ABSTRACT
To develop a tissue-engineered vascular graft, we used pericardial effusion-derived progenitor cells (PEPCs) collected from drained fluid after open-heart surgery in children with congenital heart diseases to repopulate a decellularized porcine pulmonary artery. The PEPCs were compared with human fibroblasts (HS68) and human umbilical vein endothelial cells (HUVECs) in cell growth and migration. They were cultured with the matrices via an inner approach (intima), lateral approach (media), and outer approach (adventitia). PEPCs grew and migrated better than the other two cells 14 days after seeding in the decellularized vessel. In immunofluorescence assays, PEPCs expressed CD90 and CD105 indicating a vascular differentiation. PEPCs grew in a decellularized porcine pulmonary artery matrix may have the potential for producing tissue-engineered vascular grafts.
INTRODUCTION
Cardiovascular diseases, mainly coronary artery disease and stroke, are the leading cause of mortality and morbidity worldwide.Citation1 Although the proportion of people undergoing surgery for these causes is declining, the incidence of cardiovascular disease has increased in recent years.Citation2,Citation3 Surgical vascular grafting such as coronary artery and peripheral artery bypass grafting are the most often used strategy for long-term revascularization of occluded vessel. In clinical practice, replacement of these small diameter vessels (<6 mm) usually use autologous vascular graft with internal thoracic arteries, radial arteries, or saphenous veins.Citation4,Citation5 However, patient’s blood vessels may not available or suitable for autologous transplantation.Citation6,Citation7 The challenges of limited graft availability, especially in elderly patients, together with the increased 10-year failure rate of venous grafts urge demands for alternative strategies.Citation8,Citation9
The tissue-engineered vascular grafts (TEVG) may offer an alternative approach to overcome these challenges. TEVGs use 2 main scaffolds: synthetic material and natural matrix or a mixture of both.Citation10,Citation11 The most common synthetic vascular grafts are expanded polytetrafluoroethylene (Dacron®) and polyurethane, which have long-term patency in large vessels (>6 mm).Citation12,Citation13 However, synthetic grafts are unfavorable for cell attachment and maintenance because of the tendency of thrombosis and intimal hyperplasia from chronic inflammation in small vessels (<6 mm).Citation14 Compared with synthetic materials, natural materials may have poor mechanical properties and inflammatory responses, yet they have a better biodegradability and biocompatibility. The idea of using decellularized porcine arteries as scaffolds for TEVG is attractive.Citation10,Citation11 However, the process of decellularization may alter the native three-dimensional architecture of the extracellular matrix (ECM).Citation15 For constructing a functional vessel, the ideal host cell population should contain living cells with the potential to grow, repair and remodeling after injury.Citation16 Cell candidates for TEVG are embryonic stem cells (ESC), bone marrow-derived stem cells, tissue-specific stem cells, fibroblasts, and endothelial cells. Induced pluripotent stem cells and ESCs are accessible and theoretical ideal cells for cells repopulation in a decellularized vessel, but they have tumorigenic and ethical problems. Endothelial cells and fibroblasts are differentiated cells which limited their differentiation to different cell types of a decellularized vessel.Citation17
In previous work, we isolated and characterized pericardial effusion-derived progenitor cells (PEPCs) from open-heart surgery in children.Citation18 The PEPCs expressed markers of cardiovascular lineage and helped repair ischemic heart in mice, and their conditioned medium enhanced angiogenesis of human umbilical vein cells (HUVEC) in vitro. In this study, we evaluate the potential of PEPCs to repopulate in a decellularized porcine vessel. The growth and cell migration of PEPCs were compared with human fibroblasts and HUVECs in 3 culture methods: cell seeding via intima surface, vessel cross section, and adventitia, respectively.
RESULTS
Patient sample collection and isolation of PEPCs
PEPCs were collected from 5 patients with congenital heart disease, aged 1 week to 12 months who underwent open-heart surgery.Citation18 The diagnoses include complex heart disease, total anomaly of pulmonary venous return, aortopulmonary window, endocardial cushion defect, and tetralogy of Fallot. PEPCs were grown and expanded as described in the materials and methods. After expanded to sufficient amount for cell seeding, they were stored in liquid nitrogen until use. After thawing, all samples (irrespective of patient age, sex, or cardiac disease) formed cardiospheres after 4 days of culture, which demonstrated survival and robust growth ().
Decellularized porcine pulmonary arteries
The porcine pulmonary artery turned white after chemical decellularization (Supplementary Figure S1). H & E stain of the decellularized vessel confirmed absence of cells in the vessel (). The three-layer structures (intima, media, and adventitia) with intact extracellular matrix (ECM) before () and after () decellularization were demonstrated. This was further verified by trichrome stain which showed intact smooth muscle (red) and collagen (blue, mostly in the adventitia and under intima) before () and after () decellularization. The media layer was compact, and the intimal surface was smooth without interruption after decellularization.
