.org/10.1016/j.ceb.2006.08.004
- Kumar A, Gupta T, Berzsenyi S, Giangrande A. N-cadherin negatively regulates collective drosophila glial migration via actin cytoskeleton remodeling. J Cell Sci 2015; 128:900-12; PMID:25593128
- Podkowa M, Christova T, Zhao X, Jian Y, Attisano L. p21-Activated kinase (PAK) is required for bone morphogenetic protein (BMP)-induced dendritogenesis in cortical neurons. Mol Cellular Neurosci 2013; 57:83-92; PMID:24141051; http://dx.doi.org/10.1016/j.mcn.2013.10.005
- Morris HT, Machesky LM. Actin cytoskeletal control during epithelial to mesenchymal transition: focus on the pancreas and intestinal tract. British J Cancer 2015; 112:613-20; PMID:25611303; http://dx.doi.org/10.1038/bjc.2014.658
- Steward AJ, Kelly DJ. Mechanical regulation of mesenchymal stem cell differentiation. J Anatomy 2014; PMID:25382217; http://dx.doi.org/10.1111/joa.12243
- Sreenivasappa H, Chaki SP, Lim SM, Trzeciakowski JP, Davidson MW, Rivera GM, Trache A. Selective regulation of cytoskeletal tension and cell-matrix adhesion by RhoA and Src. Int Biol 2014; 6:743-54; PMID:24984203; http://dx.doi.org/10.1039/c4ib00019f
- Bae YH, Mui KL, Hsu BY, Liu SL, Cretu A, Razinia Z, Xu T, Puré E, Assoian RK. A FAK-Cas-Rac-lamellipodin signaling module transduces extracellular matrix stiffness into mechanosensitive cell cycling. Sci Signaling 2014; 7:ra57; PMID:24939893; http://dx.doi.org/10.1126/scisignal.2004838
- Larjava H, Koivisto L, Heino J, Hakkinen L. Integrins in periodontal disease. Exp Cell Res 2014; 325:104-10; PMID:24662197; http://dx.doi.org/10.1016/j.yexcr.2014.03.010
- Guan JL. Integrin signaling through FAK in the regulation of mammary stem cells and breast cancer. Iubmb Life 2010; 62:268-76; PMID:20101634; http://dx.doi.org/10.1002/iub.311
- Bolos V, Gasent JM, Lopez-Tarruella S, Grande E. The dual kinase complex FAK-Src as a promising therapeutic target in cancer. Oncotargets Ther 2010; 3:83-97; PMID:20616959; http://dx.doi.org/10.2147/OTT.S6909
- Plotnikov SV, Pasapera AM, Sabass B, Waterman CM. Force fluctuations within focal adhesions mediate ECM-rigidity sensing to guide directed cell migration. Cell 2012; 151:1513-27; PMID:23260139; http://dx.doi.org/10.1016/j.cell.2012.11.034
- Shih YR, Tseng KF, Lai HY, Lin CH, Lee OK. Matrix stiffness regulation of integrin-mediated mechanotransduction during osteogenic differentiation of human mesenchymal stem cells. J Bone Min Res 2011; 26:730-8; PMID:20939067; http://dx.doi.org/10.1002/jbmr.278
- Jaffe AB, Hall A. Rho GTPases: biochemistry and biology. Annu Rev Cell Dev Biol 2005; 21:247-69; PMID:16212495; http://dx.doi.org/10.1146/annurev.cellbio.21.020604.150721
- Burridge K, Wennerberg K. Rho and Rac take center stage. Cell 2004; 116:167-79; PMID:14744429; http://dx.doi.org/10.1016/S0092-8674(04)00003-0
- Riento K, Ridley AJ. Rocks: multifunctional kinases in cell behaviour. Nat Rev Mol Cell Biol 2003; 4:446-56; PMID:12778124; http://dx.doi.org/10.1038/nrm1128
- Goode BL, Eck MJ. Mechanism and function of formins in the control of actin assembly. Annu Rev Biochem 2007; 76:593-627; PMID:17373907; http://dx.doi.org/10.1146/annurev.biochem.75.103004.142647
