Abstract
Type I collagen is composed of 2 polypeptides, α1(I) and α2(I), which fold into triple helix. Collagen α1(I) and α2(I) mRNAs have a conserved stem-loop structure in their 5’ UTRs, the 5’SL. LARP6 binds the 5’SL to regulate type I collagen expression. We show that 5 nucleotides within the single stranded regions of 5’SL contribute to the high affinity of LARP6 binding. Mutation of individual nucleotides abolishes the binding in gel mobility shift assay. LARP6 binding to 5’SL of collagen α2(I) mRNA is more stable than the binding to 5’SL of α1(I) mRNA, although the equilibrium binding constants are similar. The more stable binding to α2(I) mRNA may favor synthesis of the heterotrimeric type I collagen. LARP6 needs 2 domains to contact 5’SL, the La domain and the RRM. T133 in the La domain is critical for folding of the protein, while loop 3 in the RRM is critical for binding 5’SL. Loop 3 is also involved in the interaction of LARP6 and protein translocation channel SEC61. This interaction is essential for type I collagen synthesis, because LARP6 mutant which binds 5’SL but which does not interact with SEC61, suppresses collagen synthesis in a dominant negative manner. We postulate that LARP6 directly targets collagen mRNAs to the SEC61 translocons to facilitate coordinated translation of the 2 collagen mRNAs. The unique sequences of LARP6 identified in this work may have evolved to enable its role in type I collagen biosynthesis.
Introduction
Type I collagen is a complex protein composed of 2 α1(I) and one α2(I) polypeptides that fold into a triple helix. All human tissues synthesize α1-α2-α1 heterotrimer, of type I collagen, only in the skin homotrimers of α1(I) chains accumulate up to 10%.Citation1 A mechanism that regulates the exclusive synthesis of collagen heterotrimers is not well understood. Registration and disulfide bonding of α1(I) and α2(I) polypeptides at their C-terminal ends initiate folding of the triple helix.Citation2,3 Collagen polypeptides are posttranslationally modified by hydroxylations of prolines and lysines and glycosylations of hydroxyl-lysines.Citation4,5 The folding and co-translational modifications are in dynamic equilibrium, suggesting coupling of translation and folding.Citation6,7 Some evidence suggests that folding of collagen polypeptides initiates while the chains are still associated with polysomes,Citation8 implicating that the folding is determined by coordination of translation of collagen mRNAs.
Type I collagen appeared in evolution with radiation of vertebrates. Type I collagen mRNAs of all vertebrates acquired a secondary structure in their 5’ untranslated region (5’UTR) that consists of 2 stems flanking a central bulge.Citation9 This structure has been termed the collagen 5’ stem-loop (5’SL) and it is found only in collagen α1(I), α2(I) and α1(III) mRNAs, the latter encodes for type III collagen. 5’ SL binds protein LARP6 with high affinity and specificity.Citation10,11 LARP6 is a member of a superfamily of RNA binding proteins, the La-domain ribonucleoproteins (LARPs), which have 2 domains in common, the La-domain and the RNA recognition motif (RRM). The La domain is well conserved between the family members, while the RRM is highly diverged.Citation12
By binding the 5’SL, LARP6 regulates translation and stability of collagen mRNAs. Cells in which LARP6 has been knocked down produce greatly reduced amounts of type I collagen.Citation11,13 LARP6 tethers RNA helicase A (RHA) to collagen mRNAs to facilitate translation initiation. Without recruiting RHA collagen mRNAs are poorly loaded onto the polysomes.Citation14 LARP6 also interacts with nonmuscle myosin and associates collagen mRNAs with the nonmuscle myosin filaments. When nonmuscle myosin filaments are disrupted, folding of collagen heterotrimer is impaired and the cells secrete only a small amounts of α1(I) homotrimers.Citation10 Interaction of LARP6 with vimentin intermediate filaments increases the half life of collagen mRNAs.Citation15 Thus, by interacting with accessory translation factors and with cytoskeletal filaments, LARP6 stimulates synthesis of type I collagen.
Fibrosis is a major medical problem characterized by excessive production of type I collagen in various organs.Citation16-Citation19 Knock in mice in which the 5’SL of collagen α1(I) gene had been disrupted are resistant to development of hepatic fibrosis and collagen producing cells from these animals synthesize only 20-30% of type I collagen.Citation13 This clearly indicates the importance of LARP6 binding to 5’SL for development of fibrosis. There is no cure for fibrosis, therefore, elucidation of the LARP6 dependent mechanism of collagen regulation is critical, not only for development of antifibrotic drugs, but also for understanding the fundamental process of synthesis of the most abundant protein in human body, type I collagen. Here, we have characterized the binding of LARP6 to 5’SL, which has a profound importance for our understanding of type I collagen synthesis.
Results
Specificity of binding of LARP6 to 5’SL RNA
To study the LARP6-5’SL interaction in vitro we first established if bacterially expressed LARP6 faithfully reproduces the binding of LARP6 expressed in mammalian cells. To this goal we compared the binding specificities of full size LARP6 expressed in mammalian cells, purified full size LARP6 expressed by baculovirus and LARP6 containing only the La domain and RRM (LR-LARP6) expressed in E. coli. The specificity of binding was assessed by using various mutants of 5’SL (). Our previous work indicated that the La domain and RRM are sufficient for sequence specific binding and that LARP6 binds the central bulge of the 5’ SL.Citation11 Therefore, we mutated each nucleotide in the B1 and B2 parts of the bulge (), individually and tested the mutants for binding LARP6 by gel mobility shift experiments. compares the sequence of the 5’SL of human collagen α1(I) and α2(I) mRNAs and for this experiment the mutations were made in α1(I) 5’SL. The U labeled with asterisk (*U) had been mutated in our previous work and its substitution into an A abolished the binding.Citation11 compares the specificity of binding of purified LARP6 expressed from a baculovirus (left panel), of LARP6 expressed in mammalian cell extract (middle panel) and of bacterially expressed and purified LR-LARP6 (right panel). Regardless of the source of the protein, the specificity of binding to the 5’SL mutants was similar. Two nucleotides in the B1 portion of the bulge and 3 nucleotides in the B2 portion of the bulge were critical for binding (circled in ). Mutations of the B1A, B1*U (this mutation has been described in Citation11), B2C, B2A or B2G nucleotides abolished the binding of full size LARP6, expressed either by baculovirus or in whole cell extract. The LR-LARP6 expressed in E. coli interacted with the same specificity as the full size protein. So we concluded that bacterially expressed LR-LARP6 can faithfully reproduce the binding of full size protein and that posttranslational modifications of LARP6 are not necessary for binding. The purity of the purified proteins is shown in .
Figure 1. Binding of LARP6 to 5’SL RNA by gel mobility shift assay. (A) Sequence of 5’SL of human collagen α1(I) mRNA (left panel) and α2(I) mRNA (right panel). The nucleotides in α1(I) 5’SL RNA critical for binding LARP6 are circled and their mutations are indicated. The start codon is boxed. B1 and B2, single stranded regions of the bulge. The results for *U were presented in.Citation11 (B) Binding of LARP6 to α1(I) 5’SL RNA. Full size LARP6 purified after expression by baculovirus (left panel), mammalian cell extract expressing full size LARP6 (middle panel) and LR-LARP6 purified from E. coli (right panel) were used in gel mobility shift with WT and mutant 5’ SL RNA probes. The mutations in the probe are indicated at the top (black letters indicate mutations that affect binding, gray letters indicate neutral mutations). Migration of free probe and LARP6/RNA complexes is indicated. * represents a nonspecific complex in cell extracts. (C) Purity of 3 preparations of LR-LARP6 after single round of purification from E. coli and one full size LARP6 purified from baculovirus. The purifications were visualized by fast blue staining.
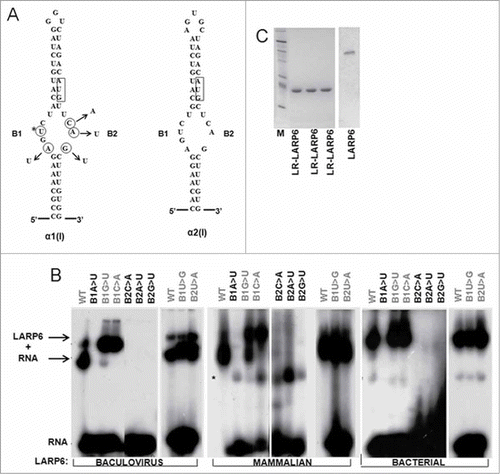
Fluorescence polarization as method to study binding of LARP6 to 5’SL
To quantitatively assess the formation of 5’SL/LR-LARP6 complexes we employed fluorescent polarization measurements (FP). FP has been used before to characterize RNA binding of several RNA binding proteins.Citation20-Citation23 FP readings depend on the size of fluorophore, the larger the complex containing the fluorophore, the higher is the FP. When 5’SL RNA was labeled at the 5’ end with fluorecein (fl-5’SL), the FP of 1 nM solution of the free RNA was ∼170 mP units. When LR-LARP6 was added to the fl-5’SL probe, the FP increased in the concentration dependent manner reaching the maximal FP of ∼320 mP units. In the figures shown this manuscript the FP of fl-5’SL RNA alone was subtracted to show only the protein dependent FP. shows a typical saturation curve obtained when 1 nM fl-5’SL RNA was titrated with increasing concentrations of LR-LARP6 (titration by protein). An apparent Kd for LR-LARP6 binding was estimated to be 0.45 nM, as the protein concentration which gave 50% saturation.
