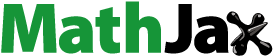
ABSTRACT
Retinoic acid has recently been shown to control the phenotype and extracellular matrix composition of corneal stromal cells cultured in vitro as monolayers. This study set out to investigate the effects of retinoic acid on human corneal keratocytes within a 3D environment. Human corneal keratocytes were encapsulated in collagen gels, which were subsequently compressed under load, and cultured in serum-free media supplemented with 10 µM retinoic acid or DMSO vehicle for 30 days. Cell proliferation was quantified on selected days, while the expression of several important keratocytes markers was evaluated at day 30 using RT-PCR and immunoblotting. The weight and size of the collagen constructs were measured before and after hydration and contraction analyses. Retinoic acid enhanced keratocyte proliferation until day 30, whereas cells in control culture conditions showed reduced numbers after day 21. Both gene and protein expressions of keratocyte-characteristic proteoglycans (keratocan, lumican and decorin), corneal crystallins and collagen type I and V were significantly increased following retinoic acid supplementation. Retinoic acid also significantly reduced the expression of matrix metalloproteases 1, 3 and 9 while not increasing α-smooth muscle actin and fibronectin expression. Furthermore, these effects were also correlated with the ability of retinoic acid to significantly inhibit the contractility of keratocytes while allowing the build-up of corneal stromal extracellular matrix within the 3D constructs. Thus, retinoic acid supplementation represents a promising strategy to improve the phenotype of 3D-cultured keratocytes, and their usefulness as a model of corneal stroma for corneal biology and regenerative medicine applications.
INTRODUCTION
The cornea is a transparent, avascular structure that provides optical transparency for light transmission and light refraction for normal vision as well as protection against external environment.Citation1,2 The World Health Organization has estimated over 10 million people from around the world suffer from corneal blindness secondary to trauma, infection or hereditary diseases.Citation3 Although transplantation is the prevailing option to correct severe corneal impairment, the sustainability of this treatment of choice remains a great challenge. This is mainly due to limited supply of corneas from healthy corneal donors, and is made worse by the growth in refractive surgeries, which degrade the cornea for subsequent use in transplantation.Citation4 These issues have fueled interest in the development of tissue-engineered corneas as a long-term strategy to address the aforementioned problems.Citation5,6 In this context, natural biological materials such as collagen, has been used to provide scaffolding support for corneal tissue engineering with promising results.Citation7-9
Collagens are one of the most abundant structural proteins within mammalian connective tissue and represent almost one-third of total body proteins.Citation10 Collagen provides structural support and mechanical stability while maintaining flexibility in various tissues, such as skin,Citation11 tendon,Citation12 bone.Citation13 and cornea.Citation14 Collagen type-I is one of the main structural elements of the extracellular matrix of the corneal stroma. As such, its use as a corneal tissue engineering scaffold has been extensively studied.Citation15-18 The use of collagen in tissue engineering has been attributed to its biocompatibility, biodegradability and low-immunogenicity.Citation19 However, conventional collagen gels are highly hydrated and therefore, difficult to be manipulated.Citation20 An additional step of ‘plastic compression’ following collagen gel formation allows the controlled engineering of collagen gels by rapid dehydration, and subsequently causing permanent deformation and a marked increase in collagen fibril density.Citation21,22 Compressed collagen gels are mechanically robust structures comprised of compacted collagen fibrils with a smooth surface topography.Citation23 It has been suggested that compressed collagen gels are a suitable mimic for the structure of biological materials,Citation21 and several studies have used corneal keratocytes in the collagen scaffold to produce a 3D tissue culture to mimic the presence of keratocytes in normal corneal stroma.Citation24-28
Keratocytes are mesenchymal-derived, flattened cells which sparsely populate the corneal stroma.Citation29,30 Their primary function is to synthesize and maintain the extracellular matrix (ECM) component of the stroma that comprises the structural backbone of the cornea.Citation31 Keratocytes are quiescent in nature and exhibit slow turn over.Citation32 The ex vivo expansion of keratocytes has been performed in order to investigate its biology in vitro, but maintaining them in their quiescent phenotype remains a considerable challenge. The use of serum-free media was shown to maintain the quiescence phenotype, but not support proliferation, of corneal stromal cells,Citation33-35 thus making cultivation of large quantities of cells by subculturing a difficult task. On the other hand, the addition of serum to culture medium helps to accelerate cell proliferation, but at the same time results in the differentiation of keratocytes to fibroblasts with altered physiological and biochemical properties, namely its phenotype, keratan sulfate production and the expressions of certain genes such as α-smooth muscle actin, aldehyde dehydrogenase (ALDH) and fibronectin.Citation29,34,36 Previous studies have tested several media formulations that allow corneal stromal cells to proliferate and survive for extended period in culture while maintaining the expression of keratocyte-specific markers. In particular, fibroblast growth factor 1 (FGF-1),Citation37 FGF-2,Citation38 FGF-2 + heparin,Citation37 and transforming growth factor β3 (TGF-β3)Citation39 have been used to reverse the serum-induced (myo)fibroblast transformation. Therefore, the challenge now is to find a new supplement for serum-free media that allows cell growth and proliferation without encouraging fibroblastic differentiation.
One of the possible supplements for serum-free growth of keratocytes is retinoic acid (RA), a molecule that plays a crucial role in vertebrate development, cellular differentiation and homeostasis,Citation40-42 and is essential to ocular health.Citation43-45
The human body produces RA, an active form of vitamin A, through two sequential oxidation steps: from retinol (absorbed by intestinal mucosa cells following dietary ingestion) to retinaldehyde, and then to RA.Citation46 Its use has been implicated in cutaneous wound healing,Citation47-49 and considerable interest has been steered towards its application in ophthalmology. As such, much of the current lines of research focuses on its potential use in improving corneal wound healing through faster epithelialization,Citation50-52 however, little is known about the effect RA has on corneal keratocytes in their native 3D environment.
In this study, we sought to investigate the effects of RA supplementation in serum-free medium on corneal keratocytes in a 3D environment for tissue engineering purposes. We considered its effect on cell proliferation, collagen production, hydration of tissue constructs and the expression of keratocytes markers at both transcriptional and protein levels in an extended period of culture.
RESULTS
Effects of RA on keratocytes proliferation
The proliferation assays showed a steady increase in the number of cells from day 3 to day 30 in the RA-supplemented group and from day 3 to day 21 in the control group (). The cell numbers of keratocytes in collagen gels cultured using medium with or without supplementation of RA showed comparable values up to 21 d in culture. However, keratocytes cultured in medium supplemented with RA continued to proliferate until day 30, whereas keratocytes cultured in control medium showed a significant decline in cell number. Hence, day 30 was taken as the day for further assessment on the expression of keratocytes markers.
