ABSTRACT
Bone defects or fractures generally heal in the absence of major interventions due to the high regenerative capacity of bone tissue. However, in situations of severe/large bone defects, these orchestrated regeneration mechanisms are impaired. With advances in modern medicine, natural and synthetic bio-scaffolds from bioceramics and polymers that support bone growth have emerged and gained intense research interest. In particular, scaffolds that recapitulate the molecular cues of extracellular signals, particularly growth factors, offer potential as therapeutic bone biomaterials. The current challenges for these therapies include the ability to engineer materials that mimic the biological and mechanical properties of the real bone tissue matrix, whilst simultaneously supporting bone vascularization. In this review, we discuss the very recent innovative strategies in bone biomaterial technology, including those of endogenous biomaterials and cell/drug delivery systems that promote bone regeneration. We present our understanding of their current value and efficacy, and the future perspectives for bone regenerative medicine.
Introduction
The higher life expectancy of the human population has increased the need for bone interventions.Citation1 Due the limitations of current techniques to reconstruct bone defects, and the development of novel therapeutics, a step-change has occurred in bone research, namely regenerative medicine.Citation2 Regenerative medicine merges biological sciences with fundamental engineering techniques to develop novel biological substitutes that can benefit human health. This field has benefitted from advances in material engineering, our heightened understanding of bone physiology, and our knowledge of mesenchymal stem cells (MSCs), as alternative therapies for the treatment of bone defects.Citation3 MSCs are beneficial due to their ability to secrete trophic factors that promote bone repair.Citation4 Regenerative medicine involves a combination of biomaterials/scaffolds, cells, and bioactive agents, all of which show therapeutic promise for the repair of damaged bone.Citation4, Citation5 The ultimate aim of regenerative medicine is to cure previously untreatable bone injuries and diseases, increasing the arsenal of medical strategies available for clinical use.
Bone defects produced by large bone tumor resections, trauma-induced nonunion fractures, biochemical disorders, infections, or abnormal skeletal development due to genetic disorders, are a major cause of disability and a loss of quality of life globally.Citation6 The treatment of bone defects to recover normal bone morphology and function represents an important and unmet clinical challenge, particularly when bone healing is impaired.Citation7 There are several reasons for bone healing defects, including bone loss due to injury, impaired vascularization, dysregulated immune responses, infection and osteomyelitis.Citation8 Surgical techniques, including the implantation of synthetic bone substitutes and bone graft implants, have been developed to aid bone recovery.Citation9 Bone grafting replaces the missing bone during surgery, and as a procedure, its demand is widespread, second only to blood transplants in terms of treatment frequency.Citation7, Citation10 Autographs remain the front line therapy but their attainability is complex and their effectiveness is limited by the associated morbidity during harvesting and poor clinical performance, particularly in osteoporosis patients.Citation11 Therefore, the development of new, effective and safer alternatives is urgently required in the field of bone regenerative therapy.
Mechanisms of bone healing
To fully understand the new therapeutic tools emerging for bone regeneration, the mechanisms of bone repair and regeneration must first be appreciated. Bone healing involves the synchronized interaction of numerous growth factors, cells and the extracellular matrix (ECM) that occur in a series of orchestrated stages in response to bone trauma.Citation12 The three major stages of bone repair include: (1) Inflammatory phase; (2) fibrovascular phase; and (3) bone formation ().Citation12–14
Figure 1. Stages of biomaterial-mediated bone repair. Bone defects can originate from a variety of causes (outlined). Natural or synthetic scaffolds (hydrogels in this example) can aid bone repair. These biomaterials possess osteoconductivity, biodegradability, controlled growth factor release, and cell encapsulation and can be produced from a range of composite materials including metals, ceramics, or polymers. Vascularization is vital to biomaterial design since impaired revascularization delays bone healing
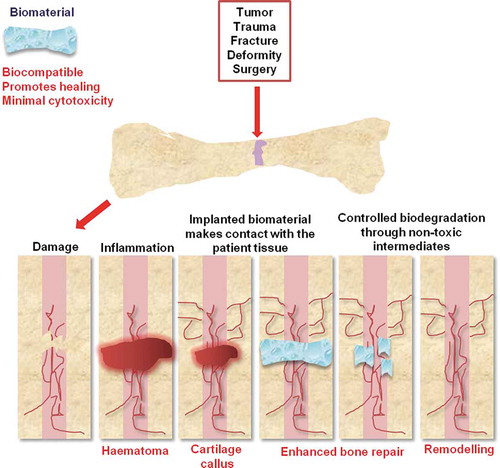
Inflammatory phase
The inflammatory cells, as well as the cytokines and ECM produced by them, play crucial roles in bone repair. A fibrin-rich blood clot forms immediately following the fracture and the cytokines released by the clot recruit inflammatory cells such as lymphocytes, macrophages, eosinophils, and neutrophils to the injury site.Citation15 Additionally, neutrophils and macrophages play a role in the debridement of necrotic tissue. Macrophages secrete the pro-inflammatory Interleukin 1 (IL1), which in turn modulates the expression of cyclooxygenases, which are involved in synthesis of prostaglandins in injured tissues.Citation16 Depletion of macrophages results in defects in fracture healing due to reduced callus size, osteogenesis and chondrogenesis.Citation17 Other pro-inflammatory factors such as Tumor Necrosis Factor alpha (TNF-alpha) and Interleukin 6 (IL6) have also been implicated in bone healing. Inflammatory cells also produce growth factors such as Fibroblast Growth Factor (FGF), Platelet-Derived Growth Factor (PDGF) and Transforming Growth Factor beta (TGF-β), which initiate the repair by inducing proliferation and differentiation of the MSCs that give rise to the fracture callus.Citation14
While the inflammatory phase begins during the earliest stages of bone repair, it has been recently demonstrated that the inflammatory cells are also present throughout later healing phases.Citation18 This phenomenon may be explained by changes between pro- to anti-inflammatory states of macrophages. Initially, pro-inflammatory or classically activated macrophages (CAMs) are produced by the immune response to tissue injury.Citation19 This results in upregulation of the pro-inflammatory cytokines TNF-alpha, IL1 and IL6 through the NFkB pathway. After wound debridement, anti-inflammatory or alternatively activated macrophages (AAMs) are generated through IL4 and IL13 signaling. In contrast to CAMs, AAMs promote collagen deposition and tissue homeostasis.Citation20 The multifactorial role of the acute pro-inflammatory response contributes to healing and inhibition of inflammation delays fracture repair.Citation14
Fibrovascular phase
The fibrovascular phase is characterized by vascular remodeling and recruitment of MSCs, which differentiate into chondrocytes and osteoblasts to drive bone regeneration.Citation12 Revascularization is essential for supplying the callus with oxygen, nutrients, inflammatory and progenitor cells to aid repair and the removal of wastes.Citation21 This process occurs by angiogenesis (new blood vessels are formed by from existing vasculature) and vasculogenesis (formation of blood vessels from endothelial progenitor cells (EPCs) in the callus). Vascular endothelial growth factor (VEGF), produced by a variety of cells, including inflammatory cells, osteoblasts and hypertrophic chondrocytes, is a driver of revascularization.Citation22 It binds to its receptors VEGFR1 and VEGFR2, activating signaling cascades which stimulate the recruitment of EPCs to the fracture. The ECM can also influence the angiogenic response to fracture healing. For example, thrombospondins (TSP) are matrix proteins with a potent anti-angiogenic activity.Citation23