Figure 2. Binding of LR-LARP6 measured by FP. (A) Titration by protein. 1 nM fl-5’SL RNA was mixed with increasing concentrations of LR-LARP6 and FP was measured. The FP of fl-5’SL RNA alone was subtracted from the FP of fl-5’SL RNA/LR-LARP6 and plotted as function of LR-LARP6 concentration. Error bars represent +- 1 SEM from 3 measurements. The apparent Kd was estimated as 50% saturation. (B) The reactions from A were loaded on gel mobility shift gel and visualized by phosphoimaging of fl-5’SL RNA. (C) Titration by RNA. 4 nM of LR-LARP6 was mixed with increasing concentrations of fl-5’SL RNA and FP was read as in B. FP of fl-5’SL RNA alone for each concentration was subtracted from the FP of fl-5’SL RNA/LR-LARP6 complex and plotted as function of RNA concentration. (D) Reactions from C were resolved on gel mobility shift gel.
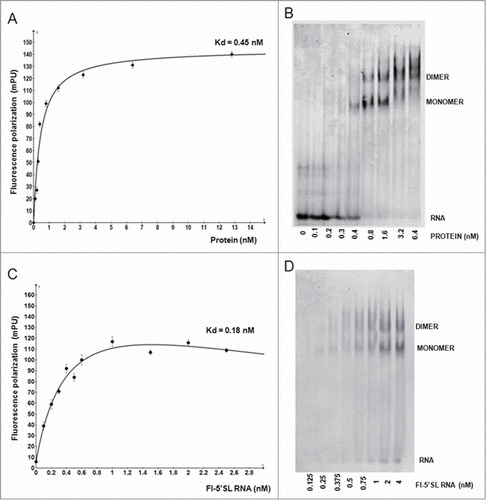
To verify that the obtained increase in FP is due to formation of RNA/protein complexes the same reactions were resolved by a gel mobility shift assay and visualized by detecting the 5’SL RNA fluorescence by phosphoimager (). Concentration dependent formation of LR-LARP6/fl-5’SL RNA complexes was detected in gel shift assays, verifying that the increase in FP reflected the formation of LR-LARP6/5’SL RNA complex. Two complexes were resolved in gel shifts, the formation of slower migrating complex was favored at higher LARP6 concentrations, suggesting that it represents a dimer of LR-LARP6 bound to fl-5’SL RNA. Because FP can not distinguish between the formation of monomers and dimers, the apparent Kd obtained from the FP readings averages the affinities of formation of both complexes. Nevertheless, it represents a relative estimate of the affinity of LARP6 binding.
To verify this result we reversed the titration and titrated 4 nM LR-LARP6 with increasing concentrations of fl-5’SL RNA (titration by RNA, ). The 50% saturation was achieved at 0.18 nM fl-5’SL RNA and approximately full saturation at 1 nM fl-5’SL RNA. The apparent Kd of 0.18 nM obtained by titration by RNA was about 2-fold lower than the apparent Kd obtained by the titration by protein (), which is in a reasonable agreement. Because titration by RNA selects for active LR-LARP6 molecules, it allows better comparison of the relative affinities obtained with different LR-LARP6 preparations and we are showing these titrations throughout the manuscript.
When titration by RNA reactions were resolved by gel mobility shift assay 2 complexes were again resolved (). Both complexes were present even at the lowest fl-5’SL RNA concentrations, suggesting that their formation is independent on fl-5’SL RNA concentration and that they represent monomers and dimers of LARP6 bound to a single fl-5’SL RNA.
Greater stability of LARP6 complexes formed on collagen α2(I) 5’ SL
Collagen α2(I) mRNA has a similar 5’SL as collagen α1(I) mRNA, with the bulge being identical (). Since the differences in the stems may affect the binding to the bulge, we compared the equilibrium binding of LR-LARP6 to α1(I) 5’SL RNA and α2(I) 5’SL RNA. shows the binding when LR-LARP6 was titrated by α1(I) and α2(I) fl-5’SL RNA. Similar saturation curves were obtained with the apparent Kd in this experiment of 0.82 nM for α1(I) fl-5’SL and 1.1 nM for α2(I) fl-5’SL. Thus, the equilibrium binding of LR-LARP6 to the 5’SL of collagen α1(I) and α2(I) mRNAs appears similar.
Figure 3. Binding of LARP6 to 5’SL of collagen α1(I) and α2(I) mRNA. (A) FP binding assay. 4 nM LR-LARP6 was titrated with increasing concentrations of α1(I) fl-5’SL RNA (circles) or with increasing concentrations of α2(I) fl-5’SL RNA (squares). (B) Competition of LR-LARP6/α(I) 5’SL RNA complex. Saturating amount of LR-LARP6 was added to radiolabeled α1(I) 5’SL RNA and complex was allowed to form for 10 minutes (lane 0). The complex was then competed with increasing molecular excess of unlabaled α1(I) 5’SL RNA (COMPETITOR α1 5’SL) or α2(I) 5’ SL RNA (COMPETITOR α2 5’ SL). After 30 minutes of incubation the samples were analyzed by gel mobility shift and autoradiography. (C) The same experiment as in B, except the LR-LARP6 complex was pre-formed on α2(I) 5’ SL RNA probe.
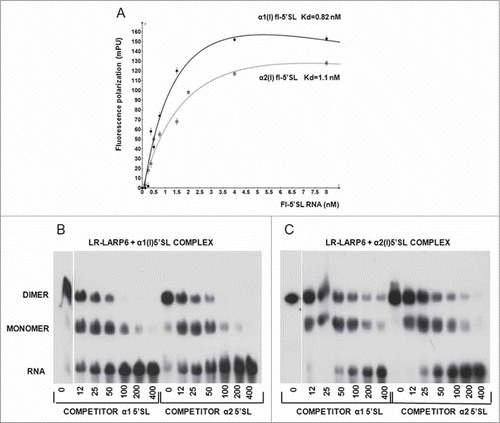
The rate of association of LARP6 with 5’SL is very fast and the complexes form instantaneously. To provide insight into their stability we used 2 approaches; titration of the preformed complexes with increasing amounts of competitor RNA after a fixed time period () and titration of preformed complexes with fixed amount of competitor RNA at different time points (). First, we formed the 5’SL/LR-LARP6 complexes by added saturating amounts of LR-LARP6 to the radiolabeled α1(I) or α2(I) 5’SL RNA and then competed the pre-formed complexes with increasing amounts of unlabeled α1(I) or α2(I) 5’SL RNA. After 30 minutes of incubation with increasing amounts of competitor RNA, the remaining complexes were analyzed by gel mobility shift and autoradiography. shows the decay of the complex pre-formed on α1(I) 5’SL. In the absence of competitor this complex was stable, with only tracing amounts of free RNA appearing after 30 minutes. With increasing concentrations of either α1(I) 5’SL or α2(I) 5’SL competitor the complex representing LARP6 dimer gradually decreased and the monomer complex and the free probe appeared. At higher molar excesses of the competitors, the monomer complex was gradually titrated out and at 400-fold excess it completely disappeared and only the free probe remained. This indicated that one LR-LARP6 molecule is dissociated first, while the removal of the remaining LR-LARP6 molecule from the 5’SL RNA required higher concentrations of the competitors. shows the same experiment for the pre-formed complex on α2(I) 5’SL RNA. Dissociation of this complex required higher molar excess of the competitors than the complex on α1(I) 5’SL RNA. A small amount of monomers and dimers was still present even at the 400-fold excess of the competitors. This indicates that complexes formed on α2(I) 5’SL are more resistant to dissociation than the α1(I) 5’SL/LARP6 complexes.
Figure 4. Temporal dissociation of LR-LARP6 complexes formed on α1(I) and α2(I) 5’SL RNAs. (A) Temporal decay of LR-LARP6/α1(I) 5’SL complex analyzed by gel mobility shift. LR-LARP6 was pre-bound to radio-labeled α1(I) 5’SL RNA for 10 min on ice. 200-fold molar excess of unlabeled α1(I) 5’SL RNA (left panel) or α2(I) 5’ SL RNA (right panel) was added, incubated for the indicated time periods and all reactions were loaded at the same time on the gel and visualized by autoradiography. (B) The same experiment with LR-LARP6 pre-bound to radio-labeled α2(I) 5’SL RNA C. Temporal decay of LR-LARP6/α1(I) 5’SL complex measured by FP. Saturating amounts of LR-LARP6 were added to fl-5’SL RNA for 10 minutes and 200-fold molar excess of unlabeled α1(I) 5’SL RNA (circles) or unlabeled α2(I) 5’SL RNA (squares) or no competitor (triangles) was then added. 2 minutes after addition of the competitors FP was read every 1 minute for 45 minutes and FP readings were plotted against time (FP of fl-5’SL RNA alone was not subtracted from the FP of fl-5’SL RNA/LR-LARP6 in this experiment). Blank; FP of fl-5’SL RNA alone. (D) Temporal decay of LR-LARP6/α2(I) 5’SL complex measured by FP. The same experiment as in C, except the complex on α2(I) fl-5’SL RNA was pre-formed and competed out.