FIGURE 1. Effects of RA supplementation on the proliferation of keratocytes embedded in compressed collagen gels. Quantification was performed using AlamarBlue® assay, and cell numbers were normalized as a percentage of cells initially seeded. Data (mean ± SD) were obtained from 3 independent experiments (n = 3) and compared using t-test (*corresponds to P < 0.05).
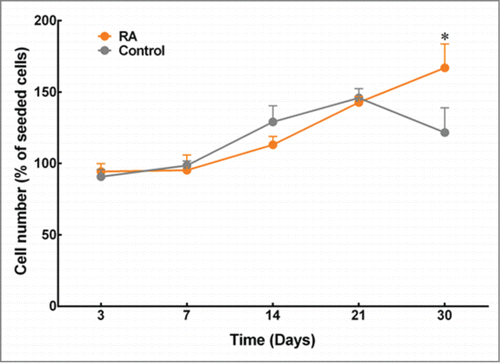
Effects of RA on engineered tissue contraction assay
We used digital images of the engineered stromal constructs to calculate the percentage change in surface area relative to their initial surface area following 30 d of culture (). The engineered stromal constructs cultured in RA-supplemented medium successfully maintained more than 95% of their surface area at the end of the experiment (). On the other hand, the surface area of the constructs cultured in serum-free medium with DMSO (control) were reduced by more than 20% of their original surface area, and the constructs cultured with serum (FBS) contracted more than 60% of their initial surface area. The differences in gel size within the control and FBS groups were significant for the duration of the experiment (p < 0 .01 and < 0 .001, respectively).
FIGURE 2. Contraction assay. (A) Contraction assay of 3D collagen constructs embedded with corneal keratocytes and cultured in serum-free medium (with RA supplementation or DMSO control) or standard medium (with serum; FBS). The outer dotted line represents the size of the gel at the beginning of the experiment. Scale bar = 10 mm. (B) The gel size (in percentage) was determined by calculating the difference in constructs' surface area between the initial day of experiment (white bar) and day 30 (colored bar). Data (mean ± SD) were obtained from 3 independent experiments (n = 3) and compared using t-test (** and *** correspond to P < 0.01 and <0.001, respectively).
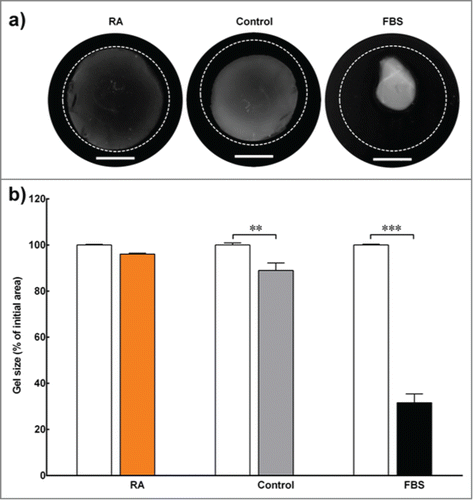
Effects of RA on engineered tissue hydration
Interestingly, there was a significant increase in the wet weight of collagen gels containing keratocytes supplemented with RA as compared to the control after 30 d (). Not only that, the results also showed that collagen gels in the control groups reduced their initial weight following the 30-day culture period. The dry weight of freeze-dried gels from the RA-supplemented group was also found to be significantly higher by 18% than the controls (). At the same time, the hydration percentage within RA-supplemented collagen gels showed to be higher compared to the collagen gels from the control group (84 ± 1 and 80 ± 2%, respectively; ).
FIGURE 3. Effects of RA supplementation on the hydration of cell-encapsulating compressed collagen gels. The weight of all gels was measured before and after the 30 d culture period to find the differences in the wet weight. The dry weight was quantified following freeze-drying of the gels. The percentage of hydration denotes the loss of bound water from the collagen gels with or without supplementation of RA. Data (mean ± SD) were obtained from 3 independent experiments (n = 3) and compared using t-test (*** correspond to P <0.001).
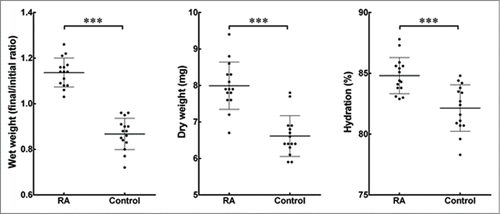
Effects of RA on the expression of keratocytes markers
Several important keratocytes markers were analyzed at the gene transcript level and compared with the control. Results showed that RA treatment significantly increased transcription of genes coding for key corneal proteoglycans (keratocan, lumican and decorin) by 1436 ± 12, 6410 ± 37, and 1510 ± 5 % of the control, respectively (). In addition, transcription levels of genes coding for corneal crystallins (ALDH1 and ALDH3), CHST6 and Collagen type V were also studied and shown to increase by 1181 ± 7, 3826 ± 38, 596 ± 1 and 941 ± 8 % of the control, respectively. On the other hand, genes coding for two keratocytes matrix metalloproteases, MMP1 and MMP9 were significantly down regulated to 6.6 ± 0.04 and 5.2 ± 0.04 %, respectively, when compared to the control (). No difference was observed for transcripts of ACTA2, the gene coding for αSMA.
FIGURE 4. Expression of keratocyte markers at the transcriptional level. Total mRNA from cells embedded in compressed collagen gels cultured for 30 d in control (gray) and +RA (orange) was extracted and analyzed by RT-PCR. Gene expression was normalized relative to that of control group. Data (mean ± SD) were obtained from 3 independent experiments (n = 3) and compared using t-test (*, ** and *** correspond to P < 0.05, <0.01 and <0.001, respectively).
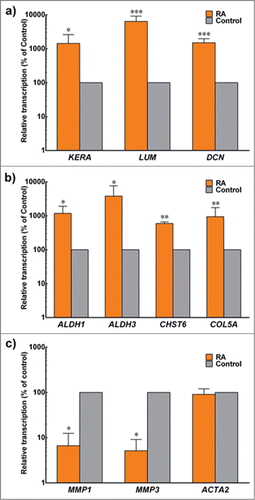
Furthermore, these data were supported by protein expression levels analyzed by immunoblotting and quantified by densitometry. RA-supplemented keratocytes showed significant percentage-increased expression for keratocan (197.4 ± 0.5), lumican (251.7 ± 0.5), decorin (321.2 ± 0.5), Collagen type I (198.5 ± 0.3), Collagen type V (210.4 ± 0.8), ALDH1 (575.9 ± 2.9), ALDH3 (301.2 ± 0.6) and CHST6 (689.0 ± 3.5) relative to the control (). Again similar to gene transcript levels, the expression of MMP-1 and MMP-3 in RA supplemented group was significantly reduced to 7.4 ± 0.08 and 10.1 ± 0.07 % of the control levels, respectively (). In addition, αSMA and fibronectin levels were not affected by RA supplementation, and remained residual compared with the heightened levels normally seen in FBS-activated fibroblasts.