- Maekawa M, Ishizaki T, Boku S, Watanabe N, Fujita A, Iwamatsu A, Obinata T, Ohashi K, Mizuno K, Narumiya S. Signaling from Rho to the actin cytoskeleton through protein kinases ROCK and LIM-kinase. Science 1999; 285:895-8; PMID:10436159; http://dx.doi.org/10.1126/science.285.5429.895
- Huang X, Yang N, Fiore VF, Barker TH, Sun Y, Morris SW, Ding Q, Thannickal VJ, Zhou Y. Matrix stiffness-induced myofibroblast differentiation is mediated by intrinsic mechanotransduction. Am J Resp Cell Mol Biol 2012; 47:340-8; PMID:22461426; http://dx.doi.org/10.1165/rcmb.2012-0050OC
- Chaudhuri O, Koshy ST, Branco da Cunha C, Shin JW, Verbeke CS, Allison KH, Mooney DJ. Extracellular matrix stiffness and composition jointly regulate the induction of malignant phenotypes in mammary epithelium. Nat Materials 2014; 13:970-8; PMID:24930031; http://dx.doi.org/10.1038/nmat4009
- Mecham RP, Heuser J. Three-dimensional organization of extracellular matrix in elastic cartilage as viewed by quick freeze, deep etch electron microscopy. Connect Tissue Res 1990; 24:83-93; PMID:2354636; http://dx.doi.org/10.3109/03008-209009152425
- Grinnell F. Fibroblast-collagen-matrix contraction: growth-factor signalling and mechanical loading. Trends Cell Biol 2000; 10:362-5; PMID:10932093; http://dx.doi.org/10.1016/S0962-8924(00)01802-X
- Thery M, Racine V, Pepin A, Piel M, Chen Y, Sibarita JB, Bornens M. The extracellular matrix guides the orientation of the cell division axis. Nat Cell Biol 2005; 7:947-53; PMID:16179950; http://dx.doi.org/10.1038/ncb1307
- Zhou J, Kim HY, Davidson LA. Actomyosin stiffens the vertebrate embryo during crucial stages of elongation and neural tube closure. Devel 2009; 136:677-88; PMID:19168681; http://dx.doi.org/10.1242/dev.026211
- Monier B, Pelissier-Monier A, Brand AH, Sanson B. An actomyosin-based barrier inhibits cell mixing at compartmental boundaries in drosophila embryos. Nat Cell Biol 2010; 12:60-5:1-9; PMID:19966783; http://dx.doi.org/10.1038/ncb2005
- Fernandez-Gonzalez R, Simoes Sde M, Roper JC, Eaton S, Zallen JA. Myosin II dynamics are regulated by tension in intercalating cells. Dev Cell 2009; 17:736-43; PMID:19879198; http://dx.doi.org/10.1016/j.devcel.2009.09.003
- Sawyer JM, Harrell JR, Shemer G, Sullivan-Brown J, Roh-Johnson M, Goldstein B. Apical constriction: a cell shape change that can drive morphogenesis. Dev Biol 2010; 341:5-19; PMID:19751720; http://dx.doi.org/10.1016/j.ydbio.2009.09.009
- Wang HB, Dembo M, Hanks SK, Wang Y. Focal adhesion kinase is involved in mechanosensing during fibroblast migration. Proc Natl Acad Sci U S A 2001; 98:11295-300; PMID:11572981; http://dx.doi.org/10.1073/pnas.201201198
- Moore KA, Polte T, Huang S, Shi B, Alsberg E, Sunday ME, Ingber DE. Control of basement membrane remodeling and epithelial branching morphogenesis in embryonic lung by Rho and cytoskeletal tension. Dev Dyn 2005; 232:268-81; PMID:15614768; http://dx.doi.org/10.1002/dvdy.20237
- Silberstein GB, Daniel CW. Glycosaminoglycans in the basal lamina and extracellular matrix of the developing mouse mammary duct. Dev Biol 1982; 90:215-22; PMID:6800862; http://dx.doi.org/10.1016/0012-1606(82)90228-7