Figure 2. Decellularization removed cells from the pulmonary artery and retained the extracellular matrix. A) H-E-stained section of a non-decellularized porcine pulmonary artery revealing numerous cells in the intima, media, and adventitia. B). Immunofluorescent of nuclei DAPI stain in the same tissue. C) H&E stained section of the same vessel after decellularization. D) DAPI staining of the same section revealing total removal of cell nuclei. E, F) trichrome stain of the vessel before E) and after F) decellularization. The three layers of the vessel remained grossly intact after decellularization: an intact smooth intima, a compact media with red stained smooth muscle fiber, and collagen-rich adventitia and subintimal area. bar = 100 µm.
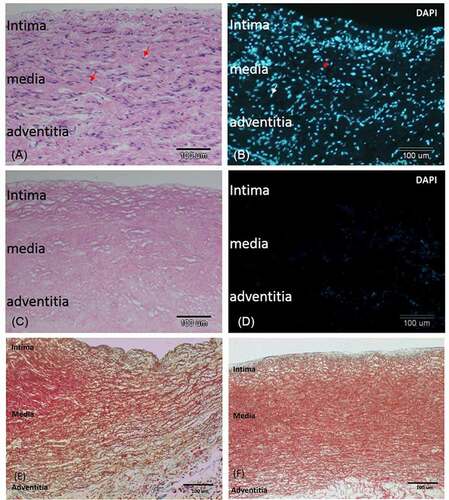
Orientation of decellularized porcine pulmonary arterial segments
To compare whether cell migrations would be affected by on different vascular surface of the vessel, the segmented vessel was cut and face upward in different orientations. demonstrates the orientations of the 3 different surfaces (intima, adventitia, cross section) for cell implantations.
Figure 3. Images of decellularized scaffold in agarose gel. images A, C, and E are vertical projections. images B, D, and F are horizontal projections. In A) and B), the intima surface was facing upward (white arrow: intima; blue arrow: adventitia), whereas in C) and D), the adventitia surface was facing upward. In E and F, the pulmonary artery was cut into a 0.5 cm inner diameter × 0.5 cm long segment and a plastic microtip was insert in the vascular cannel to allow the cross section of the vessel facing upward at the center of a 12-well plate.
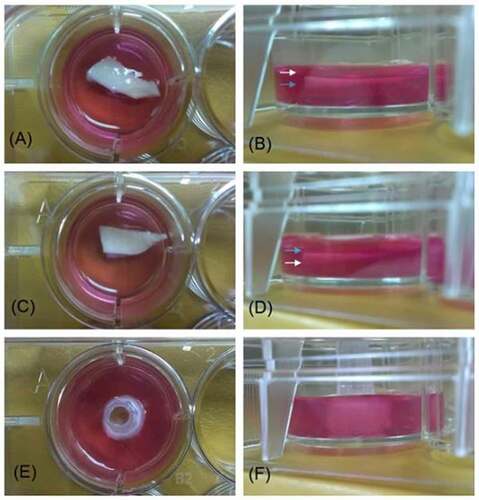
Repopulation of decellularized porcine pulmonary arterial segments with HS68 cells
HS68 cells were seeded in the decellularized porcine pulmonary artery segments via adventitia, intimal, and lateral surfaces and cultured for 7 and 14 days (). Few HS68 cells grew after seeding in the adventitia () and intimal surfaces (). Cells grew and migrated better when seeding in the lateral (cross-section) surface () at day 7 after implantation.
Figure 4. HS68 cells grew and migrated in the decellularized porcine pulmonary artery after implantation via different surfaces. Implantation via the A) adventitia, B) intima, C) lateral after 7 days, and implantation via the D) adventitia, E) intima, F) lateral after 14 days were shown with H&E and DAPI staining. arrows indicated the HS68 cells.
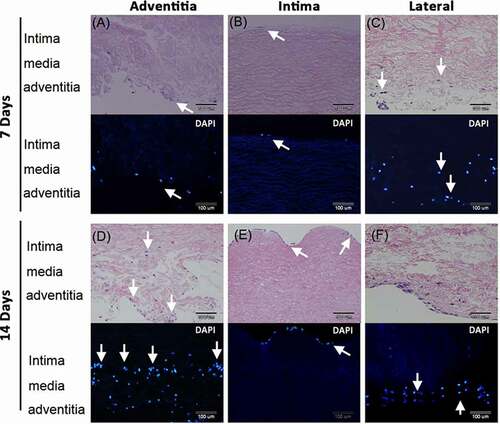
After 14 days culture, many HS68 cells grew and migrated in the vessel when seeded in the adventitia. Cells presented in the adventitia and media layers (). More cells grew on the intima compared to day 7 culture but cells were limited to this layer after seeding for 14 days on this surface (). The lateral seeding of cells showed cells grew and migrated a small distance in the medial layer ().