FIGURE 5. Expression of keratocyte markers at the protein level. (A) Lysates from corneal stroma (CS), corneal fibroblasts cultured in FBS (FBS), or 3D stromal constructs supplemented with RA (RA) or DMSO (Ctl) were extracted and analyzed by reducing SDS-PAGE followed by immunoblotting. (B-E) Quantification of protein expression from control (gray) and RA (orange) was performed by immunoblot densitometry for all markers. Protein expression was normalized relatively to that of control group. Data (mean ± SD) were obtained from 3 independent experiments (n = 3) and compared using t-test (* correspond to P < 0.05 respectively).
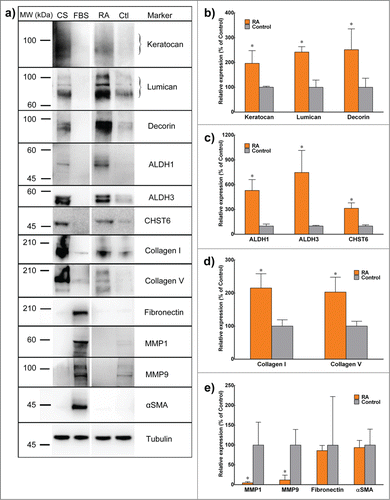
The localizations of these proteins, shown by immunofluorescence analysis, were not substantially modified by RA supplementation despite their stronger detections. However, localization of Collagen type V was shown to be similar throughout the depth of collagen gels in both groups despite its stronger detection in the RA-supplemented group at both transcriptional and protein levels ()
.DISCUSSION
Our previous work has shown that using an optimal concentration of RA encouraged the keratocytes to grow and proliferate further without the need for serum in the medium.Citation53 In this study, we extended the experiment into a 3D construct to investigate the use of RA supplementation in the generation of potential biologically relevant tissue-engineered corneal stroma. The use of RA for in vitro keratocytes studies holds great promise, since we have shown that its supplementation successfully induced proliferative potential of keratocytes when used in serum-free medium over an extended period in culture. This is important to tissue engineering as engineered constructs, such as the cornea, typically require many days in culture.
In the present study, we demonstrated that supplementation of RA in serum-free medium to culture corneal fibroblasts in a 3D collagen construct helped prevent cells from becoming fibroblastic and remained more keratocyte-like as evidenced by an increasing production of keratocyte-markers and very low expressions of fibroblastic-markers. Chemical cues are known as important elements in the control and maintenance of the keratocyte phenotype.Citation35 A considerable challenge in stromal cell culture is to encourage the keratocytes to proliferate while at the same time maintaining the keratocyte phenotype in order to continue producing the ECM proteins essential for optical transparency. Previous attempts have been made towards obtaining a proliferating culture of ‘pure’ keratocytes, including supplementation with ascorbic acid,Citation54 insulin,Citation55 growth factors,Citation56 cytokines,Citation57 and using low glucose media.Citation58 Within the 3D environment, we found that RA significantly modulated the expression of many keratocyte-characteristic ECM components (keratocan, lumican, decorin), the corneal crystallins (ALDH1, ALDH3) and carbohydrate sulfotransferase 6 (CHST6), as well as increased expression of both Collagen type I and V.
Keratocytes secrete Collagen type I and V, which are the predominant fibrillar collagens in the corneal stroma. In addition, the uniformity of these fibrillar collagens (diameter and interfibrillar spacing) is important for the maintenance of corneal function. Keratocan, lumican and decorin belong to the family of small leucine-rich proteoglycans (SLRPs) which serve as regulators of tissue hydration and collagen fibrillogenesis.Citation59,60 These SLRPs bind to fibrillar collagens and affect the collagen matrix assembly needed for corneal transparency.Citation61 Furthermore, significant increases in ALDH1 and ALDH3 expression within the RA-supplemented group were also important findings. Prominent ALDH isoenzymes in cornea, such as ALDH1 and ALDH3, exert protective effects from the harmful effects of UV-induced lipid peroxidation,Citation62-64 and maintain corneal transparency.Citation65 ALDH is produced in greater amounts by quiescent keratocytes compared to their activated phenotype, as shown both in vitro (following exposure to serum)Citation66,67 and in vivo.Citation68 As such, a decrease in corneal crystallins is posited to increase light scattering by the corneal fibroblasts, and decrease corneal transparency.Citation69 Interestingly, RA significantly modulated the expression of CHST6, an enzyme that catalyzes the transfer of sulfate group to keratan sulfate proteoglycans (KSPGs) to help maintain corneal transparency. CHST6 is mainly expressed by the keratocytes both in vivo and in vitro.Citation70 Defects in this gene are associated with macular corneal dystrophy (MCD), a rare autosomal recessive inherited disorder characterized by abnormal deposits of unsulfated KSPGs that causes progressive stromal haziness leading to visual impairment.Citation71,72 An increase synthesis of CHST6 following RA supplementation suggests that RA is safe for corneal keratocytes. At the same time, this result implies the considerable potential for RA to be used as a possible therapy for MCD, as the aim in curing this rare genetic disorder must involve and target corneal keratocytes.Citation70
The expression of MMP1 (i.e. interstitial collagenase) and MMP3 (i.e. stromelysin-1) was greatly reduced following RA supplementation, in line with previous findingsCitation73 in diabetic human skin, which showed enhanced collagen synthesis and reduction in MMP expression in the presence of RA. Under normal circumstances, MMP-1 and MMP-3 are not synthesized by quiescent keratocytes. However, the synthesis is upregulated when the keratocyte's phenotype changes into that of repair fibroblasts.Citation74,75 We also evaluated the expression of α-SMA and fibronectin and found that they appear to be unaffected by the presence of RA in the cultures i.e. low, as one would expect from serum-free conditions.
The increase in ECM production following RA-supplementation may also contribute to the measured increase in both wet and dry weight of the 3D collagen-keratocyte constructs. The wet weight of gels significantly increased after the 30-days period in culture in RA conditions compared to their initial wet weight, but decreased in control gels, indicating that RA induced the resident keratocytes to 1) produce larger amounts of newly-synthesized material, 2) enhanced the gels' ability to accumulate water, and/or 3) inhibited degradation of the initial collagen gel scaffold compared to control conditions. These conclusions are supported by the significant increase in dry weight of RA-treated gels (), as well as by the RA-induced enhancement of keratocyte-characteristic ECM components and the abrogation of MMP expression observed at the gene and protein levels ( respectively). Moreover, the higher content of keratocyte-characteristic proteoglycans (keratocan, lumican and decorin) in the RA-treated group might account for the higher capacity of these gels to retain water (). As mentioned previously, the SLRPs serve as regulators for tissue hydration. Since there were more SLRPs in collagen constructs supplemented with RA, it was not surprising that the water content within these gels was higher compared to the control. Therefore, both wet and dry weight values were important to understand the dynamic changes in the properties in this gel-keratocyte system, and were necessary to determine the constructs' hydration, an important property of the corneal stroma and its transparency.