MSCs act as osteochondral progenitor cells and are recruited to the fracture callus locally from the periosteum and bone marrow.Citation24 Recruitment of MSCs for fracture repair is regulated by cytokines released at the fracture site, particularly stromal cell-derived factor 1 (SDF1). SDF1 drives homing of MSCs through CXCR4.Citation25 Partial disruption of SDF1/CXCR4 inhibits MSC chemotaxis and bone formation. Since SDF1 is under transcriptional regulation by hypoxia inducible factor 1-alpha (HIF1-alpha) in response to ischemia, the hypoxic conditions of the fracture may direct MSC recruitment and vascularization.Citation26
Bone formation
After the fibrovascular phase, MSCs formed in the callus differentiate to either osteoblasts or chondrocytes to initiate bone formation. MSC differentiation is initially regulated by the transcription factor Sox9.Citation27, Citation28 Sox9 inhibits osteogenic activity by repressing Runx2 (regulator of osteochondral progenitor cells), hence this acts as a switch between chondrogenic or osteogenic fates in osteochondral progenitor cells.Citation29 Mechanical factors and oxygen tension are the significant cell-extrinsic factors which regulate the chondrogenic or osteogenic fate of MSCs. Greater motion predominantly induces the generation of chondrocytes, whereas stabilization induces the formation of osteoblasts and bone repair.Citation30 Hypoxia promotes chondrogenesis, while high oxygen levels promote osteoblastogenesis.Citation31
The dual role of BMP signaling in the regulation of both osteoblast and chondrocyte differentiation of MSCs has not been entirely elucidated in the context of fracture repair. BMPs may play a key role in the calcification of the cartilage matrix via both cell-autonomous and paracrine effects.Citation32 After calcification of the cartilage matrix, bone matrix is formed and the hypertrophic chondrocytes in the bone matrix are converted into osteocytes.Citation33 It was recently demonstrated that chondrocytes differentiate into osteocytes in the growth plate during development as well as bone repair.Citation33 Possibly, chondrocyte apoptosis and invading osteoblasts may contribute to the new bone at the fracture site.
Remodeling of the bony callus by osteoclasts to form the mature lamellar bone is the last stage of fracture repair.Citation34 Osteoclast-mediated degradation of the bone yields bone-sequestered factors (TGF-beta) and factors produced by the osteoclasts (complement 3a, Wnt10b, BMP6, and SLIT3) which are critical in the subsequent osteogenesis.Citation35 Finally, resorption ends with the osteoclast apoptosis. Monocyte/macrophage colony stimulating factor (MCSF) and Receptor Activator of Nuclear Factor kappaB Ligand (RANKL) as well as several other cytokines and signaling pathways are required for osteoclast formation, differentiation and survival.Citation14 In addition, degradation of the cartilage callus may be accomplished indirectly through MMPs (MMP9 and MMP13) expressed by osteoblasts and chondrocytes.Citation36
Ideal features of biomaterials for bone regeneration
The term ‘osteoconductivity’ defines the property of a biomaterial that provides a scaffold for new bone formation.Citation37 Biomaterials must bind and attract relevant cells for bone healing (). ‘Osteogenesis’ is the process by which a biomaterial actively supports de novo bone genesis through its ability to recruit osteoblasts. This is achieved through the biomaterial supporting osteogenic differentiation through enhancing cell attachment and differentiation, providing a stress-relaxation pattern that enhances bone formation.Citation38–41 Synthetic or natural biomaterials can enhance the restoration of bone structure and function.Citation42 Implant materials make contact with the patient tissue permitting interactions with endogenous bone through chemical, physical or biological processes.Citation10, Citation11 Owing to tissue contact, the biocompatibility of implant biomaterials is crucial.Citation43 Controlled biodegradation through nontoxic intermediates is also critical, since harmful by-products can slow the healing process and promote off-target cytotoxicity.Citation44 Bone can be reconstructed with both vascularized and non-vascularized autologous fibular grafts.Citation45 Vascularization is vital to biomaterial design since impaired revascularization delays bone healing. For a biomaterial to be effective, it must not trigger angiogenesis or obstruct natural bone healing processes, but must promote bone repair by providing bone stability and an osteoinductive and osteogenic environment.
Figure 2. Biomaterial-based approaches for bone tissue engineering. Scaffolds that recapitulate the molecular cues of extracellular signals, particularly growth factors, are emerging as exciting bone biomaterials. Scaffolds can be surface modified to enhance growth factor, stem cell, drug, or biomolecule binding, and when implanted, concentrate these factors at the site of the injury to aid bone repair
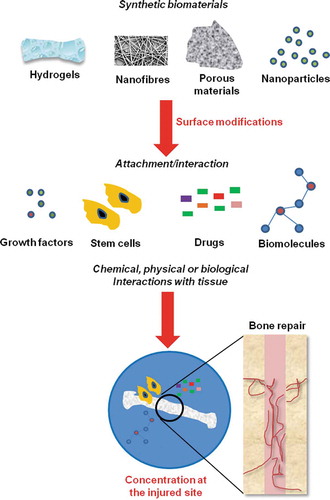
Biodegradability is related to porosity in porous biomaterials since the amplified surface area promotes interactions between the biomaterial and adjacent cells and molecules.Citation13 In addition, the similar the mechanical properties of a biomaterial are to those of the natural tissues, the greater the probability of avoiding adverse effects.Citation3 If the biomaterial properties are weaker than the adjacent tissues, the tissues may compensate which hinders bone repiar and damages the tissues. Hence, it is crucial to optimize the mechanical characteristics of biomaterials.
Emerging biomaterials to treat bone defects
To-date, numerous biomaterials for bone regeneration have been investigated (). Biomaterials can also be coupled with drugs, growth factors and osteogenic progenitor cells, but the environment onto which the biomaterial is introduced must be considered.Citation60
Table 1. Examples of bone biomaterials and their biocompatibility
Endogenous biomaterials
In recent years, more effective biomaterials have emerged, including composite matrices, hydroxyapatites and biomimetic organoids, which highlight movement in the direction of biomaterials that more closely mimic endogenous tissue.Citation45 Such approaches have been made more feasible by our enhanced understanding of bone healing and innovative technological advances. This was highlighted in recent studies by Cassino and coworkers who demonstrated the therapeutic potential of different hydroxyapatites (HAs), the major components of normal bone and teeth, to correct severe bone defects.Citation61 De Luca et al. produced composite poly L-lactic acid/nano-HA scaffolds that could promote the osteogenic differentiation of hMSCs for the repair of bone defects.Citation50 In addition, Ding and colleagues assessed the utility of silk-HA composite materials by blending nanofibers, hydrogels and water-dispersible silk-HA nanoparticles to produce injectable and homogeneous nanosytems.Citation55, Citation56 The composite hydrogels supported osteogenesis and bone healing, which highlighted their usefulness for the regeneration of irregular bone defects. Yang et al.Citation54 developed surface-modified HA nanoparticle-reinforced polylactides for 3D-printed bone tissue engineering scaffolds, whilst Kumai et al.Citation62 produced self-assembled artificial cartilage-HA conjugates for simultaneous articular cartilage and subchondral bone repair effective in rat models.