- Silberstein GB, Daniel CW. Reversible inhibition of mammary gland growth by transforming growth factor-β. Science 1987; 237:291-3; PMID:3474783; http://dx.doi.org/10.1126/science.3474783
- Huang S, Ingber DE. The structural and mechanical complexity of cell-growth control. Nat Cell Biol 1999; 1:E131-8; PMID:10559956; http://dx.doi.org/10.1038/13043
- Nelson CM, Vanduijn MM, Inman JL, Fletcher DA, Bissell MJ. Tissue geometry determines sites of mammary branching morphogenesis in organotypic cultures. Science 2006; 314:298-300; PMID:17038622; http://dx.doi.org/10.1126/science.1131000
- Alcaraz J, Xu R, Mori H, Nelson CM, Mroue R, Spencer VA, Brownfield D, Radisky DC, Bustamante C, Bissell MJ. Laminin and biomimetic extracellular elasticity enhance functional differentiation in mammary epithelia. EMBO J 2008; 27:2829-38; PMID:18843297; http://dx.doi.org/10.1038/emboj.2008.206
- Wicha MS, Lowrie G, Kohn E, Bagavandoss P, Mahn T. Extracellular matrix promotes mammary epithelial growth and differentiation in vitro. Proc Natl Acad Sci U S A 1982; 79:3213-7; PMID:6954472; http://dx.doi.org/10.1073/pnas.79.10.3213
- Ingber DE, Madri JA, Folkman J. A possible mechanism for inhibition of angiogenesis by angiostatic steroids: induction of capillary basement membrane dissolution. Endocrinology 1986; 119:1768-75; PMID:2428602; http://dx.doi.org/10.1210/endo-119-4-1768
- Lahmers S, Wu Y, Call DR, Labeit S, Granzier H. Developmental control of titin isoform expression and passive stiffness in fetal and neonatal myocardium. Circ Res 2004; 94:505-13; PMID:14707027; http://dx.doi.org/10.1161/01.RES.0000115522.52554.86
- Puchner EM, Alexandrovich A, Kho AL, Hensen U, Schafer LV, Brandmeier B, Gräter F, Grubmüller H, Gaub HE, Gautel M. Mechanoenzymatics of titin kinase. Proc Natl Acad Sci U S A 2008; 105:13385-90; PMID:18765796; http://dx.doi.org/10.1073/pnas.0805034105
- Kahn J, Shwartz Y, Blitz E, Krief S, Sharir A, Breitel DA, Rattenbach R, Relaix F, Maire P, Rountree RB. Muscle contraction is necessary to maintain joint progenitor cell fate. Dev Cell 2009; 16:734-43; PMID:19460349; http://dx.doi.org/10.1016/j.devcel.2009.04.013
- Anava S, Greenbaum A, Ben Jacob E, Hanein Y, Ayali A. The regulative role of neurite mechanical tension in network development. Biophys J 2009; 96:1661-70; PMID:19217881; http://dx.doi.org/10.1016/j.bpj.2008.10.058
- Wilson NR, Ty MT, Ingber DE, Sur M, Liu G. Synaptic reorganization in scaled networks of controlled size. J Neurosci 2007; 27:13581-9; PMID:18077670; http://dx.doi.org/10.1523/JNEUROSCI.3863-07.2007
- Serluca FC, Drummond IA, Fishman MC. Endothelial signaling in kidney morphogenesis: a role for hemodynamic forces. Current biology : CB 2002; 12:492-7; PMID:11909536; http://dx.doi.org/10.1016/S0960-9822(02)00694-2
- Vasilyev A, Liu Y, Mudumana S, Mangos S, Lam PY, Majumdar A, Zhao J, Poon KL, Kondrychyn I, Korzh V., et al. Collective cell migration drives morphogenesis of the kidney nephron. PLoS Biol 2009; 7:e9; PMID:19127979; http://dx.doi.org/10.1371/journal.pbio.1000009
- Adamo L, Naveiras O, Wenzel PL, McKinney-Freeman S, Mack PJ, Gracia-Sancho J, Suchy-Dicey A, Yoshimoto M, Lensch MW, Yoder MC., et al. Biomechanical forces promote embryonic haematopoiesis. Nature 2009; 459:1131-5; PMID:19440194; http://dx.doi.org/10.1038/nature08073