Importantly, the contraction assay confirmed that the addition of RA significantly reduced contraction of engineered constructs compared to the control gels. This is another positive finding showing that RA was not only mitogenic, but also maintained keratocytes in a non-contractile phenotype. However, the potentially limiting effects of increased collagen (as shown in the presence of RA) on gel contraction cannot be disregarded. The use of fetal bovine serum in the culture medium to promote cell proliferation results in contraction of the engineered matrixCitation76 that often mimic scarred native tissue due to activation of stromal fibroblasts into myofibroblasts.Citation77 While removal of serum can abrogate this phenomenon, we have demonstrated that further significant loss of contraction can be achieved by the simple addition of RA.
MATERIALS AND METHODS
Human corneal keratocyte isolation
Corneal tissues were obtained from Royal Berkshire Hospital, Reading, UK and Royal Victoria Infirmary, Newcastle, UK. Corneal stroma was excised from cadaverous human limbal tissue following removal of the central 7 mm for keratectomy (donors' age between 39 and 76; average ± SD = 61 ± 12 years, with no prior history of corneal diseases or ocular trauma). The male-female donor ratio was 2:3. Briefly, the epithelia-depleted corneal tissues were minced using a scalpel, transferred to DMEM:F12 medium (Invitrogen, 31331–028) supplemented with 5% fetal bovine serum (FBS; Gibco Invitrogen, 10082–147), 2 g/L (450 units/ml) collagenase type-1 (Invitrogen, 17018–029) and incubated at 37°C under continuous rotation for 5 hours, followed by incubation with 0.25% trypsin-EDTA (Gibco Invitrogen, 25200–072) for 10 minutes. The isolated keratocytes were plated onto tissue culture flasks (Greiner Bio-One, 658175) and maintained using culture medium containing DMEM:F12 medium (Invitrogen), 5% FBS (Gibco Invitrogen), 1 × 10−3 M ascorbic acid (Sigma-Aldrich, A8960), 1 × ITS liquid media supplement (Sigma-Aldrich, I3146) and 1% penicillin/streptomycin (Invitrogen, 15140–122). The culture medium was changed every 2–3 days and cultures were maintained until reaching 70–80% confluence. Cells were then transferred to culture medium without FBS (serum-free medium, SFM) to induce quiescence, and passaged after 3 days. Corneal stromal fibroblasts maintained in serum-containing media have been previously shownCitation56-58 to revert to a more keratocyte-characteristic phenotype, expressing higher keratocyte-specific markersCitation58 while showing reduced cell migration and contractility after a 3-day period of serum starvation.Citation56-58,78 Each subsequent experiment was performed 3 independent times using keratocytes from passages 3 to 5 from specific donors.
Formation of compressed collagen gels with embedded keratocytes
Collagen gels were prepared as previously described.Citation79 2 ml sterile rat-tail collagen type I (2.2 mg/ml in 0.6% acetic acid, First Link Ltd.,60–30–810) and 0.5 ml modified Eagle's minimum essential medium (MEM; Fisher Scientific, 21430–020) were mixed in a centrifuge tube placed on ice. Sodium hydroxide 1 M (Fisher Scientific, J/7620/15) was added drop-wise to neutralize the pH of the mixture, along with intermittent, gentle mixing of the solution until it changed its color from yellow to light pink. The mixture was then cast into 12-well plate molds (Greiner Bio-One, 665180), along with 2.5 × 105 serum-deprived keratocytes in each well prior to polymerization (gelling), which subsequently took place for 30 minutes at 37°C in a humidified 5% CO2 incubator. Next, the polymerised collagen gels containing keratocytes were removed from the molds and placed in between layers of nylon mesh, and the compression was performed at room temperature using a 134 g load for 5 minutes. The resulting compressed collagen gels embedded with keratocytes were then transferred into 6-well plates (Greiner Bio-One, 657160) and cultured using SFM supplemented with either 1 × 10−7 M RA (+RA, Sigma-Aldrich, R2625) or equivalent volume of DMSO vehicle (control; Fisher Scientific, 10080110) for 30 days. The culture medium was changed every 2–3 days.
Proliferation assay
The AlamarBlue® assay was used to assess keratocyte proliferation and viability within the collagen gels with or without supplementation of RA in SFM for 30 days. The gels were incubated with resazurin reagent (Sigma-Aldrich, R7017; prepared in 1:10 dilution using fresh culture medium) for 2 h at 37°C, after which 100 µl of culture supernatants were sampled in triplicate for fluorescence emission analysis at 590 nm using Fluoroskan Ascent fluorescent spectrophotometer (Thermo Labsystems, Franklin, MA), and cells replenished with fresh media. The assay was done on days 3, 7, 21 and 30 of culture. Cell number was calculated by interpolation using a standard curve for fluorescence values of 1, 5, 10, 20, 50, and 100 × 104 cells, and the values correspond to average ± SD of 3 independent experiments.
Hydration study
The weight of all cell-encapsulated collagen gels from RA-supplemented and control groups was measured before starting the experiment to find the initial weight (Wi), and similarly after the 30-day culture period for the final weight (Wf). The ratio of weight increment (wet weight) was then calculated using the following equation (Eq Equation1(1)
(1) ).
(1)
(1)
Next, we used a freeze-drying method to sublimate frozen water from the solid to gas phase under controlled pressure in order to determine the percentage of water bound to these gels. The weight of all freeze-dried gels were again measured to find the dry weight (Wd), which was later used to find the hydration percentage of all gels using the following equation (Eq Equation2(2)
(2) ).
(2)
(2)
This experiment was performed 3 independent times, using 3 replicate gels.
Gel contraction assay
Digital photography was used to image the constructs at the beginning of the experiment and on day 30 of culture. The images were then analyzed using Image J v1.46 (National Institute of Health, Bethesda, MD). For each experiment, 3 collagen gels containing keratocytes which were either cultured in serum-free medium (with RA or DMSO) or standard culture medium with serum (FBS) were evaluated and the average contraction was determined by calculating the percent decrease in surface area compared to the original gel surface area.