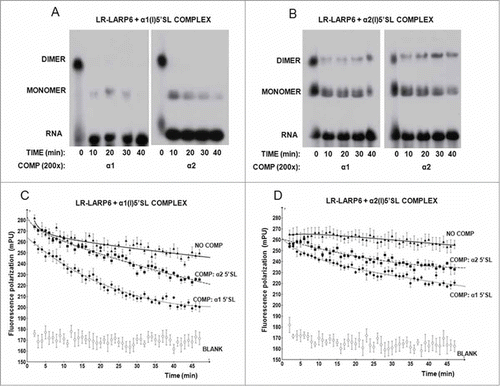
Second, we measured the temporal decay of the complexes using gel mobility shift assay and FP (). For the gel mobility shift assay, the LR-LARP6 was bound to radio-labeled α1(I) or α2(I) 5’SL RNA probes and the complexes were challenged by 200-fold molar excess of unlabeled α1(I) or α2(I) 5’SL RNA for different time periods on ice. shows temporal decay of the complex formed of α1(I) probe. After 10 min of competition time the dimer complex completely disappeared and small amount of monomers remained. After 40 min only tracing amounts of monomers could be detected. A similar pattern was seen when the LR-LARP6/α1(I)5’SL complex was challenged with α1(I) 5’SL competitor (left panel) or α2(I) 5’SL competitor (right panel). However, when the complex was pre-formed on α2(I) probe, its dissociation was much more resistant to competition with either α1(I) 5’SL competitor (, left panel) or α2(I) 5’SL competitor (right panel). About 20% of dimers and 40% of monomers still persisted after 40 min of the competition time.
For FP measurements the complexes were pre-formed on α1(I) fl-5’SL RNA or on α2(I) fl-5’SL RNA and 200-fold molar excess of unlabeled α1(I) 5’SL RNA or α2(I) 5’SL RNA competitors was added. Two minutes after the addition of competitors the FP measurements were taken every minute for 45 minutes and plotted vs time. shows the temporal decay of the α1(I) complex. Without competitor there was a small but discernible dissociation of this complex. However, in the presence of both, α1(I) 5’SL and α2(I) 5’SL competitors, the dissociation of the complex was accelerated. The initial slopes of the FP decrease were similar for both competitors, suggesting a similar rate of competition. When the same experiment was repeated with α2(I) 5’SL complex, its temporal decay was slower than that of α1(I) 5’SL complex (). The slopes of the FP decrease for both competitors were 3.3 fold lower compared to the slopes obtained with the α1(I) 5’SL complex, suggesting a greater resistance of LR-LARP6/α2(I)5’SL complex to dissociation. FP of the fl-5’SL RNAs without LR-LARP6 bound is shown as blank in .
Thus, in 2 independent assays a slower dissociation rate of the complex formed on 5’SL of collagen α2(I) mRNA was observed. Therefore, we concluded that the LARP6 binding to collagen α2(I) mRNA has a lower off rate and that it is more stable than the binding to collagen α1(I) mRNA.
Amino acids in the La module of LARP6 critical for binding 5’SL
Combination of a La-domain followed by an RRM, termed the La module, is present in all LARP family members.Citation12,24 shows comparison of amino acid sequence of the La module of human LARP6 and LARP3 (the La antigen). LARP3 binds the poly-U ends of RNA Polymerase III transcripts.Citation25,26 Crystal structure of the La module of LARP3 bound to the UGCUGUUUU duplex RNA has been solved and critical amino acids in the La domain involved in the binding have been identified.Citation27 These amino acids are also conserved in the La-domain of LARP6 (Q99, F102, Y103, F114, F135, ). Therefore, we first assessed if these conserved amino acids of the La domain are also necessary for binding of LARP6 to 5’SL. We individually mutated each of these nucleotides into alanines, expressed the mutants in mammalian cells and assessed the binding to 5’SL by gel mobility shift assays. Only one of the mutations, F135A, significantly reduced the binding (, lane 2). The other La mutants bound the 5’SL similar like the WT LARP6 (not shown). To assess if the F135A mutation did not alter the folding of LARP6 we introduced this mutations in LR-LARP6, purified the protein and compared its thermal denaturation curve to that of WT LR-LARP6 (supplemental Fig. 1, supplemental table 1). The melting temperature (Tm) of F135A mutant was similar to that of WT LARP6, suggesting that F135A mutation did not significantly affect the folding of the protein. These results indicated that the interaction between LARP6 and 5’SL RNA involves only one amino acid necessary for binding of LARP3 to poly-U.
Figure 5. Binding of LARP6 mutants to 5’SL. (A) Sequence comparison of La and RRM domains of LARP6 and LARP3. La domain is marked by full box and RRM by stippled box. Amino acids changed by single point mutations into alanines are underlined and indicated by numbers above the sequence. T133 is indicated by large letter. Loop 1 and loop 3 in the RRM are underlined. Asterisks indicate identities and dots indicate similarities between LARP6 and LARP3. (B) Binding of LARP6 mutants expressed in whole cell extract. WT HA-tagged LARP6 (lane 1) or the indicated mutants of HA-LARP6 (lanes 2-4) were expressed in HEK293 cells and cell extracts were used in gel mobility shift experiment. Lane 5 is extract of nontransfected cells (CON). Migration of 2 RNA/protein complexes (C1 and C2) is indicated. Bottom panel: expression of the transfected proteins measured by protein gel blot. (C) Binding of T133 mutants. LARP6 (lane 2), T133A (lane 3) and T133D (lane 4) were analyzed as in B. (D) Comparison of gel electrophoretic mobility of 5’SL/LARP6 complexes formed using 2 different concentrations of recombinant full size LARP6 purified from E. coli (REC I and II) and 2 concentrations of full size LARP6 expressed in whole cell extract (CEL I and II). Migration of monomers and dimmers of LARP6 and C1 and C2 complexes is indicated.
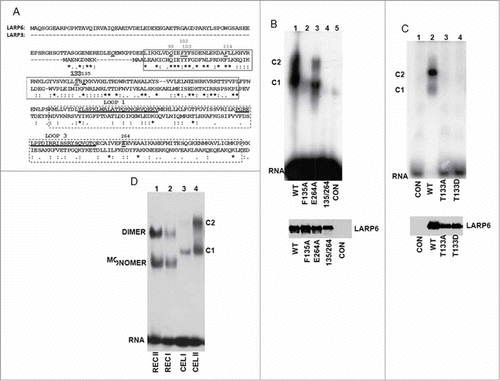
To identify other amino acids of the La domain that contribute to the binding specificity of LARP6 we performed extensive site directed mutagenesis of the La domain. Most mutations were silent, but when we mutated T133 into an A the binding was abolished (, lane 3). T133 is not conserved in LARP3 () or in the La domains of other LARPs; it represents a unique feature of LARP6. Therefore, it was important to distinguish if the lack of binding of the T133A mutant was due to disruption of a critical RNA-protein bond or to a loss of proper protein folding. The thermal denaturation profile of the LR-T133A mutant was completely different from that of WT LR-LARP6 and no transition from the folded into the unfolded state could be observed (supplemental figure 2, supplemental table 1). This suggested that the protein with T133A mutation is not folded and that the lack of its binding to 5’SL can be attributed to the grossly altered structure. Therefore, we concluded that T133 in LARP6 is a critical amino acid for maintaining the protein fold.
Our preliminary results (in preparation) and the results of others Citation28 indicate that T133 is phosphorylated. To test what a phospho-mimetic mutation, T133D, has on binding we expressed the T133D mutant and analyzed its binding to 5’SL (, lane 4). The lack of binding of T133D suggested that this mutant behaves similarly to T133A and that alterations of T133 inactivate the protein.
We also made several single point mutations throughout the RRM, but found only one (E264A), which decreased the binding. Although this mutation eliminated a negative charge, no major effect on protein folding was observed (supplemental figure 3, supplemental table 1). The combination of the E264A and F135A mutation (F135A/E264A double mutant) resulted in loss of binding to 5’SL (, lane 4), verifying the participation of both domains in high affinity binding.
During the course of these experiments we observed that 2 complexes (C1 and C2, ) were formed when LARP6 was expressed in mammalian cells and the whole cell extract was used in gel shift experiments. These complexes may be different than monomers and dimers of LARP6, seen when purified LR-LARP6 was used, and result from association of LARP6 with other proteins in the cell extract. To assess if C1 and C2 complexes contain additional proteins we compared the gel mobility of C1 and C2 complexes to that of pure recombinant full size LARP6 (). Recombinant full size LARP6 formed monomers and dimers (lanes 1 and 2), but the electrophoretic mobility of C1 and C2 complexes was slower than that of monomers and dimers of full size LARP6, suggesting presence of other proteins in both complexes. Thus, when overexpressed in whole cell extract, LARP6 monomers and dimers are associated with additional proteins. Identification of one of the components is presented in .
Figure 6. Effects of loop 3 mutations on binding. (A) Mutations of the loop 3 of the RRM of LARP6. Serine 251 is indicated by number and binding of the mutants to 5’SL is summarized to the right. (B) Binding of the HH mutant. HA-LARP6 (lane 2) and HH mutant (lane 3) were expressed in HEK293 cells and cell extract was used in gel mobility shift experiment. Lane 4 is extract of nontransfected cells (CON) and lane 1 is probe alone. Migration of 2 complexes (C1 and C2) is indicated. Bottom panel: expression of transfected proteins measured by western blot. (C) Binding of loop 3 mutants of HA-LARP6. Experiment as in B, except the mutants indicated were used. Bottom panel: expression of transfected proteins measured by protein gel blot. (D) Binding of SIM LR-ARP6 and RAN LR-LARP6. SIM and RAN mutations were introduced into LR-LARP6, proteins purified from (E) coli and binding analyzed by gel mobility shift. P; 5’SL RNA probe alone, WT; binding of 2 samples containing WT LR-LARP6, SIM; binding of 2 samples of SIM LR-LARP6, RAN; binding of 2 samples of RAN LR-LARP6. Asterisks indicate nonspecific bands. Protein preparations are shown in the bottom panel.