- North TE, Goessling W, Peeters M, Li P, Ceol C, Lord AM, Weber GJ, Harris J, Cutting CC, Huang P., et al. Hematopoietic stem cell development is dependent on blood flow. Cell 2009; 137:736-48; PMID:19450519; http://dx.doi.org/10.1016/j.cell.2009.04.023
- Forouhar AS, Liebling M, Hickerson A, Nasiraei-Moghaddam A, Tsai HJ, Hove JR, Fraser SE, Dickinson ME, Gharib M. The embryonic vertebrate heart tube is a dynamic suction pump. Science 2006; 312:751-3; PMID:16675702; http://dx.doi.org/10.1126/science.1123775
- Wei L, Imanaka-Yoshida K, Wang L, Zhan S, Schneider MD, DeMayo FJ, Schwartz RJ. Inhibition of Rho family GTPases by Rho GDP dissociation inhibitor disrupts cardiac morphogenesis and inhibits cardiomyocyte proliferation. Dev 2002; 129:1705-14; PMID:11923206
- le Noble F, Moyon D, Pardanaud L, Yuan L, Djonov V, Matthijsen R, Bréant C, Fleury V, Eichmann A. Flow regulates arterial-venous differentiation in the chick embryo yolk sac. Dev 2004; 131:361-75; PMID:14681188; http://dx.doi.org/10.1242/dev.00929
- Cohen JC, Larson JE. Cystic fibrosis transmembrane conductance regulator (CFTR) dependent cytoskeletal tension during lung organogenesis. Dev Dyn 2006; 235:2736-48; PMID:16906518; http://dx.doi.org/10.1002/dvdy.20912
- Khatiwala CB, Kim PD, Peyton SR, Putnam AJ. ECM compliance regulates osteogenesis by influencing MAPK signaling downstream of RhoA and ROCK. J Bone Mineral Res 2009; 24:886-98; PMID:19113908; http://dx.doi.org/10.1359/jbmr.081240
- Engler AJ, Sen S, Sweeney HL, Discher DE. Matrix elasticity directs stem cell lineage specification. Cell 2006; 126:677-89; PMID:16923388; http://dx.doi.org/10.1016/j.cell.2006.06.044
- Rodan GA. Bone mass homeostasis and bisphosphonate action. Bone 1997; 20:1-4; PMID:8988341; http://dx.doi.org/10.1016/S8756-3282(96)00318-3
- Han SK, Madden R, Abusara Z, Herzog W. In situ chondrocyte viscoelasticity. J Biomech 2012; 45:2450-6; PMID:22884037; http://dx.doi.org/10.1016/j.jbiomech.2012.06.028
- Lu CH, Zhang J, Zhang DH, Uzan G, Li MQ. EPCs in vascular repair: How can we clear the hurdles between bench and bedside? Front Biosci-Landmrk 2014; 19:34-48; PMID:24389171; http://dx.doi.org/10.2741/4194
- Chen QS, Jin M, Yang F, Zhu JH, Xiao QZ, Zhang L. Matrix Metalloproteinases: Inflammatory Regulators of Cell Behaviors in Vascular Formation and Remodeling. Mediat Inflamm 2013; 2013:928315; PMID:23840100; http://dx.doi.org/10.1155/2013/928315
- Hanjaya-Putra D, Yee J, Ceci D, Truitt R, Yee D, Gerecht S. Vascular endothelial growth factor and substrate mechanics regulate in vitro tubulogenesis of endothelial progenitor cells. J Cell Mol Med 2010; 14:2436-47; PMID:19968735; http://dx.doi.org/10.1111/j.1582-4934.2009.00981.x
- Georges PC, Miller WJ, Meaney DF, Sawyer ES, Janmey PA. Matrices with compliance comparable to that of brain tissue select neuronal over glial growth in mixed cortical cultures. Biophys J 2006; 90:3012-8; PMID:16461391; http://dx.doi.org/10.1529/biophysj.105.073114