Repopulation of decellularized porcine pulmonary arterial segments with HUVECs
The cell growth and migration were less evident in HUVECs implanted vessels (). Few cells were noted on the adventitia surface 7 days after seeding cells on this side (). A thin layer of cells grew on the intima after seeding cells on this surface (). Few cells grew on the intima after seeding cells from lateral surfaces (). Most cells died after culture for 14 days either implanted from adventitia (), intima (), or lateral (). Some cell debris were found in sub-intimal area indicated that cells might migrate to this location but could not survive ().
Figure 5. Human umbilical vein endothelial cells (HUVEC) grew and migrated in the decellularized porcine pulmonary artery after implantation via different surfaces. Implantation via the A) adventitia, B) intima, C) lateral after 7 days, and implantation via the D) adventitia, E) intima, F) lateral after 14 days were shown with H&E and DAPI staining. arrows indicated the HUVECs.
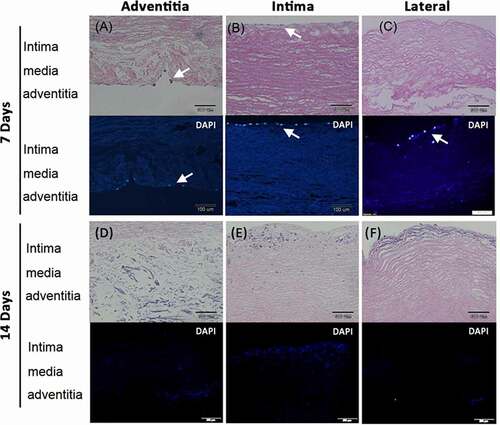
Repopulation of decellularized porcine pulmonary arterial segment with PEPCs
PEPCs were seeded in the decellularized porcine pulmonary artery segments via adventitia, intimal, and lateral surfaces and cultured for 7 and 14 days (). After 7 days culture, PEPCs seeding via the adventitia surface migrated into the intima and adventitia layer (), whereas PEPCs seeding on the intima () and lateral surfaces () grew a thin layer of cells in the intima. More cells grew after 14 days culture (). Seeding PEPCs from the adventitia surface allowed cells migration and proliferation in the vessel in 14 days.
Figure 6. Pericardial effusion-derived progenitor cells (PEPCs) grew and migrated in the decellularized porcine pulmonary artery after implantation via different surfaces. Implantation via the A) adventitia, B) intima, C) lateral after 7 days, and implantation via the D) adventitia, E) intima, F) lateral after 14 days were shown with H&E and DAPI staining. arrows indicated the PEPCs.
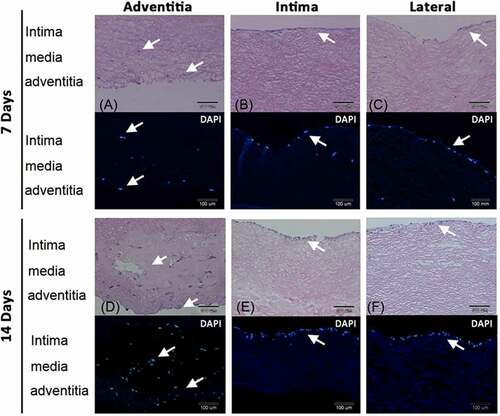
shows the pooled cell number from 2 separate experiments of HS68, HUVEC and PEPCs implanted in the adventitia, intima, and lateral in a decellularized vessel. Day-7 cultures did not show significant difference between groups. On day-14 after cell implantation, more HS68 (p < .05, Bonferroni posttests) and PEPCs (p < .01, Bonferroni posttests) grew in the decellularized vessel compared to HUVECs.
Cell differentiation
Cell differentiation markers on cells after 14 days grew in the vascular segment were examined. CD90, also known as endoglin, Thy1 (thymocyte differentiation antigen 1) is a glycophosphatidylinositol-linked glycoprotein expressed by endothelial cells, hematopoietic stem cells, and in developing nervous tissue.Citation18 CD105 is an accessory receptor for transforming growth factor beta (TGF-β) and its expression is crucial in angiogenesis.Citation18 In this experiment, HUVECs isolated from a fresh human umbilical cord vein were grown and implanted to the decellularized vessel without passage as a positive control.Citation19 depicts the expression of CD90 and CD105 in HUVECs and PEPCs in the cultured blood vessel. In line with data shown in H & E and DAPI stains, PEPCs expressed more CD90 and CD105 in the sections from cells implanted on the adventitial surface. Of note that more cells grew and migrated in the vessel after implanted with primary HUVECs isolated from a fresh umbilical vein, compared to HUVEC cell line with freeze/thaw and passages from BCRC ().