To enhance bone formation, mechanical strength, and the functional performance of large bone defects, Oryan et al. assessed the regenerative potential of Ca2+ silicate biomaterials produced by reacting calcium oxide and silica in various ratios in combination with the linear polysaccharide chitosan.Citation63 The bilaterally implanted Ca2+ silicate bio-implants greatly improved radial bone healing and growth. Lui et al. similarly produced bioactive tetracalcium phosphate/magnesium phosphate composite bone cements that enhanced bone repair.Citation51 Importantly, these materials were biodegradable. In alternative approaches, Munoz and colleagues demonstrated that non-setting injectable biomaterials loaded with ceramic particles enhance the stability of bone screws.Citation64 These materials were unique as they permitted screw augmentation without impairing or favoring bone formation in a particular direction around the screw. This approach is principally advantageous for treating osteoporotic fractures that require screw stabilization.
Kaur et al. produced lactic-co-glycolic acid (PLGA)-based carbon nanotubes (CNTs-1D), graphene nanoplatelets (GNPs-2D), and activated carbon (AC-3D) bio-implants.Citation44 The ability of these composites to repair tissue was characterized and their physicochemical, biological, and mechanical properties were compared. GNPs displayed the greatest effects most likely due to their larger surface area.Citation44 Neuforth et al. described the preparation of a morphogenetically-active bioink that consisted of amorphous microparticles (MPs) fabricated from Ca2+ and the physiological polymer, polyphosphate (polyP).Citation65 The MPs were fortified by adding polycaprolactone (PCL) to permit 3D-bioprinting. The resultant PCL/Ca-polyP-MP hybrid was used to 3D-print onto tissue-like scaffolds and showed good biomechanical properties, similar to those of cortical and trabecular bones. The scaffolds also promoted the growth of human bone-related SaOS-2 cells and upregulated the steady-state-expression of the cell migration-inducing chemokine SDF-1α. This study was particularly promising as PCL is an FDA-approved organic polymer. The use of polyP can enhance its physiological functions, offering hope for bone remodeling and regeneration therapies.
Cell-based systems
Biomaterials can be designed to trap cells, altering their functions to complete specific duties that they would otherwise be incapable of performing (). Human MSCs (hMSCs) have the capacity to differentiate into osteoblasts, adipocytes, and chondrocytes.Citation60 Chu and colleagues assessed the efficacy of bioactive MSCs and porous beta-tricalcium phosphate (beta-TCP) composites (MSCs/beta-TCP) to repair tibia defects.Citation66 The MSCs/beta-TCPs showed promising therapeutic efficacy and safety in goat models. Sponer et al. demonstrated that the combination of autologous MSCs and a beta-TCP biomaterial induced trabecular remodeling in human femoral defects.Citation67 In human clinical trials, Gomez-barrena and colleagues highlighted the safety and viability of surgical implants of biphasic Ca3(PO4)2 bioceramic granules during surgery with autologous MSCs expanded from bone marrow.Citation68 Following the assessment of 28 patients with femur, tibia or humerus diaphyseal or metaphyso-diaphyseal nonunions, no severe adverse events were reported and almost all the patients were radiologically healed a year after the procedure. Thus, both the safety and feasibility of the implant system were clinically proven.
In more recent studies, Akilal and colleagues used cowrie’s shell derived powder (CSDP) as a natural bone biomaterial and through structural and physicochemical analysis, highlighted its bioactive features.Citation69 The CSDP boosted stem cell proliferation, highlighting its versatility as a carrier/scaffold for bone tissue engineering. Zinc (Zn)-based biomaterials continue to attract research attention as cell-bearing medical implants that have similar mechanical properties to bone. Zhu and colleagues studied the biological responses of hMSCs to Zn and its underlying cell signaling mechanisms.Citation70 Zn2+ was found to activate the cAMP-PKA pathway and trigger intracellular Ca2+ signaling, resulting in MAPK activation. Additionally, Zn2+ activated the Gαq-PLC-AKT pathway. The combination of these effects led to the expression of genes that enhanced cell growth and differentiation, ECM mineralization, osteogenesis and other cellular processes.
Bone marrow-derived mesenchymal stem cells (BMSCs) are widely used for bone repair in their harvested state or following in vitro expansion.Citation60 Hassan et al. reported the unusual circadian rhythm of BMSCs that could be induced by modified titanium-based biomaterials. These have frequently been employed for dental and orthopedic implants.Citation52 When cultured onto titanium-based biomaterials, hBMSCs suppress normal circadian patterns, which may be vital for their integration into bone. Indeed in animal studies, Deluiz and colleagues demonstrated that hBMSCs seeded into bone biomaterials direct rapid mandibular bone augmentation in rats.Citation71 Mebarki el al. demonstrated that hBMSC adhesion is two-fold greater on cancellous devitalized Tutoplast®-processed bone than HA/beta-TCP.Citation72It is now established that rare earth elements promote osteogenesis and stimulate the repair of bone defects. Zhu et al. investigated the bone regeneration effects of gadolinium (Gd)-doped bioglass produced through the combination of hollow mesoporous Gd-BG microspheres with chitosan.Citation46 In cranial defect rat models, the Gd-BG scaffolds promoted the proliferation and osteogenic differentiation of hBMSCs. At the molecular level, the Gd-BG scaffolds acitviated Akt/GSK3beta signaling to mediate these effects. This highlighted the promise of Gd-BG scaffolds in bone tissue engineering therapy. Similarly, periosteum-derived stem cells (hPDSCs) induced fracture healing of mouse critical-size tibial defects in just 6 days of BMP2 stimulation in vitro.Citation73 In addition, the implanted hPDSCs recruited inflammatory cells in the fracture site in the host.
Drug-loaded biomaterials
In addition to scaffolds that interact with cells to induce specific functions for bone regeneration, scaffolds can also be used to deliver drugs to the damaged area.Citation74, Citation75 A popular approach is to release the loaded drugs at definite time points in response to release signals or stimuli such as electrochemical triggers, pH, and irradiation. Bioactive factors, such as small molecule drugs, cytokines, peptides, and proteins, can be loaded onto the delivery systems, exerting synergistic effects to promote osteoblast proliferation, differentiation, and bone regeneration.Citation60 Several stimuli-responsive biomaterials have been developed for this purpose; an example being the poly(ethylene glycol) hydrogel loaded with drugs that display tunable drug-release properties according to the rates of hydrogel erosion.Citation76 This therapeutic avenue holds potential for bone regeneration and orthopedic implants.