Reverse Transcription Polymerase Chain Reaction (RT-PCR) analysis
Following 30 days in culture, the compressed collagen gels with embedded keratocytes were harvested, and RNA was isolated using standard Trizol (Invitrogen, 15596–018) extraction. The assessment of RNA quality was performed using Nanodrop 2000 spectrophotometer (Thermo Scientific, UK) to ensure the 260/280 ratio was within the range of 1.7 to 2.0. Synthesis of cDNA from isolated total RNA was done using the RT2 First Strand kit (Qiagen, 330401) according to the manufacturer's protocol, in a TcPlus thermocycler (Techne, Staffordshire, UK). The polymerase chain reaction (PCR) was carried out using the default thermal profile of the Eco Real-Time System (Illumina, San Diego, CA), with the following 40 × 3-step cycle: 10-second denaturation, 95°C; 30-second annealing, 60°C; 15-second elongation, 72°C. The relative expression of keratocyte genes coding (summarized in ) were calculated by the comparative threshold cycle (CT) (Eco Software v3.1; Illumina) and normalized to the expression of the POLR2A housekeeping gene. Results from 3 independent experiments from 3 different donors were normalized relative to the expression from compressed collagen gels embedded with keratocytes cultured using control media.
TABLE 1. Description of primers used in RT-PCR for keratocyte gene expression analysis
Western blotting
The expression of keratocan, lumican and decorin proteoglycans, ALDH1 and ALDH3 crystallins, carbohydrate sulfotransferase 6 (CHST6), Collagen Type I and V, MMP1 and MMP9 proteases, fibronectin and α-smooth muscle actin (αSMA) were analyzed from day 30 compressed collagen gels embedded with keratocytes in both RA-treated and control groups lysates using ice-cold RIPA lysis buffer supplemented with 1x Proteases Inhibitors Cocktail (Roche, 04693116001) for 10 minutes. Both human donor cornea and keratocytes grown for 7 d in serum-containing medium (bFBS) were used as controls. After precipitation with 4 × volumes of ethanol and pellet resuspension in sample buffer, lysates were run by reducing SDS-PAGE using 10% Mini-Protean precast gels (Bio-Rad, 4561034) and blotted onto polyvinylidene difluoride (PVDF) membranes (Thermo Scientific, 88518). Membranes were then blocked in Tris-buffered saline (Fisher Scientific, 10103203) supplemented with 5% bovine serum albumin (First-Link, 40–00–450) and 0.1% Tween 20 (Fisher Scientific, 10246910), and incubated with primary antibodies against keratocan (Santa Cruz Biotechnology, sc-66941), lumican (kindly given by Dr Bruce Caterson, Cardiff School of Biosciences, Cardiff, UK), decorin (CalBiochem, PC673), ALDH1A1, MMP1, MMP9, CHST6 (Abcam, ab23375, EP1247Y, EP1254, ab154332 respectively), ALDH3A1 (Thermo Scientific, 10304480), αSMA and fibronectin (Vector Labs, VPS281 and VPF705, respectively) diluted 1:500 in blocking solution, followed by corresponding horseradish peroxidise-conjugated secondary antibodies. Anti-tubulin was used for protein loading normalization. Quantification was performed by densitometry analysis of imaged bands using Image J v1.46 (National Institute of Health, Bethesda, MD).
Immunofluorescence staining
Corneal constructs were imaged by immunofluorescence microscopy. Briefly, 7 µm sections of the gels were cut at −20°C using a cryotome (Leica Microsystems, Wetzlar, Germany) and collected on adhesion glass slides (Thermo Scientific, 640-ADH-006). Sections were fixed in 4% paraformaldehyde for 20 minutes prior to incubation with 2% goat serum (Sigma-Aldrich, G9023) and 2% bovine serum albumin (First-Link) at room temperature to block non-specific binding. Sections were then incubated overnight at 4°C with primary antibodies against keratocan, lumican, ALDH1, Collagen type I and V (same as used for Western blotting). Incubation with secondary antibodies was carried out for 1 hour using Alexa Fluor® 488-conjugated goat anti-mouse or anti-rabbit (1:200, Life Technologies, A11001 and A11008 respectively). Finally, the slides were imaged by fluorescence microscopy (Carl Zeiss Meditec, Germany).
Data analysis and statistics
Error bars represent the SD of the mean. Differences between groups were determined using Student's t-test. Significance between groups was established for p < 0.05, denoted as ‘*’ within figures.
CONCLUSION
The greatest challenge in developing a bio-engineered cornea has always been to recapitulate the biological corneal stroma within the artificial scaffold. This study demonstrates that RA can improve the quality of 3D collagen gels as an in vitro model of the corneal stroma. Furthermore, our results move the potential use of RA a step forward suggesting that RA could be used clinically. As RA supplementation induced keratocytes within a 3D collagen gel matrix to increase proteoglycans production, particularly keratocan and lumican, and remain non-contractile, it is possible that RA may help reduce scarring following wound healing by promoting proliferation and replacement with normal keratocytes within corneal stroma without the need for fibroblast/myofibroblast transformation that can alter proteoglycan and collagen production and lead to corneal haziness.
DISCLOSURE OF POTENTIAL CONFLICTS OF INTEREST
No potential conflicts of interest were disclosed.
Funding
This study was supported by Medical Research Council (United Kingdom) Grant MR/K017217/1.