Figure 8. Pericardial effusion-derived progenitor cells (PEPCs) expressed vascular differentiation surface markers at day-14 after implantation on a decellularized porcine pulmonary artery. First row showed positive control of CD105 (green) and CD90 (red) expressions on HUVECs after implanting the cells on the intimal surface at day-14. PEPCs grew and migrated better when implanted on the adventitia surface (second row) as compared with implantation on intimal (the 3rd row) and lateral surfaces (the 4th row). All PEPCs expressed CD105 and CD90 after implantation. arrows indicated cells merged with all markers. Bar = 50 µm.
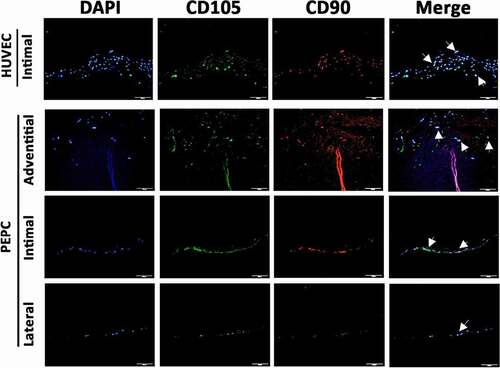
DISCUSSION
The present study demonstrated that PEPCs isolated from drain waste product of open-heart surgery have the ability to repopulate in a decellularized vessel matrix in a 2-week culture. In this model, PEPCs showed convincing cell growth and migration ability in the decellularized vessel matrix, serving as a reliable method for producing TEVGs.
An ideal TEVG with optimal mechanical strength and vascular function requires 3 basic paradigms: 1) a scaffold with well-preserved ECM; 2) incorporation with pluripotential cells that can differentiate into endothelium, muscle, nerves, and ECM; 3) coordinated signals orchestrate between cells and tissue. Different methods including chemical, enzymatic, hyper- or hypo-tonic solutions, freeze-thaw, and supercritical CO2 have been used to decellularize blood vessels.Citation10 Detergents are widely used because they are highly effective to remove cellular components of a vessel. However, all decellularized methods may result in some degree of ECMs and structural disruption, which may affect their mechanical and biological functions.Citation10 We used a simple and inexpensive SDS gradient method by exposing the vessel to 0.01%, 0.1% and 1% SDS for 24 hours, and then 1% Triton X-100 for 30 min at room temperature. With this protocol, nearly all cellular components in the vessel can be removed and ECMs can be preserved, as demonstrated in H & E and trichrome stains. Inspection with a scanning electron microscope of the ultrastructure may further confirm the results in future studies.
A blood vessel contains 3 layers: intima, media, and adventitia, which are mainly composed of endothelial cells, smooth muscle cells, and fibroblasts, respectively. Different cells have been used to repopulate a decellularized vessel to mimic these layers. With the advance of biotechnology, diverse cell sources such as somatic and stem cells have been broadly investigated. Endothelial cells (ECs) play a critical role in preventing thrombosis and pathogen invasions, and sense blood flow-induced shear force in a blood vessel. However, obtaining sufficient cells for autologous ECs seeding is difficult due to limited source and proliferative capacity of these cells. Moreover, it has been shown that more than 90% of seeded endothelial cells can be lost due to hemodynamic stress after implantation in vivo.Citation10 Fibroblasts can differentiate into myofibroblasts, which produce collagen in the tunica adventitia after stimulation with transforming growth factor-β1 (TGFβ1) in conjunction with high tensile stress. However, although Takahashi et al.Citation20 have shown that adult human fibroblasts can be induced to form pluripotent stem cells by reprogramming with 4 specific transcription factors, therefore having some stemness potential, fibroblasts cannot differentiate into smooth muscle cells or endothelial cells. One challenge in generating a functional TEVG is to distribute cells within the graft to the adequate locations. ECs and smooth muscle cells (SMCs) need to locate to the intima and tunica media space, respectively.Citation21 On the other hand, PEPCs, a type of progenitor cells, may have potential to differentiate into different cell types under appropriate stimulations and environmental niche. Previously we had shown that PEPCs can help repair ischemic heart in a mouse model.Citation18 Further studies following the present study is to investigate the potential of cell differentiations into different cell lineage by culturing PEPCs for a longer period of time and incorporate with physiological pulsatile flow in the cultural system.
An alternative to 2-D static seeding in this study is a dynamic seeding using a rotating technique. Rotational seeding utilizes centrifugal force to insert cells onto scaffolds.Citation22 Stem cell have been widely used in tissue engineering of TEVG studies.Citation23 However, stem cells has carcinogenic potential and raises ethical issue. On the other hand, PEPCs were derived from patient’s surgical waste product. It is a simple, safe and inexpensive approach compared to different stem cells models nowadays. Among 3 cell types we used, PEPCs grew and migrated the best in all tested surfaces. We expected a longer incubation of cells with the decellularized vessel in a 3D rotation culture chamber and a physiological pulsatile flow may have significant beneficial impact on the cell growth, proliferation and differentiation. Another advantage of PEPCs is no risk of rejection from patient’s own cells.