- Maynard FM, Jr., Bracken MB, Creasey G, Ditunno JF, Jr., Donovan WH, Ducker TB, Garber SL, Marino RJ, Stover SL, Tator CH., et al. International Standards for Neurological and Functional Classification of Spinal Cord Injury. American Spinal Injury Association. Spinal Cord 1997; 35:266-74; PMID:9160449; http://dx.doi.org/10.1038/sj.sc.3100432
- Horner PJ, Gage FH. Regenerating the damaged central nervous system. Nature 2000; 407:963-70; PMID:11069169; http://dx.doi.org/10.1038/35039559
- Camand E, Morel MP, Faissner A, Sotelo C, Dusart I. Long-term changes in the molecular composition of the glial scar and progressive increase of serotoninergic fibre sprouting after hemisection of the mouse spinal cord. Eur J Neurosci 2004; 20:1161-76; PMID:15341588; http://dx.doi.org/10.1111/j.1460-9568.2004.03558.x
- Raivich G, Bohatschek M, Kloss CU, Werner A, Jones LL, Kreutzberg GW. Neuroglial activation repertoire in the injured brain: graded response, molecular mechanisms and cues to physiological function. Brain Res Brain Res Rev 1999; 30:77-105; PMID:10407127; http://dx.doi.org/10.1016/S0165-0173(99)00007-7
- Choi WJ, Russell CM, Tsai CM, Arzanpour S, Robinovitch SN. Age-related changes in dynamic compressive properties of trochanteric soft tissues over the hip. J Biomech 2014; PMID:25596629; http://dx.doi.org/10.1016/j.jbiomech.2014.12.026
- Wood LK, Kayupov E, Gumucio JP, Mendias CL, Claflin DR, Brooks SV. Intrinsic stiffness of extracellular matrix increases with age in skeletal muscles of mice. J Appl Physiol 2014; 117:363-9; PMID:24994884; http://dx.doi.org/10.1152/japplphysiol.00256.2014
- Huynh J, Nishimura N, Rana K, Peloquin JM, Califano JP, Montague CR, King MR, Schaffer CB, Reinhart-King CA. Age-related intimal stiffening enhances endothelial permeability and leukocyte transmigration. Sci Transl Med 2011; 3:112ra22; PMID:22158860; http://dx.doi.org/10.1126/scitranslmed.3002761
- Issa R, Zhou X, Constandinou CM, Fallowfield J, Millward-Sadler H, Gaca MD, Gaca MD, Sands E, Suliman I, Trim N., et al. Spontaneous recovery from micronodular cirrhosis: evidence for incomplete resolution associated with matrix cross-linking. Gastroenterology 2004; 126:1795-808; PMID:15188175; http://dx.doi.org/10.1053/j.gastro.2004.03.009
- Birk DE, Bruckner P. Collagen suprastructures. Collagen 2005; 247:185-205
- Sherratt MJ, Baldock C, Haston JL, Holmes DF, Jones CJP, Shuttleworth CA, Wess TJ, Kielty CM. Fibrillin microfibrils are stiff reinforcing fibres in compliant tissues. J Mol Biol 2003; 332:183-93; PMID:12946356; http://dx.doi.org/10.1016/S0022-2836(03)00829-5
- Akhtar R, Sherratt MJ, Watson RE, Kundu T, Derby B. Mapping the micromechanical properties of cryo-sectioned aortic tissue with scanning acoustic microscopy. Mater Res Soc Symp Proc 2009; 1132E:ukpmcpa27262; PMID:19603080
- Suki B, Bates JH. Extracellular matrix mechanics in lung parenchymal diseases. Respir Physiol Neurobiol 2008; 163:33-43; PMID:18485836; http://dx.doi.org/10.1016/j.resp.2008.03.015
- Kass L, Erler JT, Dembo M, Weaver VM. Mammary epithelial cell: influence of extracellular matrix composition and organization during development and tumorigenesis. Int J Biochem Cell Biol 2007; 39:1987-94; PMID:17719831; http://dx.doi.org/10.1016/j.biocel.2007.06.025