In osteosarcoma patients, the challenge is to achieve successful bone regeneration along with the pharmacological control of cancer cell growth. In this regard, cyclodextrins loaded with chemotherapeutics that release drugs over time, demonstrate promise. A recent example of this strategy was highlighted by Bischoff and colleagues for tumor-induced bone defects.Citation74 The authors evaluated such drug-delivery systems and found that doxorubicin (Dox) eluted from cyclodextrin-coated HA displayed higher efficacy and more prolonged cytotoxic effects on osteosarcoma cells compared to Dox alone.Citation74 The biomaterial promoted vascularization, protecting the endothelial cells and primary osteoblasts from Dox-induced cytotoxicity. These exciting findings indicate how the HA-cyclodextrin-Dox chemotherapeutic strategy can kill tumor cells whilst protecting healthy bone. Strategies to enhance the spatio-temporal delivery of these therapeutic agents could further reduce side effects and maximize their therapeutic efficacy.
Biomaterials also show promise to circumvent the pain associated with severe bone trauma. Pain management for bone regeneration interventions typically involves non-steroidal anti-inflammatory drugs (NSAIDs), opioids, and local anesthetics.Citation75 NSAIDs can however delay bone healing and amplify the risk of other complications, whilst opioids are legally controlled compounds with major side effects and addictive properties. Local anesthetics can relieve pain, but their short duration of action limits their efficacy.Citation77 To circumvent these issues, Subaie et al. developed a bone regeneration biomaterial that could relieve pain through minimally-invasive procedures.Citation78 In their study, sodium magnesium phosphate nanocrystal gels were used as osteoinductive colloidal suspensions that were injected through high gauge needles to control the release of the local anesthetic mepivacaine, producing an injectable bone regeneration biomaterial with analgesic properties. When the system was assessed in vivo using the mouse-hindpaw model,Citation78 the gel controlled mepivacaine release for up to 24 h, prolonging its activity. No structural changes occurred for the drug, highlighting its compatibility with the gel. This biomaterial therefore holds promise for minimizing the invasiveness of bone regeneration procedures, and could limit the healing period of bone defects. Ultimately, this could eliminate the need for systemic drugs and NSAIDs during bone pain management procedures.
Growth factors
Growth factors directly act on bone progenitors via interaction with their corresponding receptors, which initiates the biochemical signaling in SCs for bone formation.Citation79 The first discovery of an osteoinductive factor dates back to the studies from Levander el al., who injected bone extracts into muscle and noted ectopic bone production.Citation80 Osteogenin bone extracts were developed and BMPs were discovered by Urist and colleagues.Citation81 BMP2 and BMP7 were clinically approved in 2000 and 2001, respectively, as growth factors with potent osteoinductive capability in cases of fractures that fail to heal (nonunion).Citation82–84 However, neither BMP2 nor BMP7 have achieved widespread clinical use primarily due to the safety concerns surrounding their use. BMP7 was withdrawn from the market in 2007. In a rat femoral bone defect in vivo, the dose control of surface-delivered BMP2 was demonstrated using PLGA and a biopolymeric nano-reservoir of BMP2.Citation85 This dose control influenced the volume of new bone and hastened the bone repair. BMP2 combined with alginate-enriched hydrogel scaffolds induced osteogenic differentiation of BMMSCs.Citation86 Currently, recombinant BMP2 (rhBMP2), using absorbable collagen sponge carrier, is available commercially as INFUSE® or InductOS® for bone repair.Citation87
PDGF promotes the recruitment and proliferation of cells, including MSCs, as well as blood vessel formation at the healing site. In synergistic combination with PLLA/Col/HA and PLLA/HA, PDGF-BB displayed enhanced osteogenic differentiation potential for bone regeneration.Citation88 rhPDGF-BB (Augment bone graft®) was approved in 2015 for hindfoot and ankle fusion in arthritis patients.Citation89 Growth differentiation factor 5 (GDF5) stimulates bone, cartilage, tendon and ligament formation.Citation90 Several preclinical investigations demonstrated the application of rhGDF5 for bone induction and soft tissue growth. BB-1 (GDF-5V453/V456), a mutant growth factor, was developed for bone reconstruction due to its superior BMP receptor-IA binding.Citation91 While both GDF5 and BB-1 displayed high angiogenic potential, BB-1 had greater bone repair capacity.Citation92 TGF-β1 participates in bone remodeling with insulin-like growth factor 1 (IGF-1).Citation3 TGF-β1 regulates osteoclastogenesis and recruits BMMSCs to the repair site, while IGF-1 induces the BMMSC differentiation into osteoblasts. It has been demonstrated that a combination of BMMSCs and IGF-1/TGF-β1 in a laminin gel scaffold improves ameliorates osteochondral defect model.Citation93
Conclusions and future perspectives
In summary, the strategies to overcome problematic bone healing are increasing. Despite these improvements, tissue engineering and regenerative medicines to improve bone repair remain in their infancy. Bone regeneration using biomaterials can combat bone fractures and defects, and the technology will continue to advance. Considering our knowledge of bone healing processes and intervention strategies, regenerative medicine approaches represent the future of bone healing. As our understanding of the biological “cues” that promote bone repair increase, the field of biomaterials will also evolve. Technical advances including 3D-printing that permits the man-made creation of both complex and novel biological structures will likely hold the future key to bone regeneration therapeutics. The advances in this area are highlighted by the ORTHOUNION,Citation94 a multicentre, open, comparative, randomized clinical trial (2015–000431-32) designed to compare the effectiveness of autologous hBMSC treatments plus biomaterials versus iliac crest autografts (ICA), to enhance bone healing in patients with femur, tibia, and humerus fractures. These trials are aimed at confirming that the biomaterial is superior to ICA regarding bone healing, and to confirm optimal doses for patient treatment. The confirmation of superiority of these biomaterials is likely to expedite the assessment of new and emerging biomaterial treatments in human disease.
Disclosure of potential conflicts of interest
No potential conflicts of interest were disclosed.
Compliance with ethical standards
Conflicts of interest
The authors declare that they have no conflict of interest.
Ethical approval
This article does not contain any studies with human participants or animals performed by any of the authors.
Acknowledgments
This work was supported by the Zhejiang Provincial Science and Technology Projects (No. LGF19H160024 to M.H.C, and 2017C37134 to C.Z), Zhejiang Provincial Natural Science Foundation of China (No. LY17H180010 to X.Y.C), Zhejiang Medical Technology Plan Project (No. WKJ-ZJ-1711 to Q.B), and Funds of Traditional Chinese Medicine Science and Technology Project of Zhejiang Province (2016ZA020 to X.Y.C).
Additional information
Funding
References
- Kohli N, Ho S, Brown SJ, Sawadkar P, Sharma V, Snow M, García-Gareta E. Bone remodelling in vitro: where are we headed?: a review on the current understanding of physiological bone remodelling and inflammation and the strategies for testing biomaterials in vitro. Bone. 2018;110:38–46. doi:10.1016/j.bone.2018.01.015.