PEPCs as the cell source for TEVG may have antigenicity issue. Nevertheless, PEPCs are originated from the same patient and will be stored in a tissue bank for his/her future use. Utilization of the patient’s own cells will not lead to rejection. HLA mapping for suitable donor from the bank is also possible if the collection is large enough. On the other hand, PEPCs obtained from a newborn baby or young infants might also be an additional advantage. Agarwal et al.Citation24 found an age-dependent effect of human pediatric cardiac progenitor cells isolated from juvenile heart-failure patients in repairing right ventricular heart failure in an animal model.Citation24 Further investigations should be addressed to determine how age may affect PEPCs functions.
Interestingly, our results showed that the primary HUVECs (cultured without passage) isolated from a fresh umbilical vein migrated better than the passaged cells we bought from BCRC. Previous studies have shown that the mesenchymal stem cells can be isolated successfully from an umbilical cord.Citation25 The primary HUVECs may have some degree of stemness or progenitor cells characteristics. However, HUVECs isolated from a fresh umbilical cord and cultured immediately on a decellularized vessel without cryopreservation is not always feasible. Polchow et al. compared the fresh and cryopreserved HUVECs and concluded that cryopreservation did not influence cell properties as judged from the cellular marker expression profile.Citation19 The authors did not study cell differentiation in vivo. Despite HUVECs are the most studied cells in TEVG, the road to translating into clinical practice is still far beyond expectations.Citation26 Even with the multipotent umbilical mesenchymal stem cells, the therapeutic potential of these cells may be substantially reduced during cryopreservation (cryo stun effect).Citation26 This may explain the differences of cell growth and migration of HUVECs in our results. Cell senescence might have occurred in the former cells after several passages.
The PEPCs expressed endothelial cell markers (CD90 and CD105) after implanting into the decellularized vessel. The expression of CD90 and CD105 at the intimal region indicates that the PEPC population has endothelial characteristics that is critical for a vessel to prevent thrombosis and inflammation. The present study may provide resolutions to 2 challenges in TEVG: 1) a reliable cell source with appropriate growth characteristics, and 2) a decellularized biological vessel scaffold to test various methods and culture cells. Our approach provides a promising approach for the treatment of cardiovascular diseases, especially those with small vessel (< 6 mm diameter) disease. The idea of combination of PEPC cell therapy and tissue engineering might be extended to isolating and expanding other progenitor cells originated from surgical drainage fluids of other organs/tissues for tissue repair.
Lack of protein quantification, SEM images, laminin, elastin or fibronectin staining to verify ECM and ultrastructure preservation is a limitation of the present study. Here we only used trichrome staining to demonstrate the preservation of the ultrastructure after decellularization. Further studies should be performed to address this issue.
CONCLUSIONS
Seeding of autologous progenitor cells, collected from children’s open-heart postoperative pericardial effusion fluid, to a decellularized porcine pulmonary artery scaffold is a promising approach for producing tissue-engineered vascular grafts.
MATERIALS AND METHODS
Collection of pericardial effusion-derived progenitor cells
All human studies were conducted according to the principles of Helsinki Declaration and were approved by our Institutional Review Board (NCKU-A-ER-104-213). Informed consent was obtained from his/her parents or guardians. PEPCs were collected every 24 h for 2 days from children’s open-heart postoperative pericardial effusion fluid, as described in our previous work.Citation18 In brief, cells were collected after centrifugation at 1500 rpm for 10 min at 4°C and resuspend in 30 ml cold RPMI medium (Gibco, Invitrogen, Carlsbad, CA). Mononuclear cells were isolated by density gradient centrifugation with Ficoll-Paque (GE Healthcare, Buckinghamshire, UK) according to the manufacturer’s instruction. Immediately after harvest, these cells (at a concentration of 2 × 104 cells/μl) were cultured directly without sorting on a fibronectin-precoated 48-well plate with cardiosphere-forming medium (300 μl/well). The medium was composed of 35% IMDM and 65% DMEM/F-12 Mix (Gibco, Invitrogen, Carlsbad, CA), 3.5% FBS, 1% penicillin-streptomycin, 1% L-glutamine, 0.1 mM 2-mercaptoethanol, 1% thrombin, 2% B-27, 0.1% fibroblast growth factor-basic (bFGF), 0.01% epidermal growth factor (EGF), and 0.1% cardiotrophin-1 at final working concentrations. On the second and third day, 200 μl of the supernatant containing erythrocytes and white blood cells were replaced by fresh medium by slowly aspiration without disturbing the loosely attached cells. The procedures were repeated every 3 days to completely remove the erythrocytes and unattached cells. Cells were expanded in a ratio of 1: 2 in 5–7 days thereafter. Cells after 5–9 passage (in vitro culture for 35– 45 days) were used for subsequent studies.