REFERENCES
- Griffith M, Hakim M, Shimmura S, Watsky MA, Li F, Carlsson D, Doillon CJ, Nakamura M, Suuronen E, Shinozaki N, et al. Artificial human corneas: scaffolds for transplantation and host regeneration. Cornea 2002; 21:S54-S61; PMID:12484700; http://dx.doi.org/10.1097/01.ico.0000263120.68768.f8
- Ruberti JW, Zieske JD. Prelude to corneal tissue engineering–gaining control of collagen organization. Prog Retin Eye Res 2008; 27:549-77; PMID:18775789; http://dx.doi.org/10.1016/j.preteyeres.2008.08.001
- Whitcher JP, Srinivasan M, Upadhyay MP. Corneal blindness: a global perspective. Bull World Health Org 2001; 79:214-21; PMID:11285665
- Ousley PJ, Terry MA. Objective screening methods for prior refractive surgery in donor tissue. Cornea 2002; 21:181-8; PMID:11862091; http://dx.doi.org/10.1097/00003226-200203000-00011
- Germain L, Carrier P, Auger FA, Salesse C, Guérin SL. Can we produce a human corneal equivalent by tissue engineering? Prog Retin Eye Res 2000; 19:497-527; PMID:10925241; http://dx.doi.org/10.1016/S1350-9462(00)00005-7
- Shah A, Brugnano J, Sun S, Vase A, Orwin E. The development of a tissue-engineered cornea: biomaterials and culture methods. Pediatr Res 2008; 63:535-44; PMID:18427299; http://dx.doi.org/10.1203/PDR.0b013e31816bdf54
- Orwin EJ, Borene ML, Hubel A. Biomechanical and optical characteristics of a corneal stromal equivalent. J Biomech Eng 2003; 125:439-44; PMID:12968568; http://dx.doi.org/10.1115/1.1589773
- Borene ML, Barocas VH, Hubel A. Mechanical and cellular changes during compaction of a collagen-sponge-based corneal stromal equivalent. Ann Biomed Eng 2004; 32:274-83; PMID:15008375; http://dx.doi.org/10.1023/B:ABME.0000012747.97620.3a
- Wilson SL, Wimpenny I, Ahearne M, Rauz S, El Haj AJ, Yang Y. Chemical and topographical effects on cell differentiation and matrix elasticity in a corneal stromal layer model. Adv Funct Mater 2012; 22:3641-9; http://dx.doi.org/10.1002/adfm.201200655
- Harkness RD. Biological functions of collagen. Biological Reviews 1961; 36:399-455; PMID:13904721; http://dx.doi.org/10.1111/j.1469-185X.1961.tb01596.x
- Cheng W, Yan-hua R, Fang-gang N, Guo-an Z. The content and ratio of type I and III collagen in skin differ with age and injury. Afr J Biotechnol 2013; 10:2524-9
- Fratzl P, Misof K, Zizak I, Rapp G, Amenitsch H, Bernstorff S. Fibrillar structure and mechanical properties of collagen. J Struc Biol 1998; 122:119-22; http://dx.doi.org/10.1006/jsbi.1998.3966
- Viguet-Carrin S, Garnero P, Delmas PD. The role of collagen in bone strength. Osteoporos Int 2006; 17:319-36; PMID:16341622; http://dx.doi.org/10.1007/s00198-005-2035-9
- Ruggiero F, Burillon C, Garrone R. Human corneal fibrillogenesis. Collagen V structural analysis and fibrillar assembly by stromal fibroblasts in culture. Invest Ophthalmol Vis Sci 1996; 37:1749-60; PMID:8759342
- Orwin EJ, Hubel A. In vitro culture characteristics of corneal epithelial, endothelial, and keratocyte cells in a native collagen matrix. Tissue Eng 2000; 6:307-19; PMID:10992428; http://dx.doi.org/10.1089/107632700418038
- Doillon CJ, Watsky MA, Hakim M, Wang J, Munger R, Laycock N, Osborne R, Griffith M. A collagen-based scaffold for a tissue engineered human cornea: physical and physiological properties. Int J Artif Organs 2003; 26:764-73; PMID:14521175
- Li F, Griffith M, Li Z, Tanodekaew S, Sheardown H, Hakim M, Carlsson DJ. Recruitment of multiple cell lines by collagen-synthetic copolymer matrices in corneal regeneration. Biomaterials 2005; 26:3093-104; PMID:15603804; http://dx.doi.org/10.1016/j.biomaterials.2004.07.063
- Mi S, Khutoryanskiy VV, Jones RR, Zhu X, Hamley IW, Connon CJ. Photochemical cross‐linking of plastically compressed collagen gel produces an optimal scaffold for corneal tissue engineering. J Biomed Mater Res A 2011; 99:1-8; PMID:21732526; http://dx.doi.org/10.1002/jbm.a.33152
- Maeda M, Tani S, Sano A, Fujioka K. Microstructure and release characteristics of the minipellet, a collagen-based drug delivery system for controlled release of protein drugs. J Control Release 1999; 62:313-24; PMID:10528069; http://dx.doi.org/10.1016/S0168-3659(99)00156-X
- Chandran PL, Barocas VH. Microstructural mechanics of collagen gels in confined compression: poroelasticity, viscoelasticity, and collapse. J Biomech Eng 2004; 126:152-66; PMID:15179845; http://dx.doi.org/10.1115/1.1688774
- Brown RA, Wiseman M, Chuo CB, Cheema U, Nazhat SN. Ultrarapid engineering of biomimetic materials and tissues: Fabrication of nano‐and microstructures by plastic compression. Adv Funct Mater 2005; 15:1762-70; http://dx.doi.org/10.1002/adfm.200500042
- Mi S, Chen B, Wright B, Connon CJ. Plastic compression of a collagen gel forms a much improved scaffold for ocular surface tissue engineering over conventional collagen gels. J Biomed Mater Res A 2010; 95:447-53; PMID:20648540; http://dx.doi.org/10.1002/jbm.a.32861
- Jones RR, Hamley IW, Connon CJ. Ex vivo expansion of limbal stem cells is affected by substrate properties. Stem Cell Res 2012; 8:403-9; PMID:22386779; http://dx.doi.org/10.1016/j.scr.2012.01.001
- Levis HJ, Brown RA, Daniels JT. Plastic compressed collagen as a biomimetic substrate for human limbal epithelial cell culture. Biomaterials 2010; 31:7726-37; PMID:20674002; http://dx.doi.org/10.1016/j.biomaterials.2010.07.012
- Mi S, Chen B, Wright B, Connon CJ. Ex vivo construction of an artificial ocular surface by combination of corneal limbal epithelial cells and a compressed collagen scaffold containing keratocytes. Tissue Eng Part A 2010; 16:2091-100; PMID:20109018; http://dx.doi.org/10.1089/ten.tea.2009.0748
- Guo Q, Phillip JM, Majumdar S, Wu PH, Chen J, Calderón-Colón X, Schein O, Smith BJ, Trexler MM, Wirtz D, et al. Modulation of keratocyte phenotype by collagen fibril nanoarchitecture in membranes for corneal repair. Biomaterials 2013; 34:9365-72; PMID:24041426; http://dx.doi.org/10.1016/j.biomaterials.2013.08.061