- Butcher DT, Alliston T, Weaver VM. A tense situation: forcing tumour progression. Nat Rev Cancer 2009; 9:108-22; PMID:19165226; http://dx.doi.org/10.1038/nrc2544
- Levental KR, Yu H, Kass L, Lakins JN, Egeblad M, Erler JT, Fong SF, Csiszar K, Giaccia A, Weninger W., et al. Matrix crosslinking forces tumor progression by enhancing integrin signaling. Cell 2009; 139:891-906; PMID:19931152; http://dx.doi.org/10.1016/j.cell.2009.10.027
- Provenzano PP, Inman DR, Eliceiri KW, Knittel JG, Yan L, Rueden CT, White JG, Keely PJ., et al. Collagen density promotes mammary tumor initiation and progression. BMC Med 2008; 6:11; PMID:18442412; http://dx.doi.org/10.1186/1741-7015-6-11
- Kadler KE, Hill A, Canty-Laird EG. Collagen fibrillogenesis: fibronectin, integrins, and minor collagens as organizers and nucleators. Curr Opin Cell Biol 2008; 20:495-501; PMID:18640274; http://dx.doi.org/10.1016/j.ceb.2008.06.008
- Guarnieri D, Battista S, Borzacchiello A, Mayol L, De Rosa E, Keene DR, Muscariello L, Barbarisi A, Netti PA. Effects of fibronectin and laminin on structural, mechanical and transport properties of 3D collageneous network. J Mater Sci Mater Med 2007; 18:245-53; PMID:17323155; http://dx.doi.org/10.1007/s10856-006-0686-5
- Egeblad M, Rasch MG, Weaver VM. Dynamic interplay between the collagen scaffold and tumor evolution. Curr Opin Cell Biol 2010; 22:697-706; PMID:20822891; http://dx.doi.org/10.1016/j.ceb.2010.08.015
- Wenstrup RJ, Florer JB, Brunskill EW, Bell SM, Chervoneva I, Birk DE. Type V collagen controls the initiation of collagen fibril assembly. J Biol Chem 2004; 279:53331-7; PMID:15383546; http://dx.doi.org/10.1074/jbc.M409622200
- Nasser NJ. Heparanase involvement in physiology and disease. Cell Mol Life Sci 2008; 65:1706-15; PMID:18425416; http://dx.doi.org/10.1007/s00018-008-7584-6
- Orian-Rousseau V. CD44, a therapeutic target for metastasising tumours. Eur J Cancer 2010; 46:1271-7; PMID:20303742; http://dx.doi.org/10.1016/j.ejca.2010.02.024
- Wozniak MA, Desai R, Solski PA, Der CJ, Keely PJ. ROCK-generated contractility regulates breast epithelial cell differentiation in response to the physical properties of a three-dimensional collagen matrix. J Cell Biol 2003; 163:583-95; PMID:14610060; http://dx.doi.org/10.1083/jcb.200305010
- Klein EA, Yin L, Kothapalli D, Castagnino P, Byfield FJ, Xu T, Levental I, Hawthorne E, Janmey PA, Assoian RK. Cell-cycle control by physiological matrix elasticity and in vivo tissue stiffening. Curr Biol 2009; 19:1511-8; PMID:19765988; http://dx.doi.org/10.1016/j.cub.2009.07.069
- Provenzano PP, Inman DR, Eliceiri KW, Keely PJ. Matrix density-induced mechanoregulation of breast cell phenotype, signaling and gene expression through a FAK-ERK linkage. Oncogene 2009; 28:4326-43; PMID:19826415; http://dx.doi.org/10.1038/onc.2009.299
- Emerman JT, Pitelka DR. Maintenance and induction of morphological differentiation in dissociated mammary epithelium on floating collagen membranes. In Vitro 1977; 13:316-28; PMID:559643; http://dx.doi.org/10.1007/BF02616178
- Streuli CH, Schmidhauser C, Bailey N, Yurchenco P, Skubitz AP, Roskelley C, Bissell MJ. Laminin mediates tissue-specific gene expression in mammary epithelia. J Cell Biol 1995; 129:591-603; PMID:7730398; http://dx.doi.org/10.1083/jcb.129.3.591