Cell culture
HS68 fibroblasts were bought from Bioresource Collection and Research Center (BCRC 60,038, Hsinchu, Taiwan). The cells were cultured in a 10-ml Petri dish and incubated at 37°C under 5% CO2 atmosphere with DMEM (30–2002, American Type Culture Collection, Teddington, UK) supplemented with 100 U/mL penicillin and 100 mg/mL streptomycin (15,140,122; Gibco, Paisley, UK) and 10% fetal bovine serum. HUVECs (H-UV001) were also bought from BCRC, Taiwan. To test whether primary HUVECs might have a better growth and migration, cells were isolated from a fresh umbilical vein, which was kindly provided by Dr. Chen-Hsiang Yu, Department of Obstetrics and Gynecology of our hospital, according to methods described by Baudin et al.Citation27 The cells were cultured in M199 medium (31,100,035 Gibco, Paisley, UK) supplemented with 0.1 mg/ml heparin, 0.05 mg/ml endothelial cell growth supplement, 100 U/mL penicillin, 100 mg/mL streptomycin, and 20% (v/v) fetal bovine serum. PEPCs were cultured in the 10-cm dish coated with 0.02 ug/ml fibronectin and enriched with mesenchymal stem cell medium (Sigma, St. Louis, MO) with cardiosphere-forming medium as mentioned above.
Vessel decellularization, preparation, and recellularization
Arterial vessels were prepared from porcine arteries obtained from porcine cadavers immediately after sacrifice and stored at −80°C in normal saline. Before seeding cells for culture, a 4-cm porcine pulmonary artery was retrieved and decellularized by incubation with 0.01% sodium dodecyl sulfate (SDS), followed by 0.1% SDS and 1% SDS 24 h sequentially for each concentration and followed by incubated with 1% Triton X-100 for 30 min at room temperature. The specimen was washed 4 times with 45 ml PBS + 25% isopropanol (v/v) for 30 min, and then 45 ml PBS 4 times for 30 minutes. All solutions used for decellularization contained 1% penicillin and streptomycin (Supplementary Figure S1).
To compare the efficacy of cell proliferation and migration from different surfaces (intimal, adventitial and cross section (lateral)) (Supplementary Figure S2), the decellularized vessel was bisected into a 0.5 cm inner diameter (I.D.) × 1.5 cm long segment (for intimal or adventitial facing upward) or a 0.5 cm I.D. × 0.5 cm long conduit with the vessel cross section facing upward. 1 × 105 cells in 20 µl medium were evenly applied to the vascular surface and incubated for 10 minutes to allow for cell adhesion before adding gel-containing medium. The 1.5% agarose gel was made by dissolving the powder (Sigma, USA) in the medium. The gel was added into the well of a 12-well plate to immobilize the vessel and restrict cell migration through one designated surface of the segmented vessel. In the cross-section surface group, a micro pipette tip was cut short and inserted into the vessel lumen and stand on the gel pre-filled well. The gel was dissolved in different medium (HS68 with DMEM; HUVEC with M199; and PEPCs with mesenchymal stem cell medium). Cells grew on the decellularized vessels were counted by KCC (one of the authors) blindly 7 days and 14 days by calculating the nuclei stained with DAPI under a fluorescent microscope with a 4× objective lens. Cells in three random fields were selected for calculation.
Hematoxylin-Eosin and trichrome staining
The paraffin-embedded sections were de-paraffinized in xylene 3 times for 5 min, and rehydrated in ethanol (100%, 95%, 85%, and 75%, 5 min each), then rinsed in water for 15 min. The tissues were stained with hematoxylin (Sigma, St. Louis, MO, USA) for 3 min, washed with running water, and counterstained with eosin (Muto Pure Chemicals, Tokyo, Japan) for a few seconds. Masson Trichrome staining (HT15-1KIT, Sigma, St. Louis, MO, USA) was performed according to the manufacturer’s instruction. The tissue slide was then dehydrated in 100% ethanol for 3 min and in xylene for 5 to 10 min, sealed with mounting medium (J.T. Baker, Thermo Fisher Scientific, Waltham, MA), and observed under a light microscope.
Immunofluorescence staining
The paraffin-embedded tissue sections were de-paraffinized and rehydrated as described above. Before applying the primary antibody, the tissue section was incubated in the blocking buffer (1% BSA in PBS) for 30 minutes at room temperature to prevent nonspecific staining. For double-immunofluorescence staining, the primary antibodies were mixed and diluted in incubation buffer according to the manufacturer’s instructions and incubated overnight at 4 °C. After washing with PBS, the tissue sections were incubated with fluorescent-conjugated secondary antibody (Alexa Fluor® 488 goat anti-rabbit IgG (H + L), ab150077 for CD90 while ab150113 goat anti-mouse IgG (H + L) was applied for CD105, Abcam, Cambridge, UK) for 1 h at room temperature. Nuclei were stained with 4ʹ 6-diamidino-2-phenylindole (DAPI, Invitrogen, Carlsbad, CA) for 10 min, then washed with PBS. Primary antibodies were CD90 (ab133350, Abcam, Cambridge, UK) and CD105 (MA5-17,041, Invitrogen, Rockford, IL, USA). The slides were sealed with Fluoromount G (SouthernBiotech, Birmingham, AL, USA).