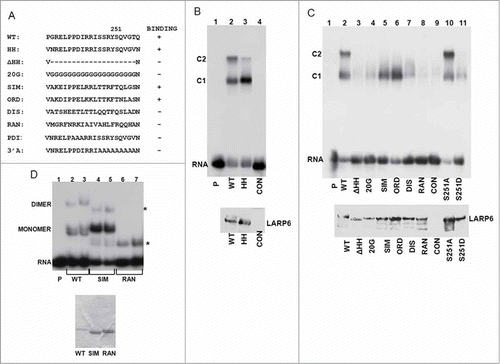
Figure 7. Identification of SEC61 in complex with LARP6 and 5’SL. (A) Control supershift assay. WT HA-LARP6 was expressed in HEK293 cells and gel shift performed with cell extract without antibody (lane 2) or with anti-nucleolin antibody added (lane 3). Lane 1 is probe alone. Migration of C1 and C2 complexes is indicated. (B) SEC61α in complex with LARP6 and 5’SL. WT HA-LARP6 was expressed in HEK293 cells and gel shift performed with cell extracts without antibody (lane 2), with anti-HA antibody added (lane 3) or with anti-SEC61α antibody added (lane 4). Lane 1 is probe alone. (C) SEC61β in complex with LARP6 and 5’SL. Experiment as in B, except anti-SEC61β antibody was added (lane 4). (D) SIM mutant does not complex with SEC61. HA-tagged SIM mutant was transfected into HEK293 cells. Gel mobility shift was done with cell extracts without antibody (lane 2), with anti-nucleolin antibody added (lane 3), with anti-HA antibody added (lane 4) or with anti-SEC61β antibody added (lane 5). Lane 1 is probe alone and lane 6 is extract of nontransfected cells. (E) SEC61β in complex with LARP6 and 5’SL in extracts of human lung fibroblasts (HLFs). WT HA-LARP6 was expressed in HLFs and gel mobility shift experiment done with extract without antibody (lane 2), with anti-nucleolin antibody added (lane 3), with anti-HA antibody added (lane 4) or with anti-SEC61β antibody added (lane 5). Lane 1 is probe alone. Arrow indicates retardation of complex caused by anti-SEC61β antibody. (F) Incorporation of V5-tagged SEC61α into complex with LARP6 and 5’SL. V5-tagged SEC61α was coexpressed with HA-tagged LARP6 and cell extracts were used in gel mobility shift without antibody (lane 2), with anti-nucleolin antibody added (lane 3), with anti-HA antibody added (lane 4) or with anti-V5 antibody added (lane 5). Supershift with anti-V5 antibody is indicated by arrow. Lane 1 is probe alone and lane 6 is extract of nontransfected cells. (G) Immunoprecipitation of transfected HA-LARP6 with SEC61 in microsomal extract. HLFs were transfected with HA-tagged LARP6 and microsomal extract prepared. The amount of HA-LARP6, SEC61β and collagen α1(I) polypeptide (COL1A1) in 5% of the input extract (I, lane 1), the amount pulled down with beads without antibody (B, lane 2) and the amount pulled down with anti-SEC61β antibody (IP, lane 3) was analyzed by western blot. (H) Co-fractionation of endogenous LARP6 with ER membranes. HLFs were fractionated by sequential detergent extraction into cytosolic fraction (SOL) and membrane fraction (MEM). The fractions were analyzed for LARP6, calnexin (CAL) and actin (ACT) by protein gel blot.
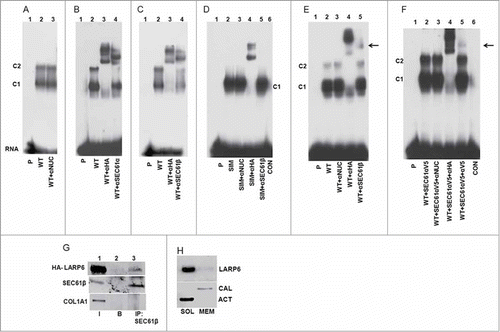
Function of loop 3 of the RRM of LARP6
Evolutionary tree of LARP proteins has been constructed and the analysis identified unique structural features of the RRM of vertebrate LARP6 proteins.Citation12 The most distinct feature of the RRM of vertebrate LARP6 is acquisition of 2 extended loops; loop 1 which connects strands 1 and 2 and loop 3 which connects strands 3 and 4 of the putative β-sheet of the RRM (these loops are underlined in ). These loops are 22 amino acids long in vertebrate LARP6, but only 4 amino acids long in invertebrate LARP6. Deletion of loop 1 had no effect on LARP6 binding to 5’SL (not shown), however, deletion of loop 3 had profound effects. To delete loop 3 from LARP6 we introduced 2 HpaI sites flanking the 5’ and 3’ ends of the loop 3 sequence by site directed mutagenesis. This changed 2 amino acids at the N-terminal end and 2 amino acids at the C-terminal end of loop 3 (HH mutant, ), but allowed us to delete 20 amino acids of the loop 3 or to change blocks of amino acids within the loop 3. The introduction of 2 HpaI sites and the resulting 4 amino acid change (HH mutant) did not affect the binding. When expressed in HEK293 cells the HH mutant strongly bound 5’SL, only the intensity of the slower migrating complex (C2) was reduced and the intensity of faster migrating complex (C1) was increased (, compare lane 2 and lane 3). Next, we introduced a series of mutations of the loop 3 () and tested the binding in cell extracts (). Deletion of the entire loop 3 (ΔHH mutant) abolished the binding (, lane 3). To exclude that shortening of the loop was responsible for the lack of binding we substituted all 20 amino acids with glycines (20G mutant). This mutant also failed to bind 5’SL RNA (lane 4). When amino acids throughout the loop 3 were substituted by amino acids with similar properties (SIM mutant), the formation of complex C1 was not affected, but the complex C2 was not formed (, lane 5). The same result was obtained with another conservative mutant, ORD (lane 6). This suggested that loop 3 must contain amino acids with certain properties. Thus, when loop 3 was substituted by amino acids with opposite properties (DIS mutant) the binding was greatly reduced (lane 7) and when random sequence was substituted (RAN mutant) the binding was abolished (lane 8). To verify that the RAN mutant was properly folded, we made the RAN mutation in LR-LARP6 and analyzed thermal denaturation of the protein (supplemental figure 1). Its denaturation curve was slightly flattened compared to the WT LR-LARP6, but the transition temperature between folded and unfolded form was similar to WT LR-LARP6 (supplemental table 1). This suggested that the RAN mutant had similar fold as WT LR-LARP6. Therefore, we concluded that loop 3 participates in binding 5’SL and that it must contain amino acid sequence with certain physical/chemical properties.
Another interesting finding from these experiments was that loop 3 is also critical for formation of the complex C2. We were able to create 2 mutants (SIM and ORD), which uncoupled the binding to 5’SL and the formation of the C2 complex. C2 complex likely contains dimers of LARP6 associated with additional proteins (). Thus, SIM and ORD mutations may have lost the ability to dimerize and/or to attract the other proteins into the complex. To assess if SIM mutant can dimerize we prepared SIM LR-LARP6 and RAN LR-LARP6 and compared their binding to that of WT LR-LARP6 (). WT LR-LARP6 formed monomer and dimer complexes with 5’SL (lanes 2 and 3), as before, while SIM LR-LARP6 preferentially formed the monomers. RAN LR-LARP6 failed to bind 5’SL, although its thermal denaturation curve (supplemental Figure 1) suggested that the protein is properly folded. Thus, the failure of SIM mutant to form the C2 complex in cell extracts may be due in to its inability to form dimers, as well as to the inability to interact with additional proteins.
The results indicated that binding 5’SL and sequestering other proteins in higher order complexes, including formation of LARP6 dimers, are 2 distinct functions of the loop 3.
Phospho-mimic mutation of serine 251 in loop 3
There are 3 serines in the loop 3, so we wanted to test if phospho-mimic mutations of these serines change the binding to 5’SL. Changing S247 or S248 into an aspartic acid did not affect the binding (not shown). Changing of S251 into an alanine also did not change the binding (, lane 10), but when this serine was mutated into an aspartic acid, binding of LARP6 was dramatically reduced (, lane 11). Only weak formation of complex C1 was seen and complex C2 was absent. Proper folding of the S251A and S251D mutants was verified by the thermal denaturation analysis (supplemental Fig. 3, supplemental Table 1). Thus, a phospho-mimic mutation of S251 had a similar effect as the mutations of entire loop 3 (DIS and 20G mutations). This suggested that a negative charge at the position 251 is highly detrimental to the function of loop 3 and that phosphorylation of S251 may regulate the loop 3 mediated functions of LARP6. The role of S251 phosphorylation in collagen biosynthesis and the kinase responsible is currently under investigation.
SEC61 as a component of LARP6/5’SL complexes
The two complexes, C1 and C2, formed when LARP6 was expressed in the whole cell extract, contain additional proteins and not simply represent monomers and dimers of LARP6. To investigate which proteins may be present in these complexes we performed gel mobility shift experiments after addition of various antibodies to the extract. A supershift induced by an antibody would indicate presence of the protein in complex with LARP6 and 5’SL RNA. To verify this approach we overexpressed HA-LARP6 in HEK293 cells and added anti-nucleolin antibody (negative control) and anti-HA antibody (positive control) to the extract. Anti-nucleolin antibody did not change the migration of the C1 and C2 complexes (, compare lanes 2 and 3), but anti-HA antibody supershifted both complexes, indicating that they contain HA-LARP6 (, lane 3). Then, we tested numerous other antibodies. When antibody against Sec61α, the α subunit of SEC61 translocon of the ER membrane,Citation29-Citation31 was added, a supershift of the complex C2 was induced and the intensity of complex C1 was reduced (lane 4). This was surprising, but suggested that SEC61 translocon (or its α subunit) associated with LARP6 and 5’SL RNA in the complex C2 and, perhaps, in the complex C1. We used non-denaturing detergent in making cell extracts, so SEC61 was liberated from the ER membrane and was available to interact with LARP6 and 5’SL RNA. Because SEC61 is a trimer of α, β and γ subunits Citation29-Citation31 we repeated the supershift experiments using an antibody against SEC61β subunit (). SEC61β antibody caused a supershift of the C2 complex and reduction of the complex C1 (lane 4), similarly to the SEC61α antibody. This strongly argued that the complete SEC61 translocon associated with LARP6 and 5’SL RNA in both complexes; C1 and C2.