- Thompson RE, Boraas LC, Sowder M, Bechtel MK, Orwin EJ. Three-dimensional cell culture environment promotes partial recovery of the native corneal keratocyte phenotype from a subcultured population. Tissue Eng Part A 2013; 19:1564-72; PMID:23410050; http://dx.doi.org/10.1089/ten.tea.2012.0084
- Lakshman N, Petroll WM. Growth factor regulation of corneal keratocyte mechanical phenotypes in 3-D collagen matrices. Invest Ophthalmol Vis Sci 2012; 53:1077; PMID:22247479; http://dx.doi.org/10.1167/iovs.11-8609
- Fini ME. Keratocyte and fibroblast phenotypes in the repairing cornea. Prog Retin Eye Res 1999; 18:529-51; PMID:10217482; http://dx.doi.org/10.1016/S1350-9462(98)00033-0
- He J, Bazan HEP. Epidermal growth factor synergism with TGF-β1 via PI-3 Kinase activity in corneal keratocyte differentiation. Invest Ophthalmol Vis Sci 2008; 49:2936-45; PMID:18579759; http://dx.doi.org/10.1167/iovs.07-0900
- West-Mays JA, Dwivedi DJ. The keratocyte: corneal stromal cell with variable repair phenotypes. Int J Biochem Cell Biol 2006; 38:1625-31; PMID:16675284; http://dx.doi.org/10.1016/j.biocel.2006.03.010
- Müller LJ, Pels L, Vrensen GF. Novel aspects of the ultrastructural organization of human corneal keratocytes. Invest Ophthalmol Vis Sci 1995; 36:2557-67; PMID:7499078
- Barry PA, Cavanagh HD, Jester JV. Effect of serum, BFGF, TGF(Beta-1) and heparin on in-vitro myofibroblast transformation in rabbit corneal keratocytes. Invest Ophthalmol Vis Sci: Lippincott-Raven Publ 227 East Washington SQ, Philadelphia, PA 19106, 1994:1356
- Beales MP, Funderburgh JL, Jester JV, Hassell JR. Proteoglycan synthesis by bovine keratocytes and corneal fibroblasts: maintenance of the keratocyte phenotype in culture. Invest Ophthalmol Vis Sci 1999; 40:1658-63; PMID:10393032
- Berryhill BL, Kader R, Kane B, Birk DE, Feng J, Hassell JR. Partial restoration of the keratocyte phenotype to bovine keratocytes made fibroblastic by serum. Invest Ophthalmol Vis Sci 2002; 43:3416-21; PMID:12407151
- Jester JV, Barry-Lane PA, Cavanagh HD, Petroll WM. Induction of [α]-Smooth Muscle Actin Expression and Myofibroblast Transformation in Cultured Corneal Keratocytes. Cornea 1996; 15:505-16; PMID:8862928; http://dx.doi.org/10.1097/00003226-199609000-00011
- Maltseva O, Folger P, Zekaria D, Petridou S, Masur SK. Fibroblast growth factor reversal of the corneal myofibroblast phenotype. Invest Ophthalmol Visual Sci 2001; 42:2490-5; PMID:11581188
- Long CJ, Roth MR, Tasheva ES, Funderburgh M, Smit R, Conrad GW, et al. Fibroblast growth factor-2 promotes keratan sulfate proteoglycan expression by keratocytes in vitro. J Biol Chem 2000; 275:13918-23; PMID:10788517; http://dx.doi.org/10.1074/jbc.275.18.13918
- Karamichos D, Hutcheon AEK, Zieske JD. Reversal of fibrosis by TGF-β3 in a 3D in vitro model. Exp Eye Res 2014; 124:31-6; PMID:24800655; http://dx.doi.org/10.1016/j.exer.2014.04.020
- Kastner P, Grondona JM, Mark M, Gansmuller A, LeMeur M, Decimo D, Vonesch JL, Dollé P, Chambon P. Genetic analysis of RXRα developmental function: convergence of RXR and RAR signaling pathways in heart and eye morphogenesis. Cell 1994; 78:987-1003; PMID:7923367; http://dx.doi.org/10.1016/0092-8674(94)90274-7
- Hofmann C, Eichele G. Retinoids in development. Retinoids: Biol Chem Med 1994; 2:387-441
- Lohnes D, Mark M, Mendelsohn C, Dolle P, Dierich A, Gorry P, Gansmuller A, Chambon P. Function of the retinoic acid receptors (RARs) during development (I). Craniofacial and skeletal abnormalities in RAR double mutants. Development 1994; 120:2723-48; PMID:7607067
- Hassell JR, Newsome DA, De Luca LM. Increased biosynthesis of specific glycoconjugates in rat corneal epithelium following treatment with vitamin A. Invest Ophthalmol Vis Sci 1980; 19:642-7; PMID:7380623
- Stonecipher KG, Jensen HG, Rowsey JJ, Nordquist RE. Topical application of all-trans-retinoic acid. Graefe's Arch Clin Exp Ophthamol 1988; 226:371-6; http://dx.doi.org/10.1007/BF02172970
- Smith J, Steinemann TL. Vitamin A deficiency and the eye. Int Ophthalmol Clin 2000; 40:83-91; PMID:11064859; http://dx.doi.org/10.1097/00004397-200010000-00007
- Samarawickrama C, Chew S, Watson S. Retinoic acid and the ocular surface. Surv Ophthalmol 2015; 60:183-95; PMID:25890622; http://dx.doi.org/10.1016/j.survophthal.2014.10.001
- Popp C, Kligman AM, Stoudemayer TJ. Pretreatment of photoaged forearm skin with topical tretinoin accelerates healing of full‐thickness wounds. Br J Dermatol 1995; 132:46-53; PMID:7756151; http://dx.doi.org/10.1111/j.1365-2133.1995.tb08623.x
- Wicke C, Halliday B, Allen D, Roche NS, Scheuenstuhl H, Spencer MM, Roberts AB, Hunt TK. Effects of steroids and retinoids on wound healing. Arch Surg 2000; 135:1265-70; PMID:11074878; http://dx.doi.org/10.1001/archsurg.135.11.1265
- Kitano Y, Yoshimura K, Uchida G, Sato K, Harii K. Pretreatment with topical all-trans-retinoic acid is beneficial for wound healing in genetically diabetic mice. Arch Dermatol Res 2001; 293:515-21; PMID:11820728; http://dx.doi.org/10.1007/PL00007466
- Mehra KS, Mikuni I, Kumar A. Effects of vitamin A and cortisone on healing of corneal penetrating wounds. Tokai J Exp Clin Med 1982; 7:319-23; PMID:7123571
- Johansen S, Heegaard S, Prause JU, Rask‐Pedersen E. The healing effect of all‐trans retinoic acid on epithelial corneal abrasions in rabbits. Acta Ophthalmol Scand 1998; 76:401-4; PMID:9716324; http://dx.doi.org/10.1034/j.1600-0420.1998.760403.x
- Hattori M, Shimizu K, Katsumura K, Oku H, Sano Y, Matsumoto K, Yamaguchi Y, Ikeda T. Effects of all-trans retinoic acid nanoparticles on corneal epithelial wound healing. Graefe's Arch Clin Exp Ophthamol 2012; 250:557-63; http://dx.doi.org/10.1007/s00417-011-1849-8
- Gouveia RM, Connon CJ. The effects of retinoic acid on human corneal stromal keratocytes cultured in vitro under serum-free conditions. Invest Ophthalmol Vis Sci 2013; 54:7483-91; PMID:24150763; http://dx.doi.org/10.1167/iovs.13-13092