Statistics
Two-way analysis of variance (ANOVA) was performed to determine if there were any significant differences between the different test conditions using GraphPad Prism (Version 8.0, GraphPad Software, Inc., California, USA). Bonferroni multiple comparison tests was used for pairwise comparisons. At least 3 independent experiments with duplicate to triplicate were done for each different treatment. P value < .05 was considered statistically significant.
Supplemental Material
Download Zip (1.6 MB)Acknowledgments
The authors would like to acknowledge technical support from Ms. Ya-Li Hsiao. This work was supported by Ministry of Science and Technology, Taiwan MOST-103-2314-B-006-077, MOST-110-2314-B-006-048-MY2 and National Cheng Kung University Hospital NCKUH-10506007 to JNW. TWW acknowledges funding from MOST-109-2327-B-006-005, NCKUH-1106018, and the Center of Applied Nanomedicine, National Cheng Kung University from the Featured Areas Research Center Program within the framework of the Higher Education Sprout Project by the Ministry of Education (MOE) in Taiwan.
Disclosure statement
No potential conflict of interest was reported by the author(s).
Supplementary material
Supplemental data for this article can be accessed on the publisher’s website.
Additional information
Funding
References
- Deaths: HM. Leading causes for 2016 national vital statistics reports: from the centers for disease control and prevention, national center for health statistics. National Vital Statistics System. 2018;67:1–77.
- Rowe VL, Lee W, Weaver FA, Etzioni D. Patterns of treatment for peripheral arterial disease in the United States: 1996-2005. J Vasc Surg. 2009;49(4):910–17. doi:https://doi.org/10.1016/j.jvs.2008.11.054.
- Humphries MD, Brunson A, Li CS, Melnikow J, Romano PS. Amputation trends for patients with lower extremity ulcers due to diabetes and peripheral artery disease using statewide data. J Vasc Surg. 2016;64(6):1747–55 e3. doi:https://doi.org/10.1016/j.jvs.2016.06.096.
- Slovut DP, Lipsitz EC. Surgical technique and peripheral artery disease. Circulation. 2012;126(9):1127–38. doi:https://doi.org/10.1161/CIRCULATIONAHA.111.059048.
- Pashneh-Tala S, MacNeil S, Claeyssens F. The tissue-engineered vascular graft-past, present, and future. Tissue Eng Part B Rev. 2016;22(1):68–100. doi:https://doi.org/10.1089/ten.teb.2015.0100.
- Klinkert P, Post PN, Breslau PJ, Van Bockel JH. Saphenous vein versus PTFE for above-knee femoropopliteal bypass A review of the literature. Eur J Vasc Endovasc Surg. 2004;27(4):357–62. doi:https://doi.org/10.1016/j.ejvs.2003.12.027.
- Chew DK, Owens CD, Belkin M, Donaldson MC, Whittemore AD, Mannick JA, Conte MS. Bypass in the absence of ipsilateral greater saphenous vein: safety and superiority of the contralateral greater saphenous vein. J Vasc Surg. 2002;35(6):1085–92. doi:https://doi.org/10.1067/mva.2002.124628.
- Conte MS. The ideal small arterial substitute: a search for the Holy Grail? FASEB J. 1998;12(1):43–45. doi:https://doi.org/10.1096/fsb2fasebj.12.1.43.
- Gaudino M, Cellini C, Pragliola C, Trani C, Burzotta F, Schiavoni G, Nasso G, Possati G. Arterial versus venous bypass grafts in patients with in-stent restenosis. Circulation. 2005;112(9_supplement):I265–9. doi:https://doi.org/10.1161/CIRCULATIONAHA.104.512905.
- Fang S, Ellman DG, Andersen DC. Review: tissue engineering of small-diameter vascular grafts and their in vivo evaluation in large animals and humans. Cells. 2021;10(3):713. doi: https://doi.org/10.3390/cells10030713. PMID: 33807009; PMCID: PMC8005053.
- Saito J, Kaneko M, Ishikawa Y, Yokoyama U. Challenges and Possibilities of Cell-Based Tissue-Engineered Vascular Grafts. Cyborg and Bionic Systems. 2021;1–16. doi: https://doi.org/10.34133/2021/1532103.
- Conte MS. Critical appraisal of surgical revascularization for critical limb ischemia. J Vasc Surg. 2013;57(2):8S–13S. doi:https://doi.org/10.1016/j.jvs.2012.05.114.