- Astori G, Soncin S, Lo Cicero V, Siclari F, Surder D, Turchetto L, Soldati G, Moccetti T. Bone marrow derived stem cells in regenerative medicine as advanced therapy medicinal products. Am J Transl Res. 2010;2:285–95.
- Iaquinta MR, Mazzoni E, Manfrini M, D’Agostino A, Trevisiol L, Nocini R, Trombelli L, Barbanti-Brodano G, Martini F, Tognon M, et al. Innovative Biomaterials for Bone Regrowth. Int J Mol Sci. 2019;20:618. doi:10.3390/ijms20030618.
- Martin V, Bettencourt A. Bone regeneration: biomaterials as local delivery systems with improved osteoinductive properties. Mater Sci Eng C Mater Biol Appl. 2018;82:363–71. doi:10.1016/j.msec.2017.04.038.
- Przekora A. Current trends in fabrication of biomaterials for bone and cartilage regeneration: materials modifications and biophysical stimulations. Int J Mol Sci. 2019;20:435.
- Pecheux L, Forget P, Geurten C, Rausin L, Nicolescu R, Hoyoux C. [Bone disorders and complications of pediatric acute lymphoblastic leukemia: monocentric study and review of the literature]. Rev Med Liege. 2018;73:575–82.
- Winkler T, Sass FA, Duda GN, Schmidt-Bleek K. A review of biomaterials in bone defect healing, remaining shortcomings and future opportunities for bone tissue engineering: the unsolved challenge. Bone Joint Res. 2018;7:232–43. doi:10.1302/2046-3758.73.BJR-2017-0270.R1.
- Albeshri S, Alblaihess A, Niazy AA, Ramalingam S, Sundar C, Alghamdi HS. Biomarkers as independent predictors of bone regeneration around biomaterials: a systematic review of literature. J Contemp Dent Pract. 2018;19:605–18. doi:10.5005/jp-journals-10024-2306.
- Piuzzi NS, Hussain ZB, Chahla J, Cinque ME, Moatshe G, Mantripragada VP, Muschler GF, LaPrade RF. Variability in the preparation, reporting, and use of bone marrow aspirate concentrate in musculoskeletal disorders: a systematic review of the clinical orthopaedic literature. J Bone Joint Surg Am. 2018;100:517–25. doi:10.2106/JBJS.17.00451.
- Taraballi F, Bauza G, McCulloch P, Harris J, Tasciotti E. Concise review: biomimetic functionalization of biomaterials to stimulate the endogenous healing process of cartilage and bone tissue. Stem Cells Transl Med. 2017;6:2186–96. doi:10.1002/sctm.17-0181.
- Sheikh Z, Hamdan N, Ikeda Y, Grynpas M, Ganss B, Glogauer M. Natural graft tissues and synthetic biomaterials for periodontal and alveolar bone reconstructive applications: a review. Biomater Res. 2017;21:9. doi:10.1186/s40824-017-0095-5.
- Marsell R, Einhorn TA. The biology of fracture healing. Injury. 2011;42:551–55. doi:10.1016/j.injury.2011.03.031.
- Einhorn TA, Gerstenfeld LC. Fracture healing: mechanisms and interventions. Nat Rev Rheumatol. 2015;11:45–54. doi:10.1038/nrrheum.2014.164.
- Bahney CS, Zondervan RL, Allison P, Theologis A, Ashley JW, Ahn J, Miclau T, Marcucio RS, Hankenson KD. Cellular biology of fracture healing. J Orthop Res. 2019;37:35–50. doi:10.1002/jor.24170.
- Claes L, Recknagel S, Ignatius A. Fracture healing under healthy and inflammatory conditions. Nat Rev Rheumatol. 2012;8:133–43. doi:10.1038/nrrheum.2012.1.
- Bankers-Fulbright JL, Kalli KR, McKean DJ. Interleukin-1 signal transduction. Life Sci. 1996;59:61–83. doi:10.1016/0024-3205(96)00135-X.
- Raggatt LJ, Wullschleger ME, Alexander KA, Wu AC, Millard SM, Kaur S, Maugham ML, Gregory LS, Steck R, Pettit AR, et al. Fracture healing via periosteal callus formation requires macrophages for both initiation and progression of early endochondral ossification. Am J Pathol. 2014;184:3192–204. doi:10.1016/j.ajpath.2014.08.017.
- Kolar P, Schmidt-Bleek K, Schell H, Gaber T, Toben D, Schmidmaier G, Perka C, Buttgereit F, Duda GN. The early fracture hematoma and its potential role in fracture healing. Tissue Eng Part B Rev. 2010;16:427–34. doi:10.1089/ten.teb.2009.0687.
- Lu LY, Loi F, Nathan K, Lin TH, Pajarinen J, Gibon E, Nabeshima A, Cordova L, Jämsen E, Yao Z, et al. Pro-inflammatory M1 macrophages promote Osteogenesis by mesenchymal stem cells via the COX-2-prostaglandin E2 pathway. J Orthop Res. 2017;35:2378–85. doi:10.1002/jor.23553.
- Gordon S, Martinez FO. Alternative activation of macrophages: mechanism and functions. Immunity. 2010;32:593–604. doi:10.1016/j.immuni.2010.05.007.
- Lu C, Marcucio R, Miclau T. Assessing angiogenesis during fracture healing. Iowa Orthop J. 2006;26:17–26.
- Otrock ZK, Mahfouz RA, Makarem JA, Shamseddine AI. Understanding the biology of angiogenesis: review of the most important molecular mechanisms. Blood Cells Mol Dis. 2007;39:212–20. doi:10.1016/j.bcmd.2007.04.001.
- Adams JC, Lawler J. The thrombospondins. Cold Spring Harb Perspect Biol. 2011;3:a009712. doi:10.1101/cshperspect.a009712.
- Colnot C. Skeletal cell fate decisions within periosteum and bone marrow during bone regeneration. J Bone Mineral Res. 2009;24:274–82. doi:10.1359/jbmr.081003.
- Kitaori T, Ito H, Schwarz EM, Tsutsumi R, Yoshitomi H, Oishi S, Nakano M, Fujii N, Nagasawa T, Nakamura T, et al. Stromal cell-derived factor 1/CXCR4 signaling is critical for the recruitment of mesenchymal stem cells to the fracture site during skeletal repair in a mouse model. Arthritis Rheum. 2009;60:813–23. doi:10.1002/art.24330.
- Ceradini DJ, Kulkarni AR, Callaghan MJ, Tepper OM, Bastidas N, Kleinman ME, Capla JM, Galiano RD, Levine JP, Gurtner GC, et al. Progenitor cell trafficking is regulated by hypoxic gradients through HIF-1 induction of SDF-1. Nat Med. 2004;10:858–64. doi:10.1038/nm1075.
- Akiyama H, Kim JE, Nakashima K, Balmes G, Iwai N, Deng JM, Zhang Z, Martin JF, Behringer RR, Nakamura T, et al. Osteo-chondroprogenitor cells are derived from Sox9 expressing precursors. Proc Natl Acad Sci U S A. 2005;102:14665–70. doi:10.1073/pnas.0504750102.