The SIM mutant of LARP6 formed only complex C1 (, lane 5), therefore, we used this mutant to test if the C1 complex of SIM mutant contains SEC61. When supershift experiments were done using the SIM mutant, anti-HA antibody supershifted the single complex formed (, lane 4), but anti-SEC61β antibody had no effect (lane 5). Therefore, we concluded that the SIM mutant, not only had lost the ability to form dimers, but also had lost the ability to interact with the SEC61 translocon. This also suggested that SEC61 is complexed with 5’SL RNA by interaction with WT LARP6 and not by directly binding the 5’SL RNA.
To provide further evidence that SEC61 can complex with LARP6 and 5’SL RNA we employed a different cell type, human lung fibroblasts (HLFs). We overexpressed HA-tagged LARP6 in HLFs and performed supershift experiments. shows that anti-SEC61β antibody caused retardation of a complex (lane 5, arrow), suggesting the formation of 5’SL/LARP6/SEC61 complex in this cell type, as well. Next, we overexpressed SEC61α subunit tagged at the C-terminus with a V5 tag (SEC61α-V5) in HLFs to analyze if the tagged protein will be incorporated into the complex with LARP6/5’SL RNA. When SEC61α-V5 was co-expressed with HA-tagged LARP6, complexes C1 and C2 were formed and they were both supershifted with anti-HA antibody, as before (, lane 4). Addition of anti-V5 antibody induced a weak supershift (lane 5, arrow), suggesting that a small amount of SEC61α-V5 was associated into the complex with LARP6 and 5’SL RNA. Because complete SEC61 translocon appears to associates with LARP6 and 5’SL, the transfected SEC61α-V5 subunit must compete with the endogenous SEC61α for limiting amounts of SEC61β and Sec61γ to form the chimeric translocons. Thus, a weak supershift is consistent with the notion that complete SEC61 translocon incorporates into the complexes with LARP6 and 5’SL RNA.
To provide independent evidence that LARP6 can interact with SEC61 translocon we expressed HA-tagged LARP6 in HLFs, prepared microsomes from these cells and used the micosomal extract in co-immunoprecipitation experiments (). HA-LARP6 was found in the microsomal extract, as was SEC61β (lane 1). As additional control, we also analyzed collagen α1(I) polypeptide, which is normally present in the ER lumen, and it was also present in the microsomal extract (lane 1). When SEC61β was immunoprecipitated, it pulled down HA-LARP6, while collagen α1(I) polypeptide (lane 3) and control immunoprecipitation (lane 2) did not. This suggested that LARP6 and SEC61 can interact and is consistent with the results of supershift experiments. A relatively small fraction of HA-LARP6 was pulled down compared to the fraction of SEC61β that was pulled down, suggesting that HA-LARP6 had not interacted with all translocons.
The small amounts of endogenous LARP6 found associated with internal membranes precluded immunoprecipitation experiments to show the interaction between the endogenous LARP6 and SEC61 (see ). Endogenous LARP6 is predominantly cytosolic protein in HLF,Citation11 but if it interacts with SEC61, we expected to find a small amount associated with the ER membrane. Without purifying microsomes, we fractionated extracts of HLFs into cytosolic and membrane fractions using sequential detergent extraction Citation32 and analyzed endogenous LARP6 by western blot. shows that the majority of LARP6 was cytosolic, but a small amount was extracted with the membrane fraction. Analysis of the ER marker, calnexin,Citation33 verified that the membrane fraction contained ER proteins and analysis of the cytosolic marker, actin, confirmed that there was no cross-contamination of the fractions. A small steady state amount of endogenous LARP6 found in the membrane fraction suggested that the protein does not accumulate at the ER membrane, but may only transiently associate.
We concluded from the above experiments that the loop 3 of LARP6 RRM has 2 functions, one is to participate in binding 5’SL and the other is to tether the collagen mRNAs to other proteins, including SEC61 translocation channel.
SIM mutant acts as dominant negative protein in collagen synthesis
The discovery that SEC61 translocon can associate with LARP6 and 5’SL RNA in a higher order complex was surprising. Because we could demonstrate this interaction only when LARP6 was overexpressed, it was important to assess if this interaction has a functional significance for biosynthesis of type I collagen. To this goal, we made 2 additional loop 3 mutants. In one mutant we substituted 3 amino acids at the N-terminal side of the loop 3 (PDI mutant, ) and in the other mutant we substituted 9 amino acids at the C-terminal side (3’A mutant, ). All proteins in these experiments were overexpressed using adenoviruses to achieve >90% transduction efficiency of HLFs, necessary to study the dominant negative effects on collagen synthesis. shows the binding of these new mutants to 5’SL when expressed in HLFs. As before, WT LARP6 formed 2 complexes, C1 and C2, SIM mutant formed only the complex C1, while RAN mutant failed to form any complex (, lanes 2-4). PDI mutant failed to bind 5’SL (lane 5) and 3’A mutant showed minimal formation of complex C1 and no complex C2 (lane 6). Based on the location of PDI and 3’A mutations we concluded that, both, the N-terminal part and the C-terminal part of loop 3 participate in binding 5’SL. Then, we assessed the effect of the mutants on expression of collagen α1(I) and α2(I) polypeptides (). Overexpression of WT LARP6 had no effect on secretion of collagen polypeptides into the cell medium (, lane 1). Overexpression of 2 LARP6 mutants which cannot bind 5’SL (PDI, RAN) or one mutant which has greatly reduced binding (3’A) also had no effect on collagen secretion (lanes 3-5). However, overexpression of SIM mutant, which can bind 5’SL but can not interact with SEC61 or form dimers, reduced the production of both collagen polypeptides (lane 2), acting as a dominant negative protein for translation or secretion of collagen polypeptides.
Figure 8. SIM mutant as dominant negative protein for type I collagen expression. (A) Binding of PDI and 3’A mutants of LARP6. WT HA-LARP6 and the mutants indicated were expressed in HLFs and cell extracts were used in gel mobility shift experiments. P, probe alone, CON, extract of nontransfected cells. Expression of the transfected proteins is shown in B. (B) SIM mutant acts as dominant negative for collagen synthesis. Analysis of the cellular medium. Collagen α1(I) (COL1A1) and α2(I) (COL1A2) polypeptides were analyzed in the cellular medium of cells in A by western blot. Loading control, fibronectin (FIB). LARP6, expression of the transfected proteins in cellular extract. (C) Intracellular level of collagen polypeptides. Expression of collagen α1(I) (COL1A1) and α2(I) (COL1A2) polypeptides in the cellular extract after overexpression of WT HA-LARP6 and the indicated mutants, analyzed by protein gel blot. Loading control: actin (ACT). LARP6, expression of the transfected proteins. (D) Total RNA was extracted from cells in C and expression of collagen α1(I) (COL1A1) and α2(I) (COL1A2) mRNAs analyzed by RT-PCR. Loading control: GAPDH mRNA.
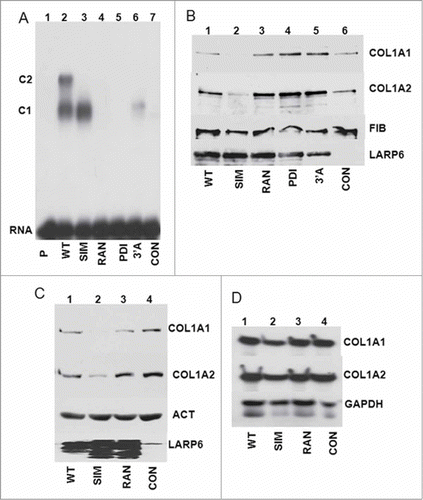
We also analyzed the effect of SIM mutant on cellular level of collagen polypeptides and on expression of collagen mRNAs. SIM mutant also reduced the cellular level of collagen polypeptides (), without affecting the level of collagen mRNAs (), supporting the notion that uncoupling 5’SL binding from the interaction with SEC61 affects translation of collagen polypeptides. Thus, the ability of LARP6 to simultaneously interact with 5’SL of collagen mRNAs and with SEC61 translocon may be an important function in biosynthesis of type I collagen.