- Guo X, Hutcheon AEK, Melotti SA, Zieske JD, Trinkaus-Randall V, Ruberti JW. Morphologic characterization of organized extracellular matrix deposition by ascorbic acid–stimulated human corneal fibroblasts. Invest Ophthalmol Vis Sci 2007; 48:4050-60; PMID:17724187; http://dx.doi.org/10.1167/iovs.06-1216
- Musselmann K, Kane B, Alexandrou B, Hassell JR. Stimulation of collagen synthesis by insulin and proteoglycan accumulation by ascorbate in bovine keratocytes in vitro. Invest Ophthalmol Vis Sci 2006; 47:5260-6; PMID:17122111; http://dx.doi.org/10.1167/iovs.06-0612
- Builles N, Bechetoille N, Justin V, Ducerf A, Auxenfans C, Burillon C, Sergent M, Damour O. Development of an optimised culture medium for keratocytes in monolayer. Biomed Mater Eng 2006; 16:S95-S104; PMID:16823117
- Jester JV, Ho-Chang J. Modulation of cultured corneal keratocyte phenotype by growth factors/cytokines control in vitro contractility and extracellular matrix contraction. Exp Eye Res 2003; 77:581-92; PMID:14550400; http://dx.doi.org/10.1016/S0014-4835(03)00188-X
- Foster JW, Gouveia RM, Connon CJ. Low-glucose enhances keratocyte-characteristic phenotype from corneal stromal cells in serum-free conditions. Sci Rep 2015; 5:10839
- Fullwood NJ, Davies Y, Nieduszynski IA, Marcyniuk B, Ridgway AE, Quantock AJ. Cell surface-associated keratan sulfate on normal and migrating corneal endothelium. Invest Ophthalmol Vis Sci 1996; 37:1256-70; PMID:8641829
- Iozzo RV. The family of the small leucine-rich proteoglycans: key regulators of matrix assembly and cellular growth. Crit Rev Biochem Mol Biol 1997; 32:141-74; PMID:9145286; http://dx.doi.org/10.3109/10409239709108551
- Svensson L, Närlid I, Oldberg Å. Fibromodulin and lumican bind to the same region on collagen type I fibrils. FEBS Lett 2000; 470:178-82; PMID:10734230; http://dx.doi.org/10.1016/S0014-5793(00)01314-4
- Downes JE, Swann PG, Holmes RS. Ultraviolet light-induced pathology in the eye: associated changes in ocular aldehyde dehydrogenase and alcohol dehydrogenase activities. Cornea 1993; 12:241-8; PMID:8500338; http://dx.doi.org/10.1097/00003226-199305000-00010
- Pappa A, Estey T, Manzer R, Brown D, Vasiliou V. Human aldehyde dehydrogenase 3A1 (ALDH3A1): biochemical characterization and immunohistochemical localization in the cornea. Biochem J 2003; 376:615-23; PMID:12943535; http://dx.doi.org/10.1042/bj20030810
- Estey T, Piatigorsky J, Lassen N, Vasiliou V. ALDH3A1: a corneal crystallin with diverse functions. Exp Eye Res 2007; 84:3-12; PMID:16797007; http://dx.doi.org/10.1016/j.exer.2006.04.010
- Jester JV, Moller-Pedersen T, Huang J, Sax CM, Kays WT, Cavangh HD, Petroll WM, Piatigorsky J. The cellular basis of corneal transparency: evidence for ‘corneal crystallins’. J Cell Sci 1999; 112:613; PMID:9973596
- Jester JV, Barry PA, Lind GJ, Petroll WM, Garana R, Cavanagh HD. Corneal keratocytes: in situ and in vitro organization of cytoskeletal contractile proteins. Invest Ophthalmol Vis Sci 1994; 35:730; PMID:8113024
- Pei Y, Reins RY, McDermott AM. Aldehyde dehydrogenase (ALDH) 3A1 expression by the human keratocyte and its repair phenotypes. Experimental Eye Research 2006; 83:1063-73; PMID:16822507; http://dx.doi.org/10.1016/j.exer.2006.05.011
- Feng Y, Feng Y, Zhu X, Dang Y, Ma Q. Alkali burn causes aldehyde dehydrogenase 3A1 (ALDH3A1) decrease in mouse cornea. Mol Vis 2004; 10:845-50; PMID:15547490
- Jester JV, Budge A, Fisher S, Huang J. Corneal keratocytes: phenotypic and species differences in abundant protein expression and in vitro light-scattering. Invest Ophthalmol Vis Sci 2005; 46:2369; PMID:15980224; http://dx.doi.org/10.1167/iovs.04-1225
- Di Iorio E, Barbaro V, Volpi N, Bertolin M, Ferrari B, Fasolo A, Arnaldi R, Brusini P, Prosdocimo G, Ponzin D, et al. Localization and expression of CHST6 and keratan sulfate proteoglycans in the human cornea. Exp Eye Res 2010; 91:293-9; PMID:20537995; http://dx.doi.org/10.1016/j.exer.2010.06.001
- Jones ST, Zimmerman LE. Histopathologic differentiation of granular, macular and lattice dystrophies of the cornea. Am J Ophthalmol 1961; 51:394-410; PMID:13790593; http://dx.doi.org/10.1016/0002-9394(61)92085-2
- Klintworth GK. Research into the pathogenesis of macular corneal dystrophy. Trans Ophthalmol Soc U K 1980; 100:186-94; PMID:6973846
- Lateef H, Stevens MJ, Varani J. All-trans-retinoic acid suppresses matrix metalloproteinase activity and increases collagen synthesis in diabetic human skin in organ culture. Am J Pathol 2004; 165:167-74; PMID:15215172; http://dx.doi.org/10.1016/S0002-9440(10)63285-3
- Fini ME, Girard MT, Matsubara M. Collagenolytic/gelatinolytic enzymes in corneal wound healing. Act Ophthalmol 1992; 70:26-33; http://dx.doi.org/10.1111/j.1755-3768.1992.tb02165.x
- Girard MT, Matsubara M, Kublin C, Tessier MJ, Cintron C, Fini ME. Stromal fibroblasts synthesize collagenase and stromelysin during long-term tissue remodeling. J Cell Sci 1993; 104:1001; PMID:8314885
- Borderie VM, Mourra N, Laroche L. Influence of fetal calf serum, fibroblast growth factors, and hepatocyte growth factor on three-dimensional cultures of human keratocytes in collagen gel matrix. Graefe's Arch Clin Exp Ophthamol 1999; 237:861-9; http://dx.doi.org/10.1007/s004170050324
- Jester JV, Petroll WM, Barry PA, Cavanagh HD. Expression of α-smooth muscle (α-SM) actin during corneal stromal wound healing. Invest Ophthalmol Vis Sci 1995; 36:809-19; PMID:7706029
- Gouveia RM, Jones RR, Hamley IW, Connon CJ. The bioactivity of composite Fmoc-RGDS-collagen gels. Biomaterials Sci 2014; 2:1222-9; http://dx.doi.org/10.1039/C4BM00121D
- Foster JW, Jones RR, Bippes CA, Gouveia RM, Connon CJ. Differential nuclear expression of Yap in basal epithelial cells across the cornea and substrates of differing stiffness. Exp Eye Res 2014; 127:37-41; PMID:24992208; http://dx.doi.org/10.1016/j.exer.2014.06.020