- Bi W, Deng JM, Zhang Z, Behringer RR, de Crombrugghe B. Sox9 is required for cartilage formation. Nat Genet. 1999;22:85–89. doi:10.1038/8792.
- Eames BF, Sharpe PT, Helms JA. Hierarchy revealed in the specification of three skeletal fates by Sox9 and Runx2. Dev Biol. 2004;274:188–200. doi:10.1016/j.ydbio.2004.07.006.
- Le AX, Miclau T, Hu D, Helms JA. Molecular aspects of healing in stabilized and non-stabilized fractures. J Orthop Res. 2001;19:78–84. doi:10.1016/S0736-0266(00)00006-1.
- Burke D, Dishowitz M, Sweetwyne M, Miedel E, Hankenson KD, Kelly DJ. The role of oxygen as a regulator of stem cell fate during fracture repair in TSP2-null mice. J Orthop Res. 2013;31:1585–96. doi:10.1002/jor.22396.
- Yu YY, Lieu S, Lu C, Miclau T, Marcucio RS, Colnot C. Immunolocalization of BMPs, BMP antagonists, receptors, and effectors during fracture repair. Bone. 2010;46:841–51. doi:10.1016/j.bone.2009.11.005.
- Yang L, Tsang KY, Tang HC, Chan D, Cheah KS. Hypertrophic chondrocytes can become osteoblasts and osteocytes in endochondral bone formation. Proc Natl Acad Sci U S A. 2014;111:12097–102. doi:10.1073/pnas.1302703111.
- Teitelbaum SL. Osteoclasts: what do they do and how do they do it? Am J Pathol. 2007;170:427–35. doi:10.2353/ajpath.2007.060834.
- Matsuoka K, Park KA, Ito M, Ikeda K, Takeshita S. Osteoclast-derived complement component 3a stimulates osteoblast differentiation. J Bone Mineral Res. 2014;29:1522–30. doi:10.1002/jbmr.2187.
- Wu AC, Raggatt LJ, Alexander KA, Pettit AR. Unraveling macrophage contributions to bone repair. Bonekey Rep. 2013;2:373. doi:10.1038/bonekey.2013.107.
- Konishi T, Honda M, Nagaya M, Nagashima H, Thian ES, Aizawa M. Injectable chelate-setting hydroxyapatite cement prepared by using chitosan solution: fabrication, material properties, biocompatibility, and osteoconductivity. J Biomater Appl. 2017;31:1319–27. doi:10.1177/0885328217704060.
- Dozza B, Salamanna F, Baleani M, Giavaresi G, Parrilli A, Zani L, Lucarelli E, Martini L, Fini M, Donati DM, et al. Nonunion fracture healing: evaluation of effectiveness of demineralized bone matrix and mesenchymal stem cells in a novel sheep bone nonunion model. J Tissue Eng Regen Med. 2018;12:1972–85. doi:10.1002/term.2732.
- RMN EQ, Mohamed NN, Radwan LRS, Ibrahim FMM. Effect of bone marrow mesenchymal stem cells on healing of temporomandibular joints in rats with induced rheumatoid arthritis. Eur J Oral Sci. 2018;126:272–81. doi:10.1111/eos.12533.
- Guo J, Hu H, Gorecka J, Bai H, He H, Assi R, Isaji T, Wang T, Setia O, Lopes L, et al. Adipose-derived mesenchymal stem cells accelerate diabetic wound healing in a similar fashion as bone marrow-derived cells. Am J Physiol Cell Physiol. 2018;315:C885–C96. doi:10.1152/ajpcell.00120.2018.
- Han J, Ma B, Liu H, Wang T, Wang F, Xie C, Li M, Liu H, Ge S. Hydroxyapatite nanowires modified polylactic acid membrane plays barrier/osteoinduction dual roles and promotes bone regeneration in a rat mandible defect model. J Biomed Mater Res A. 2018;106:3099–110. doi:10.1002/jbm.a.36502.
- Amini AR, Laurencin CT, Nukavarapu SP. Bone tissue engineering: recent advances and challenges. Crit Rev Biomed Eng. 2012;40:363–408. doi:10.1615/CritRevBiomedEng.v40.i5.10.
- Kampleitner C, Obi J, Vassilev N, Epstein MM, Hoffmann O. Biological Compatibility Profile on Biomaterials for Bone Regeneration. J Vis Exp. 2018. doi:10.3791/58077.
- Kaur T, Kulanthaivel S, Thirugnanam A, Banerjee I, Pramanik K. Biological and mechanical evaluation of poly(lactic-co-glycolic acid)-based composites reinforced with 1D, 2D and 3D carbon biomaterials for bone tissue regeneration. Biomed Mater. 2017;12:025012. doi:10.1088/1748-605X/aa5f76.
- Wang W, Yeung KWK. Bone grafts and biomaterials substitutes for bone defect repair: a review. Bioact Mater. 2017;2:224–47. doi:10.1016/j.bioactmat.2017.05.007.
- Zhu DY, Lu B, Yin JH, Ke QF, Xu H, Zhang CQ, Guo Y-P, Gao Y-S. Gadolinium-doped bioglass scaffolds promote osteogenic differentiation of hBMSC via the Akt/GSK3β pathway and facilitate bone repair in vivo. Int J Nanomedicine. 2019;14:1085–100. doi:10.2147/IJN.S193576.
- Hu G, Xiao L, Fu H, Bi D, Ma H, Tong P. Study on injectable and degradable cement of calcium sulphate and calcium phosphate for bone repair. J Mater Sci Mater Med. 2010;21:627–34. doi:10.1007/s10856-009-3885-z.
- Wang J, Zhang L, Sun X, Chen X, Xie K, Lin M, Yang G, Xu S, Xia W, Gou Z, et al. Preparation and in vitro evaluation of strontium-doped calcium silicate/gypsum bioactive bone cement. Biomed Mater. 2014;9:045002. doi:10.1088/1748-6041/9/4/045002.
- Akilal N, Lemaire F, Bercu NB, Sayen S, Gangloff SC, Khelfaoui Y, Rammal H, Kerdjoudj H. Cowries derived aragonite as raw biomaterials for bone regenerative medicine. Mater Sci Eng C Mater Biol Appl. 2019;94:894–900. doi:10.1016/j.msec.2018.10.039.
- De Luca A, Vitrano I, Costa V, Raimondi L, Carina V, Bellavia D. Improvement of osteogenic differentiation of human mesenchymal stem cells on composite poly l-lactic acid/nano-hydroxyapatite scaffolds for bone defect repair. J Biosci Bioeng. 2020;129:250–57.
- Liu J, Liao J, Li Y, Yang Z, Ying Q, Xie Y, Zhou A. Bioactive tetracalcium phosphate/magnesium phosphate composite bone cement for bone repair. J Biomater Appl. 2019;34:239–49. doi:10.1177/0885328219845597.
- Hassan N, McCarville K, Morinaga K, Mengatto CM, Langfelder P, Hokugo A, Tahara Y, Colwell CS, Nishimura I. Titanium biomaterials with complex surfaces induced aberrant peripheral circadian rhythms in bone marrow mesenchymal stromal cells. PLoS One. 2017;12:e0183359. doi:10.1371/journal.pone.0183359.