- Sethi T, Rintoul RC, Moore SM, MacKinnon AC, Salter D, Choo C, Chilvers ER, Dransfield I, Donnelly SC, Strieter R., et al. Extracellular matrix proteins protect small cell lung cancer cells against apoptosis: a mechanism for small cell lung cancer growth and drug resistance in vivo. Nat Med 1999; 5:662-8; PMID:10371505; http://dx.doi.org/10.1038/9511
- Coussens LM, Werb Z. Inflammatory cells and cancer: think different! J Exp Med 2001; 193:F23-6; PMID:11257144
- Mitsiades N, Yu WH, Poulaki V, Tsokos M, Stamenkovic I. Matrix metalloproteinase-7-mediated cleavage of Fas ligand protects tumor cells from chemotherapeutic drug cytotoxicity. Cancer Res 2001; 61:577-81; PMID:11212252
- Egeblad M, Werb Z. New functions for the matrix metalloproteinases in cancer progression. Nat Rev Cancer 2002; 2:161-74; PMID:11990853; http://dx.doi.org/10.1038/nrc745
- Ng MR, Brugge JS. A stiff blow from the stroma: collagen crosslinking drives tumor progression. Cancer Cell 2009; 16:455-7; PMID:19962663; http://dx.doi.org/10.1016/j.ccr.2009.11.013
- Kirschmann DA, Seftor EA, Fong SF, Nieva DR, Sullivan CM, Edwards EM, Sommer P, Csiszar K, Hendrix MJ. A molecular role for lysyl oxidase in breast cancer invasion. Cancer Res 2002; 62:4478-83; PMID:12154058
- Erler JT, Weaver VM. Three-dimensional context regulation of metastasis. Clin Exp Metastasis 2009; 26:35-49; PMID:18814043; http://dx.doi.org/10.1007/s10585-008-9209-8
- Erler JT, Bennewith KL, Nicolau M, Dornhofer N, Kong C, Le QT, Chi JT, Jeffrey SS, Giaccia AJ. Lysyl oxidase is essential for hypoxia-induced metastasis. Nature 2006; 440:1222-6; PMID:16642001; http://dx.doi.org/10.1038/nature04695
- Payne SL, Fogelgren B, Hess AR, Seftor EA, Wiley EL, Fong SF, Csiszar K, Hendrix MJ, Kirschmann DA. Lysyl oxidase regulates breast cancer cell migration and adhesion through a hydrogen peroxide-mediated mechanism. Cancer Res 2005; 65:11429-36; PMID:16357151; http://dx.doi.org/10.1158/0008-5472.CAN-05-1274
- Paszek MJ, Zahir N, Johnson KR, Lakins JN, Rozenberg GI, Gefen A, Reinhart-King CA, Margulies SS, Dembo M, Boettiger D., et al. Tensional homeostasis and the malignant phenotype. Cancer Cell 2005; 8:241-54; PMID:16169468; http://dx.doi.org/10.1016/j.ccr.2005.08.010
- Provenzano PP, Eliceiri KW, Campbell JM, Inman DR, White JG, Keely PJ. Collagen reorganization at the tumor-stromal interface facilitates local invasion. BMC Med 2006; 4:38; PMID:17190588; http://dx.doi.org/10.1186/1741-7015-4-38
- Ingman WV, Wyckoff J, Gouon-Evans V, Condeelis J, Pollard JW. Macrophages promote collagen fibrillogenesis around terminal end buds of the developing mammary gland. Dev Dyn 2006; 235:3222-9; PMID:17029292; http://dx.doi.org/10.1002/dvdy.20972
- Bissell MJ, Radisky D. Putting tumours in context. Nat Rev Cancer 2001; 1:46-54; PMID:11900251; http://dx.doi.org/10.1038/35094059
- Cox TR, Erler JT. Remodeling and homeostasis of the extracellular matrix: implications for fibrotic diseases and cancer. Dis Models Mechanisms 2011; 4:165-78; PMID:21324931; http://dx.doi.org/10.1242/dmm.004077