- Mohr FW, Morice M-C, Kappetein AP, Feldman TE, Ståhle E, Colombo A, et al. Coronary artery bypass graft surgery versus percutaneous coronary intervention in patients with three-vessel disease and left main coronary disease: 5-year follow-up of the randomised, clinical SYNTAX trial. The Lancet. 2013;381(9867):629–38. doi:https://doi.org/10.1016/S0140-6736(13)60141-5.
- Haruguchi H, Teraoka S. Intimal hyperplasia and hemodynamic factors in arterial bypass and arteriovenous grafts: a review. J Artif Organs. 2003;6(4):227–35. doi:https://doi.org/10.1007/s10047-003-0232-x.
- Fu RH, Wang YC, Liu SP, Shih TR, Lin HL, Chen YM, Sung JH, Lu CH, Wei JR, Wang ZW, et al. Decellularization and recellularization technologies in tissue engineering. Cell Transplant. 2014;23(4–5):621-30. doi:https://doi.org/10.3727/096368914X678382 PMID: 24816454
- Mayer JE Jr., Shin’oka T, Shum-Tim D. Tissue engineering of cardiovascular structures. Curr Opin Cardiol. 1997;12(6):528–32. doi:https://doi.org/10.1097/00001573-199711000-00005.
- Nemeno-Guanzon JG, Lee S, Berg JR, Jo YH, Yeo JE, Nam BM, Koh YG, Lee JI. Trends in tissue engineering for blood vessels. J Biomed Biotechnol. 2012;2012:956345. doi:https://doi.org/10.1155/2012/956345 Epub 2012 Nov 8. PMID: 23251085; PMCID: PMC3518873
- Wong TW, Kan CD, Chiu WT, Fok KL, Ruan YC, Jiang X, et al. Progenitor cells derived from drain waste product of open-heart surgery in children. J Clin Med. 2019;8(7):1028. doi: https://doi.org/10.3390/jcm8071028. PMID: 31336927; PMCID: PMC6678880.
- Polchow B, Kebbel K, Schmiedeknecht G, Reichardt A, Henrich W, Hetzer R, Lueders C. Cryopreservation of human vascular umbilical cord cells under good manufacturing practice conditions for future cell banks. J Transl Med. 2012;10(1):98. doi:https://doi.org/10.1186/1479-5876-10-98.
- Takahashi K, Tanabe K, Ohnuki M, Narita M, Ichisaka T, Tomoda K, Yamanaka S. Induction of pluripotent stem cells from adult human fibroblasts by defined factors. Cell. 2007;131(5):861–72. doi:https://doi.org/10.1016/j.cell.2007.11.019 PMID: 18035408
- Song HG, Rumma RT, Ozaki CK, Edelman ER, Chen CS. Vascular tissue engineering: progress, challenges, and clinical promise. Cell Stem Cell. 2018;22(3):340–54. doi:https://doi.org/10.1016/j.stem.2018.02.009.
- Godbey WT, Hindy SB, Sherman ME, Atala A. A novel use of centrifugal force for cell seeding into porous scaffolds. Biomaterials. 2004;25(14):2799–805. doi:https://doi.org/10.1016/j.biomaterials.2003.09.056.
- Rana D, Zreiqat H, Benkirane-Jessel N, Ramakrishna S, Ramalingam M. Development of decellularized scaffolds for stem cell-driven tissue engineering. J Tissue Eng Regen Med. 2017;11(4):942–65. doi:https://doi.org/10.1002/term.2061.
- Agarwal U, Smith AW, French KM, Boopathy AV, George A, Trac D, Brown ME, Shen M, Jiang R, Fernandez JD, et al. Age-dependent effect of pediatric cardiac progenitor cells after juvenile heart failure. Stem Cells Transl Med. 2016;5(7):883–92. doi:https://doi.org/10.5966/sctm.2015-0241 Epub 2016 May 5. PMID: 27151913; PMCID: PMC4922847
- Lu LL, Liu YJ, Yang SG, Zhao QJ, Wang X, Gong W, Han ZB, Xu ZS, Lu YX, Liu D, et al. Isolation and characterization of human umbilical cord mesenchymal stem cells with hematopoiesis-supportive function and other potentials. Haematologica. 2006;91(8):1017–26. Epub 2006 Jul 25. PMID: 16870554.
- Arutyunyan I, Fatkhudinov T, Sukhikh G. Umbilical cord tissue cryopreservation: a short review. Stem Cell Res Ther. 2018;9(1):236. doi:https://doi.org/10.1186/s13287-018-0992-0.
- Baudin B, Bruneel A, Bosselut N, Vaubourdolle M. A protocol for isolation and culture of human umbilical vein endothelial cells. Nat Protoc. 2007;2(3):481–85. doi:https://doi.org/10.1038/nprot.2007.54.