Discussion
In the last several years a compelling evidence has emerged that the expression of type I collagen is regulated by binding of LARP6 to the 5’SL of collagen mRNAs.Citation10,11,13-15,34-40 This work revealed that 5’SL/LARP6 interaction is critical for high level of collagen expression, thus, understanding the LARP6 dependent mechanism of collagen synthesis is important for understanding reparative and reactive fibrosis. LARP6 tethers stimulatory factors, like RHA, STRAP or FKBP3 to collagen mRNAs to increase their translational competitiveness.Citation14,35,40 In addition, LARP6 associates collagen mRNAs with vimentin filaments to increase their half life.Citation15,34 Both events contribute to the increased synthesis. Thus, it was important to characterize the interaction of LARP6 with 5’SL in detail. In this report we describe that: 1. Five nucleotides within the single stranded region of 5’SL RNA are critical for interaction with LARP6. Some of these nucleotides are necessary for maintaining the tertiary structure of 5’SL and some may be directly contacted LARP6, but we could not distinguish between these functions. 2. Two domains of LARP6 are needed for interaction with 5’ SL RNA, the La domain and the RRM. T133 in the La domain is a critical for maintaining proper folding of LARP6, while F135 contributes to binding. 3. The loop 3 within the RRM is a unique feature of the vertebrate LARP6 and is neccessary for binding 5’SL. Loop 3 also participates in formation of a complex consisting of LARP6, 5’SL and protein translocation channel SEC61. 4. LARP6 mutant which can bind 5’SL, but which cannot interact with SEC61, acts as a dominant negative protein for collagen synthesis. This indicates that coupling of 5’SL binding and interaction with SEC61 is an important step in collagen biosynthesis. 5. The complex of LARP6 and collagen α2(I) 5’SL is more resistant to dissociation than the complex of LARP6 and collagen α1(I) 5’SL. The more stable binding of LARP6 to collagen α2(I) mRNA may be a determinant for preferential synthesis of heterotrimers of type I collagen over that of homotrimers of α1(I) chains.Citation1
LARP6 binds 5’SL of type I collagen mRNAs with Kd in the low nM range. Such tight binding has been described for several RNA binding proteins Citation41-Citation44 and in the case of histone mRNA stem-loop binding protein the binding involves only 1-2 direct contacts between the RNA and the protein.Citation45,46 The five nucleotides of 5’SL critical for binding LARP6 reside in the single stranded regions. From our work it is not possible to determine how many nucleotides make contacts with LARP6 and how many are involved in formation of the RNA tertiary structure recognized by LARP6. The stringent conditions of gel mobility shift assay detect only stable interactions, thus, the nucleotides identified () support the stable binding of LARP6 to collagen mRNAs. The stable interaction is needed if premature translation of collagen mRNA is to be prevented and for coordination of their translation on the membrane of the ER.Citation40,47 Collagen mRNAs are present at 5,000-30,000 copies per cell Citation48 or at 20-40 fg/cell,Citation49 which corresponds to low nM concentrations. Therefore, the apparent Kd of LARP6 binding estimated in low nM range is needed for LARP6 dependent collagen regulation in the cellular environment.
The only crystal structure of a LARP protein solved is that of the La module of LARP3 bound to the dsRNA containing 3 uridines at the 3’ end.Citation27 The crystal structure identified 5 amino acids within the La domain that make direct contacts with the poly-U terminus. These amino acids are conserved in LARP6 (), but only one of these amino acids is important for binding 5’SL (F135). Mutations of the 4 other conserved amino acids had no effect, suggesting a completely different recognition of the 5’SL by LARP6 than poly-U by LARP3. While it is possible that F135 of LARP6 contacts the U nucleotide of the 5’SL, the interactions of LARP6 with the other nucleotides must involve LARP6 specific sequences. We identified T133 as an amino acid critical for proper folding of LARP6; mutation of T133A resulted in completely unfolded protein (supplemental Figure 2). A threonine at the similar position is not found in the La domain of other LARP family members, however, it is conserved in LARP6 of all vertebrates, suggesting an indispensable role. The T133A mutant or the phospho-mimic T133D mutant were unable to bind 5’SL (). It has been reported that T133 is phosphorylated Citation28 and our preliminary proteomics analysis confirmed this finding. This raises a possibility that the structure and function of LARP6 may be modulated by phosphorylation of T133. The kinase involved and the functional significance of T133 phosphorylation are currently under investigation.
Another domain of LARP6 indispensable for binding 5’SL is the RRM. The unique features of RRM of vertebrate LARP6 are 2 extended loops, loop 1 and loop 3, that connect the strands of the β-sheet of this domain (). These loops are 22 amino acids long, compared to 4 amino acids in the invertebrate LARP6.Citation12 Deletion of loop 1 had no effect on LARP6 binding to 5’SL, however, mutations of loop 3 were highly detrimental. When loop 3 was substituted with glycines (20G mutant) or with random amino acids (RAN mutant) the binding to 5’SL was greatly reduced. The RAN mutant appeared to be folded similarly to WT LARP6 (supplementary figure 1), suggesting that loop 3 participates in specific RNA/protein contacts, rather than in maintaining the protein folding. Two smaller mutations of loop 3, one at the N-terminus (PDI) and the other at the C-terminus (3’A), also dramatically reduced the binding, suggesting the involvement of most amino acids within the loop 3. However, one amino acid, S251, has a potential to have a regulatory role. While S251A mutation was silent, the phospho-mimic mutation S251D reduced the binding to 5’SL (). Thus, in addition to T133 phosphorylation, which may change the folding of LARP6, phosphorylation of S251 may regulate the recognition of the 5’SL. Although the S251 phosphorylation has yet to be demonstrated in vivo, our results indicate that the specific amino acids of LARP6 have evolved to support its role in expression of vertebrate type I collagen.
During the course of binding experiments with purified LR-LARP6 we observed formation of 2 complexes. These complexes clearly represented monomers and dimers of LARP6 bound to single 5’SL RNA, because their formation was dependent on the LR-LARP6 concentration () and independent of the 5’SL concentration (). Recombinant full size LARP6 also forms monomers and dimers (). However, when full size LARP6 was overexpressed in cellular extracts, the 2 complexes seen (C1 and C2), had slower electrophoretic motbility than the monomers and dimers of recombinant LARP6, suggesting recruitment of other cellular proteins into the complex with LARP6 and 5’SL RNA. However, we have never seen the evidence of dimers formation in cell extracts without overexpressing LARP6.Citation11,14,40 This suggests that the concentration of endogenous LARP6 is too low in cell extracts to allow detectable formation of the dimmers, but we can not exclude their existence under native conditions.
The surprising discovery was that protein translocation channel SEC61 (translocon SEC61,Citation29-Citation31) was found as one of the components. In our experiments SEC61 was solubilized from the ER membrane using a detergent. Both antibodies, against SEC61α and against SEC61β, caused a supershift of the LARP6/5’SL RNA complex (), suggesting that the fully assembled translocon was present.Citation50 When SEC61α was tagged, the tagged subunit was also incorporated into the complex with LARP6 and 5’SL RNA (). Immunoprecipitation experiments using microsomes verified that LARP6 and SEC61 can interact (), although the amount of LARP6 pulled down was small. This indicates that either the interaction is weak or that only a subset of SEC61 complexes binds LARP6. A small amount of endogenous LARP6 co-fractionated with the ER membranes (), suggesting that an interaction of LARP6 with membrane proteins, including Sec61, is possible in vivo. Because of the small amount of LARP6 on the membrane, we could not directly demonstrate the interaction of the endogenous LARP6 and SEC61. But, based on our findings we speculate that LARP6 may directly deliver collagen mRNAs to the SEC61 translocons. The existence of a specialized subset of SEC61 translocons dedicated to translation of collagen polypeptides has been suggested before.Citation51 After initiation of translation the collagen mRNAs are retained at the ER membrane by interactions of ribosomes with SEC61 translocons Citation52,53 and nascent collagen polypeptides are translocated into the ER lumen by the signal peptide dependent mechanism.
Biological relevance of the interaction between LARP6 and SEC61 is underscored by the dominant negative effect of the SIM mutant. When selected amino acids of loop 3 were replaced with amino acids with similar properties (SIM mutant), the protein bound 5’SL, but could not form complex with SEC61. The SIM mutant also could not form dimers, either overexpressed in cell extracts () or expressed as SIM LR-LARP6 (). The SIM mutant suppressed collagen synthesis in dominant negative manner, while WT LARP6 or the mutants which cannot bind 5’SL, were without effect (). We believe that this effect was predominantly due to the inability to interact with the SEC61, rather than to inability to form dimers, as dimer formation has never been observed with endogenous LARP6. Thus, uncoupling the binding to collagen mRNAs from the interaction with SEC61 is detrimental to collagen synthesis and strongly supports the notion that direct targeting of collagen mRNAs to the SEC61 translocons is a critical step in collagen biosynthesis.
The equilibrium binding constants for binding of LR-LARP6 to 5’SL of collagen α1(I) mRNA and α2(I) mRNA were similar (). However, when LR-LARP6 was bound to α2(I) 5’SL, the complex resisted higher concentrations of competitor RNAs () and for prolonged periods of time () than the complex pre-formed on α1(I) 5’SL, suggesting that it is less prone to dissociation. Type I collagen is always secreted as heterotrimer of 2 α1(I) and one α2(I) polypeptides,Citation4,5 although homotrimers of α1(I) polypeptides readily form if α2(I) polypeptide is not available for folding. This was observed in mice in which collagen α2(I) gene had been inactivated Citation54,55 and in rare human mutations that inactivated the expression of α2(I) gene.Citation56 The more stable binding of LARP6 to collagen α2(I) mRNA may preferentially recruit collagen α2(I) mRNA into the translational pathway with α1(I) mRNA and may contribute to the exclusive synthesis of heterotrimeric type I collagen.
In conclusion, by studying binding of LARP6 to 5’SL RNA several aspects relevant to biosynthesis of type I collagen have been revealed. The high affinity binding of LARP6 may be needed for recognition of collagen mRNAs in the cellular environment, where they are present at nM concentrations. The more stable binding of LARP6 to collagen α2(I) mRNA is suggested as a mechanism which drives synthesis of heterotrimeric type I collagen. Association of SEC61 translocon with the 5’SL/LARP6 complex is critical for collagen synthesis and suggests that collagen mRNAs are directly delivered to the protein translocation channel to coordinate their translation. Amino acid motifs specific for LARP6 have evolved to support these functions of the protein. Further elaboration on the results presented here will greatly advance our understanding of the biosynthesis of type I collagen.