- Bloise N, Petecchia L, Ceccarelli G, Fassina L, Usai C, Bertoglio F, Balli M, Vassalli M, Cusella De Angelis MG, Gavazzo P, et al. The effect of pulsed electromagnetic field exposure on osteoinduction of human mesenchymal stem cells cultured on nano-TiO2 surfaces. PLoS One. 2018;13:e0199046. doi:10.1371/journal.pone.0199046.
- Yang WF, Long L, Wang R, Chen D, Duan S, Xu FJ. Surface-Modified Hydroxyapatite Nanoparticle-Reinforced Polylactides for Three-Dimensional Printed Bone Tissue Engineering Scaffolds. J Biomed Nanotechnol. 2018;14:294–303. doi:10.1166/jbn.2018.2495.
- Ding Z, Fan Z, Huang X, Lu Q, Xu W, Kaplan DL. Silk-Hydroxyapatite Nanoscale Scaffolds with Programmable Growth Factor Delivery for Bone Repair. ACS Appl Mater Interfaces. 2016;8:24463–70. doi:10.1021/acsami.6b08180.
- Ding Z, Han H, Fan Z, Lu H, Sang Y, Yao Y, Cheng Q, Lu Q, Kaplan DL. Nanoscale Silk-Hydroxyapatite Hydrogels for Injectable Bone Biomaterials. ACS Appl Mater Interfaces. 2017;9:16913–21. doi:10.1021/acsami.7b03932.
- Grue BH, Veres SP. Use of tendon to produce decellularized sheets of mineralized collagen fibrils for bone tissue repair and regeneration. J Biomed Mater Res B Appl Biomater. 2020;108:845–56.
- Li M, Zhang C, Zhong Y, Zhao J. A Novel Approach to Utilize Icariin as Icariin-Derived ECM on Small Intestinal Submucosa Scaffold for Bone Repair. Ann Biomed Eng. 2017;45:2673–82. doi:10.1007/s10439-017-1900-y.
- Lim CT, Ren X, Afizah MH, Tarigan-Panjaitan S, Yang Z, Wu Y, Chian KS, Mikos AG, Hui JHP. Repair of osteochondral defects with rehydrated freeze-dried oligo[poly(ethylene glycol) fumarate] hydrogels seeded with bone marrow mesenchymal stem cells in a porcine model. Tissue Eng Part A. 2013;19:1852–61. doi:10.1089/ten.tea.2012.0621.
- Ho-Shui-Ling A, Bolander J, Rustom LE, Johnson AW, Luyten FP, Picart C. Bone regeneration strategies: engineered scaffolds, bioactive molecules and stem cells current stage and future perspectives. Biomaterials. 2018;180:143–62. doi:10.1016/j.biomaterials.2018.07.017.
- Cassino PC, Rosseti LS, Ayala OI, Martines MAU, Portugual LC, Oliveira CG, Silva IS, Caldas RDA. Potencial of different hydroxyapatites as biomaterials in the bone remodeling. Acta Cir Bras. 2018;33:816–23. doi:10.1590/s0102-865020180090000010.
- Kumai T, Yui N, Yatabe K, Sasaki C, Fujii R, Takenaga M, Fujiya H, Niki H, Yudoh K. <p>A novel, self-assembled artificial cartilage–hydroxyapatite conjugate for combined articular cartilage and subchondral bone repair: histopathological analysis of cartilage tissue engineering in rat knee joints. Int J Nanomedicine. 2019;14:1283–98. doi:10.2147/IJN.S193963.
- Oryan A, Alidadi S. Reconstruction of radial bone defect in rat by calcium silicate biomaterials. Life Sci. 2018;201:45–53. doi:10.1016/j.lfs.2018.03.048.
- Solana Munoz J, Kettenberger U, Procter P, Pioletti DP. Non-setting, injectable biomaterials containing particulate hydroxyapatite can increase primary stability of bone screws in cancellous bone. Clin Biomech (Bristol, Avon). 2018;59:174–80. doi:10.1016/j.clinbiomech.2018.09.023.
- Neufurth M, Wang X, Wang S, Steffen R, Ackermann M, Haep ND, Schröder HC, Müller WEG. 3D printing of hybrid biomaterials for bone tissue engineering: calcium-polyphosphate microparticles encapsulated by polycaprolactone. Acta Biomater. 2017;64:377–88. doi:10.1016/j.actbio.2017.09.031.
- Chu W, Gan Y, Zhuang Y, Wang X, Zhao J, Tang T, Dai K. Mesenchymal stem cells and porous beta-tricalcium phosphate composites prepared through stem cell screen-enrich-combine(-biomaterials) circulating system for the repair of critical size bone defects in goat tibia. Stem Cell Res Ther. 2018;9:157. doi:10.1186/s13287-018-0906-1.
- Šponer P, Filip S, Kučera T, Brtková J, Urban K, Palička V, Kočí Z, Syka M, Bezrouk A, Syková E, et al. Utilizing autologous multipotent mesenchymal stromal cells and β -tricalcium phosphate scaffold in human bone defects: a prospective, controlled feasibility trial. Biomed Res Int. 2016;2016:2076061. doi:10.1155/2016/2076061.
- Gomez-Barrena E, Rosset P, Gebhard F, Hernigou P, Baldini N, Rouard H, Sensebé L, Gonzalo-Daganzo RM, Giordano R, Padilla-Eguiluz N, et al. Feasibility and safety of treating non-unions in tibia, femur and humerus with autologous, expanded, bone marrow-derived mesenchymal stromal cells associated with biphasic calcium phosphate biomaterials in a multicentric, non-comparative trial. Biomaterials. 2019;196:100–08. doi:10.1016/j.biomaterials.2018.03.033.
- Akilal NLF, Bercu NB, Sayen S, Gangloff SC, Khelfaoui Y, Rammal H, Kerdjoudj H. Cowries derived aragonite as raw biomaterials. Mater Sci Eng C Mater Biol Appl. 2019 1;94:894–900.
- Zhu D, Su Y, Young ML, Ma J, Zheng Y, Tang L. Biological Responses and Mechanisms of Human Bone Marrow Mesenchymal Stem Cells to Zn and Mg Biomaterials. ACS Appl Mater Interfaces. 2017;9:27453–61. doi:10.1021/acsami.7b06654.
- Deluiz D, Delcroix GJR, D’Ippolito G, Grau-Monge C, Bonnin-Marquez A, Reiner T, Tinoco EMB, Amadeu T, Pires FR, Schiller PC, et al. Human Bone Marrow-Derived Mesenchymal Stromal Cell-Seeded Bone Biomaterial Directs Fast and Superior Mandibular Bone Augmentation in Rats. Sci Rep. 2019;9:11806. doi:10.1038/s41598-019-48236-8.
- Mebarki M, Coquelin L, Layrolle P, Battaglia S, Tossou M, Hernigou P, Rouard H, Chevallier N. Enhanced human bone marrow mesenchymal stromal cell adhesion on scaffolds promotes cell survival and bone formation. Acta Biomater. 2017;59:94–107. doi:10.1016/j.actbio.2017.06.018.