Material and Methods
Constructs
Human LARP6 cDNA was cloned into pcDNA3 vector with HA-tag at the N-terminus. Site directed mutagenesis of the single amino acids was done by QuickChange mutagenesis kit (Stratagene, 200523-5), according to the manufacturer's instructions. Deletion of the 20 amino acids in loop 3 was done by introducing 2 HpaI restriction sites at nt 600-606 and nt 660-666 (nt #1 is the A of the start codon). Then, HpaI-HpaI fragment was cut out and plasmid were religated (ΔHpa construct). Substitutions of the 20 amino acids of loop 3 were done by ligating double stranded oligonucleotides with the desired mutations into the ΔHpa plasmid. The identity of all mutations was verified by sequencing.
Constructs for expression of LARP6 having only La and RRM domains (LR-LARP6) in E. coli were made by PCR amplification of the pcDNA3 constructs with 5’ primer starting at amino acid 65 and containing a NdeI site and 3’ primer starting at amino acid 301 and containing a XbaI site and cloning of the PCR products into NdeI and XhoI sites of pET15b vector. Construct expressing full size LARP6 in E. coli was made by PCR amplification of full size LARP6 cDNA and cloning into pET15b vector.
Templates for in vitro transcription of mutant probes were made by ligating double-stranded oligonucleotides with the desired sequence into EcoRI-HindIII sites of pGEM3 vector (Promega). In vitro transcription was done after linearization of the templates with HindIII.
Adenoviruses were constructed by re-cloning of the LARP6 mutants from pcDNA3 vectors into pAd-CMV-Track vector, following by recombination in BJ5 E. coli cells, as described.Citation57
Purification of recombinant LARP6
pET15b plasmids were transformed into Roseta E. coli strain and the cells grown in terrific broth to OD260 of 0.8, after which the expression was induced by 1 mM IPTG and growth continued for 5 h at 25°C. His-tag LR-LARP6 was purified by Ni-column as described. The purified protein was dialyzed against PBS or against 200 mM NaCl, 0.5% NP-40 and its purity was estimated by SDS-PAGE and staining with Fast-blue. Typically, the purity was >90% after the single step purification. Baculovirus expressing full size LARP6 was constructed and purified according to manufacturer procedure (Life Technologies, 10359-016).Citation58
Cells and transfections
HEK293 cells were grown under normal conditions. Transfections were done in 6-well plates with 1 μg of plasmid using TransIT-293 transfection reagent (Mirus, MIR2700). The cells were harvested 48 h after the transfections. Human lung fibroblasts (HLFs) Citation59 were fractionated into cytosolic and membrane fractions by sequential detergent extraction, as described.Citation32 Transduction of HLFs with adenoviruses was done at multiplicity of infection of 500. Three days after the transduction, expression collagen polypeptides was measured by protein gel blot.
Preparation of microsomes and immunoprecipitations
Microsomes were prepared according to,Citation32 with minor modifications. Approximately 2 × 107 HLFs were resuspended in 0.35 mL of hypotonic buffer and homogenized in Dounce homogenizer. The homogenate was adjusted to 1.9 M sucrose, layered on the top of 2.5 M sucrose cushion and overlayed with 1.3 M sucrose and centrifuged at 260,000 g for 3 h at 4°C in a Beckman MLS-50 rotor. The rough microsome fraction banding below the 1.3 M/1.9 M sucrose interface was collected and diluted in isotonic buffer and centrifuged in a Beckman TLA 120.2 rotor at 66,000 g for 20 min at 4°C. The pellet was lysed in 0.5 % NP-40, 50 mM Tris-HCl, pH 7.5, 150 mM NaCl and used for immunoprecipitations.
Immunoprecipitations were done using 1 μg SEC61β antibody (Thermo Scientific, PA3-015) which was incubated with the extract for 30 min at 4°C. 20 ul of equilibrated protein A/G beads were then added and incubated for additional 30 min. Control reactions received only the equilibrated A/G beads. The beads were washed 4 times and the samples analyzed by Western blotting.
Separation of cytosolic and membrane fractions of cells, without isolation of microsomes, was done as described in.Citation32
Western blots
Western blots were done with 20 μl of cellular extracts of transfected cells. The same amount was used in gel shift experiments, so that the protein expression could be directly compared to the binding activity. For measuring collagen secretion into the cellular medium, cells were washed with serum free medium and incubated in serum free medium for 3 h. The medium was collected and collagen secreted was directly measured by western blot. The antibodies used were: anti-collagen α1(I) from Rockland (600-401-103), anti-collagen α2(I) from Santa Cruz Biotechnology (sc-8786), anti-HA from Sigma (H9658), anti-calnexin and anti-fibronectin from BD Transduction Laboratories (610523 and 610077), anti-SEC61α from Upstate (07-204), anti-actin from Abcam (ab8227), anti-LARP6 from Novus Biologicals (H00055323) and anti-SEC61β from Thermo Scientific (PA3-015).
Gel mobility shift experiments
Cell extracts were prepared by lysis in 140 mM KCl, 10 mM MgCl2, 0.5% NP-40 and equal amounts (20 μl) were used for gel mobility shift. The 5’SL RNA probes were prepared by in vitro transcription in the presence of 32P-UTP from templates containing 5’SL sequence of human collagen α1(I) and α2(I) mRNAs or various mutants of the α1(I) 5’SL. The mutations made are indicated in . The competitor RNAs were prepared by in vitro transcription without radio-labeling. The amount of probe used in the reactions was typically 4 ng and the binding reaction contained 2 μg of yeast t-RNA and 1 μg of total liver RNA to suppress nonspecific binding. After incubation at RT for 30 min the reactions were resolved on 6% native gels. When bacterially expressed and purified LR-LARP6 was used there was no t-RNA or liver RNA in the reaction. Detection of the bands was by autoradiography or by phosphoimaging. For supershift experiments 1 μl of indicated antibodies was added to the reaction.
For competition of the preformed LR-LARP6/5’SL complexes, the amount of bacterially expressed LR-LARP6 that completely saturated 7 ng of the radio-labeled probe was empirically determined. Then, this amount was mixed with the probe and incubated at RT for 10 min, the indicated molar amounts of cold competitor RNAs were added and the incubation was continued for additional 30 min, when the complexes were resolved on native gels. Time dependent competition experiments were done by adding 200-fold molar excess of competitor RNA for different time periods, incubated on ice and then all time points were loaded at the same time onto native gels.
Fluorescence polarization (FP)
5’SL RNA and its various mutants labeled at the 5’ end with fluorescein were purchased from Dharmacon. 1 nM of fl-5’SL RNA was incubated in 100 mM NaCl, 10 mM Tris pH 7.5, 5 mM MgCl2, 0.25% NP-40 with increasing amounts of bacterially expressed LR-LARP6 for 10 min at RT in 384 well plates in total volume of 25 μl/well and FP was read in Biotek Synergy H1 plate reader. For titration of LARP6 with 5’SL RNA, 4 nM LARP6 was incubated with increasing amounts of fl-5’SL RNA. For each fl-5’SL RNA concentration a blank without LARP6 was read and the FP of the blank was subtracted from the FP of the corresponding fl-5’SL-LARP6 reaction. All reactions were performed in triplicates and apparent dissociation constants (Kd) were estimated by nonlinear curve fitting as the concentration which gives 50% saturation. After reading the FP, some reactions were directly loaded on gel shift gels and bands were visualized by imaging the fluorescence signals.
For the time course of decay of 5’SL/LR-LARP6 complexes, saturating amounts of LR-LARP6 were added to 1 nM fl-5’SL RNA in total volume of 100 μl in 96-well plate and 200 fold excess of unlabeled 5’ SL competitor RNA was added. 2 min after the addition of the competitor, FP was measured in triplicates at 1 min intervals for additional 45 min. FP of the blank without LR-LARP6 was not subtracted from these readings and FP was plotted vs time. The decay rate was inferred from the slopes of the decay curves.
Determination of protein folding
Unfolding of Larp6 and mutants was monitored by fluorescence (λex = 295 nm, λem = 350 nm; 5 nm slit widths) using a Cary Eclipse spectrophotometer (Agilent Technologies, Santa Clara, California) equipped with a Peltier temperature control. 5 μM samples of Larp6 in PBS buffer were equilibrated for 10 minutes at 283 K prior to heating. Samples were heated at a rate of 0.50 K / min. The resulting thermal unfolding curves were fit to a 6-parameter, 2-state denaturation model, assuming a negligible change in heat capacity upon unfolding.Citation60 Curve fitting was performed by the nonlinear, least squares fitting program, DataFit (Oakdale Engineering).
Disclosure of Potential Conflicts of Interest
No potential conflicts of interest were disclosed.
996467_suppl.zip
Download Zip (1.3 MB)Funding
This work was supported in part from the NIH grant 2R01DK059466-07A2 and Florida Department of Health grant 0000024303 to B. S.
Supplemental Materials
Supplemental data for this article can be accessed on the publisher's website.