- Bolander J, Ji W, Leijten J, Teixeira LM, Bloemen V, Lambrechts D, Chaklader M, Luyten FP. Healing of a Large Long-Bone Defect through Serum-Free In Vitro Priming of Human Periosteum-Derived Cells. Stem Cell Rep. 2017;8:758–72. doi:10.1016/j.stemcr.2017.01.005.
- Bischoff I, Tsaryk R, Chai F, Furst R, Kirkpatrick CJ, Unger RE. In vitro evaluation of a biomaterial-based anticancer drug delivery system as an alternative to conventional post-surgery bone cancer treatment. Mater Sci Eng C Mater Biol Appl. 2018;93:115–24. doi:10.1016/j.msec.2018.07.057.
- Borgeat A, Ofner C, Saporito A, Farshad M, Aguirre J. The effect of nonsteroidal anti-inflammatory drugs on bone healing in humans: a qualitative, systematic review. J Clin Anesth. 2018;49:92–100. doi:10.1016/j.jclinane.2018.06.020.
- Khodaverdi E, Golmohammadian A, Mohajeri SA, Zohuri G, Mirzazadeh Tekie FS, Hadizadeh F. Biodegradable in situ gel-forming controlled drug delivery system based on thermosensitive poly(epsilon-caprolactone)-poly(ethylene glycol)-poly(epsilon-caprolactone) hydrogel. ISRN Pharm. 2012;2012:976879. doi:10.5402/2012/976879.
- Lim G, Lin GH, Monje A, Chan HL, Wang HL. Wound healing complications following guided bone regeneration for ridge augmentation: a systematic review and meta-analysis. Int J Oral Maxillofac Implants. 2018;33:41–50. doi:10.11607/jomi.5581.
- Ahmed E, Al Subaie ML, Tamimi I, Stone LS, Millecamps M, Barralet J, Tamimi F. Injectable bone regeneration biomaterial with analgesic properties. Front Bioeng Biotechnol Conference Abstract: 10th World Biomaterials Congress; Montreal, Canada; 2018.
- Santo VE, Gomes ME, Mano JF, Reis RL. Controlled release strategies for bone, cartilage, and osteochondral engineering–Part I: recapitulation of native tissue healing and variables for the design of delivery systems. Tissue Eng Part B Rev. 2013;19:308–26. doi:10.1089/ten.teb.2012.0138.
- Levander G, Willstaedt H. Alcohol-soluble osteogenetic substance from bone marrow. Nature. 1946;157:587. doi:10.1038/157587b0.
- Urist MR, Iwata H, Strates BS. Bone morphogenetic protein and proteinase in the guinea pig. Clin Orthop Relat Res. 1972;85:275–90. doi:10.1097/00003086-197206000-00039.
- Michon F, Forest L, Collomb E, Demongeot J, Dhouailly D. BMP2 and BMP7 play antagonistic roles in feather induction. Development. 2008;135:2797–805. doi:10.1242/dev.018341.
- Qing W, Guang-Xing C, Lin G, Liu Y. The osteogenic study of tissue engineering bone with BMP2 and BMP7 gene-modified rat adipose-derived stem cell. J Biomed Biotechnol. 2012;2012:410879. doi:10.1155/2012/410879.
- Schmidt-Bleek K, Willie BM, Schwabe P, Seemann P, Duda GN. BMPs in bone regeneration: less is more effective, a paradigm-shift. Cytokine Growth Factor Rev. 2016;27:141–48. doi:10.1016/j.cytogfr.2015.11.006.
- Bouyer M, Guillot R, Lavaud J, Plettinx C, Olivier C, Curry V, Boutonnat J, Coll J-L, Peyrin F, Josserand V, et al. Surface delivery of tunable doses of BMP-2 from an adaptable polymeric scaffold induces volumetric bone regeneration. Biomaterials. 2016;104:168–81. doi:10.1016/j.biomaterials.2016.06.001.
- Jung T, Lee JH, Park S, Kim YJ, Seo J, Shim HE, Kim K-S, Jang H-S, Chung H-M, Oh S-G, et al. Effect of BMP-2 Delivery Mode on Osteogenic Differentiation of Stem Cells. Stem Cells Int. 2017;2017:7859184. doi:10.1155/2017/7859184.
- McKay WF, Peckham SM, Badura JM. A comprehensive clinical review of recombinant human bone morphogenetic protein-2 (INFUSE Bone Graft). Int Orthop. 2007;31:729–34. doi:10.1007/s00264-007-0418-6.
- Raghavendran HR, Mohan S, Genasan K, Murali MR, Naveen SV, Talebian S, McKean R, Kamarul T. Synergistic interaction of platelet derived growth factor (PDGF) with the surface of PLLA/Col/HA and PLLA/HA scaffolds produces rapid osteogenic differentiation. Colloids Surf B Biointerfaces. 2016;139:68–78. doi:10.1016/j.colsurfb.2015.11.053.
- DiGiovanni CW, Lin SS, Daniels TR, Glazebrook M, Evangelista P, Donahue R, Beasley W, Baumhauer JF. The Importance of Sufficient Graft Material in Achieving Foot or Ankle Fusion. J Bone Joint Surg Am. 2016;98:1260–67. doi:10.2106/JBJS.15.00879.
- Jin L, Li X. Growth differentiation factor 5 regulation in bone regeneration. Curr Pharm Des. 2013;19:3364–73. doi:10.2174/1381612811319190003.
- Kleinschmidt K, Ploeger F, Nickel J, Glockenmeier J, Kunz P, Richter W. Enhanced reconstruction of long bone architecture by a growth factor mutant combining positive features of GDF-5 and BMP-2. Biomaterials. 2013;34:5926–36. doi:10.1016/j.biomaterials.2013.04.029.
- Kleinschmidt K, Wagner-Ecker M, Bartek B, Holschbach J, Richter W. Superior angiogenic potential of GDF-5 and GDF-5(V453/V456) compared with BMP-2 in a rabbit long-bone defect model. J Bone Joint Surg Am. 2014;96:1699–707. doi:10.2106/JBJS.M.01462.
- Gugjoo MB, Amarpal, Abdelbaset-Ismail A, Aithal HP, HP A, Pawde AM, Kumar GS, Sharma GT. Mesenchymal stem cells with IGF-1 and TGF- β1 in laminin gel for osteochondral defects in rabbits. Biomed Pharmacother. 2017;93:1165–74. doi:10.1016/j.biopha.2017.07.032.
- Gomez-Barrena E, Padilla-Eguiluz NG, Avendano-Sola C, Payares-Herrera C, Velasco-Iglesias A, Torres F, et al. A multicentric, open-label, randomized, comparative clinical trial of two different doses of expanded hbm-mscs plus biomaterial versus iliac crest autograft, for bone healing in nonunions after long bone fractures: study protocol. Stem Cells Int. 2018;2018:6025918. doi:10.1155/2018/6025918.