References
- Uitto J. Collagen polymorphism: isolation and partial characterization of alpha 1(I)-trimer molecules in normal human skin. Arch Biochem Biophys 1979; 192:371-9; PMID:434832; http://dx.doi.org/10.1016/0003-9861(79)90105-X
- Bachmann A, Kiefhaber T, Boudko S, Engel J, Bachinger HP. Collagen triple-helix formation in all-trans chains proceeds by a nucleation/growth mechanism with a purely entropic barrier. Proc Natl Acad Sci U S A 2005; 102:13897-902; PMID:16172389; http://dx.doi.org/10.1073/pnas.0505141102
- Boudko S, Frank S, Kammerer RA, Stetefeld J, Schulthess T, Landwehr R, Lustig A, Bächinger HP, Engel J. Nucleation and propagation of the collagen triple helix in single-chain and trimerized peptides: transition from third to first order kinetics. J Mol Biol 2002; 317:459-70; PMID:11922677; http://dx.doi.org/10.1006/jmbi.2002.5439
- Kivirikko KI. Collagen biosynthesis: a mini-review cluster. Matrix Biol 1998; 16:355-6; PMID:9524355; http://dx.doi.org/10.1016/S0945-053X(98)90008-7
- Myllyharju J, Kivirikko KI. Collagens, modifying enzymes and their mutations in humans, flies and worms. Trends Genet 2004; 20:33-43; PMID:14698617; http://dx.doi.org/10.1016/j.tig.2003.11.004
- Pace JM, Wiese M, Drenguis AS, Kuznetsova N, Leikin S, Schwarze U, Chen D, Mooney SH, Unger S, Byers PH. Defective C-propeptides of the proalpha2(I) chain of type I procollagen impede molecular assembly and result in osteogenesis imperfecta. J Biol Chem 2008; 283:16061-7; PMID:18375391; http://dx.doi.org/10.1074/jbc.M801982200
- Tajima S, Takehana M, Azuma N. Production of overmodified type I procollagen in a case of osteogenesis imperfecta. J Dermatol 1994; 21:219-22; PMID:8056893
- Beck K, Boswell BA, Ridgway CC, Bachinger HP. Triple helix formation of procollagen type I can occur at the rough endoplasmic reticulum membrane. J Biol Chem 1996; 271:21566-73; PMID:8702943; http://dx.doi.org/10.1074/jbc.271.35.21566
- Yamada Y, Mudryj M, de Crombrugghe B. A uniquely conserved regulatory signal is found around the translation initiation site in three different collagen genes. J Biol Chem 1983; 258:14914-9; PMID:6689169
- Cai L, Fritz D, Stefanovic L, Stefanovic B. Nonmuscle myosin-dependent synthesis of type I collagen. J Mol Biol 2010; 401:564-78; PMID:20603131; http://dx.doi.org/10.1016/j.jmb.2010.06.057
- Cai L, Fritz D, Stefanovic L, Stefanovic B. Binding of LARP6 to the conserved 5' stem-loop regulates translation of mRNAs encoding type I collagen. J Mol Biol 2010; 395:309-26; PMID:19917293; http://dx.doi.org/10.1016/j.jmb.2009.11.020
- Bousquet-Antonelli C, Deragon JM. A comprehensive analysis of the La-motif protein superfamily. RNA 2009; 15:750-64; PMID:19299548; http://dx.doi.org/10.1261/rna.1478709
- Parsons CJ, Stefanovic B, Seki E, Aoyama T, Latour AM, Marzluff WF, Rippe RA, Brenner DA. Mutation of the 5' untranslated region stem-loop structure inhibits {alpha}1(i) collagen expression in vivo. J Biol Chem 2011; 286:8609-19; PMID:21193410; http://dx.doi.org/10.1074/jbc.M110.189118
- Manojlovic Z, Stefanovic B. A novel role of RNA helicase A in regulation of translation of type I collagen mRNAs. RNA 2012; 18:321-34; PMID:22190748; http://dx.doi.org/10.1261/rna.030288.111
- Challa AA, Stefanovic B. A novel role of vimentin filaments: binding and stabilization of collagen mRNAs. Mol Cell Biol 2011; 31:3773-89; PMID:21746880; http://dx.doi.org/10.1128/MCB.05263-11
- Zeisberg M, Neilson EG. Mechanisms of tubulointerstitial fibrosis. J Am Soc Nephrol 2010; 21:1819-34; PMID:20864689; http://dx.doi.org/10.1681/ASN.2010080793
- Shahbaz AU, Sun Y, Bhattacharya SK, Ahokas RA, Gerling IC, McGee JE, Weber KT. Fibrosis in hypertensive heart disease: molecular pathways and cardioprotective strategies. J Hypertens 2010; 28(Suppl 1):S25-32; PMID:20823713; http://dx.doi.org/10.1097/01.hjh.0000388491.35836.d2
- Rombouts K, Marra F. Molecular mechanisms of hepatic fibrosis in non-alcoholic steatohepatitis. Dig Dis 2010; 28:229-35; PMID:20460917; http://dx.doi.org/10.1159/000282094
- Pinzani M, Macias-Barragan J. Update on the pathophysiology of liver fibrosis. Expert Rev Gastroenterol Hepatol 2010; 4:459-72; PMID:20678019; http://dx.doi.org/10.1586/egh.10.47
- Henderson BR, Saeedi BJ, Campagnola G, Geiss BJ. Analysis of RNA binding by the dengue virus NS5 RNA capping enzyme. PLoS One 2011; 6:e25795; PMID:22022449; http://dx.doi.org/10.1371/journal.pone.0025795
- Mao C, Flavin KG, Wang S, Dodson R, Ross J, Shapiro DJ. Analysis of RNA-protein interactions by a microplate-based fluorescence anisotropy assay. Anal Biochem 2006; 350:222-32; PMID:16448619; http://dx.doi.org/10.1016/j.ab.2005.12.010
- Rau M, Stump WT, Hall KB. Intrinsic flexibility of snRNA hairpin loops facilitates protein binding. RNA 2012; 18:1984-95; PMID:23012481; http://dx.doi.org/10.1261/rna.035006.112
- Xing L, Niu M, Kleiman L. In vitro and in vivo analysis of the interaction between RNA helicase A and HIV-1 RNA. J Virol 2012; 86:13272-80; PMID:23015696; http://dx.doi.org/10.1128/JVI.01993-12
- Alfano C, Sanfelice D, Babon J, Kelly G, Jacks A, Curry S, Conte MR. Structural analysis of cooperative RNA binding by the La motif and central RRM domain of human La protein. Nat Struct Mol Biol 2004; 11:323-9; PMID:15004549; http://dx.doi.org/10.1038/nsmb747
- Maraia RJ. La protein and the trafficking of nascent RNA polymerase iii transcripts. J Cell Biol 2001; 153:F13-8; PMID:11352926; http://dx.doi.org/10.1083/jcb.153.4.F13
- Maraia RJ, Intine RV. La protein and its associated small nuclear and nucleolar precursor RNAs. Gene Expr 2002; 10:41-57; PMID:11868987
- Teplova M, Yuan YR, Phan AT, Malinina L, Ilin S, Teplov A, Patel DJ. Structural basis for recognition and sequestration of UUU(OH) 3' temini of nascent RNA polymerase III transcripts by La, a rheumatic disease autoantigen. Mol Cell 2006; 21:75-85; PMID:16387655; http://dx.doi.org/10.1016/j.molcel.2005.10.027
- Shiromizu T, Adachi J, Watanabe S, Murakami T, Kuga T, Muraoka S, Tomonaga T. Identification of missing proteins in the neXtProt database and unregistered phosphopeptides in the PhosphoSitePlus database as part of the Chromosome-centric Human Proteome Project. J Proteome Res 2013; 12:2414-21; PMID:23312004; http://dx.doi.org/10.1021/pr300825v
- Becker T, Bhushan S, Jarasch A, Armache JP, Funes S, Jossinet F, Gumbart J, Mielke T, Berninghausen O, Schulten K, et al. Structure of monomeric yeast and mammalian Sec61 complexes interacting with the translating ribosome. Science 2009; 326:1369-73; PMID:19933108; http://dx.doi.org/10.1126/science.1178535
- Dejgaard K, Theberge JF, Heath-Engel H, Chevet E, Tremblay ML, Thomas DY. Organization of the Sec61 translocon, studied by high resolution native electrophoresis. J Proteome Res 2010; 9:1763-71; PMID:20112977; http://dx.doi.org/10.1021/pr900900x
- Gogala M, Becker T, Beatrix B, Armache JP, Barrio-Garcia C, Berninghausen O, Beckmann R. Structures of the Sec61 complex engaged in nascent peptide translocation or membrane insertion. Nature 2014; 506:107-10; PMID:24499919; http://dx.doi.org/10.1038/nature12950
- Stephens SB, Dodd RD, Lerner RS, Pyhtila BM, Nicchitta CV. Analysis of mRNA partitioning between the cytosol and endoplasmic reticulum compartments of mammalian cells. Methods Mol Biol 2008; 419:197-214; PMID:18369985; http://dx.doi.org/10.1007/978-1-59745-033-1_14
- Bergeron JJ, Brenner MB, Thomas DY, Williams DB. Calnexin: a membrane-bound chaperone of the endoplasmic reticulum. Trends Biochem Sci 1994; 19:124-8; PMID:8203019; http://dx.doi.org/10.1016/0968-0004(94)90205-4
- Challa AA, Vukmirovic M, Blackmon J, Stefanovic B. Withaferin-A reduces type I collagen expression in vitro and inhibits development of myocardial fibrosis in vivo. PLoS One 2012; 7:e42989; PMID:22900077; http://dx.doi.org/10.1371/journal.pone.0042989
- Manojlovic Z, Blackmon J, Stefanovic B. Tacrolimus (FK506) prevents early stages of ethanol induced hepatic fibrosis by targeting LARP6 dependent mechanism of collagen synthesis. PLoS One 2013; 8:e65897; PMID:23755290; http://dx.doi.org/10.1371/journal.pone.0065897
- Stefanovic B, Brenner DA. 5' stem-loop of collagen alpha 1(I) mRNA inhibits translation in vitro but is required for triple helical collagen synthesis in vivo. J Biol Chem 2003; 278:927-33; PMID:12419812; http://dx.doi.org/10.1074/jbc.M209175200
- Stefanovic B, Hellerbrand C, Brenner DA. Regulatory role of the conserved stem-loop structure at the 5' end of collagen alpha1(I) mRNA. Mol Cell Biol 1999; 19:4334-42; PMID:10330174
- Stefanovic B, Lindquist J, Brenner DA. The 5' stem-loop regulates expression of collagen alpha1(I) mRNA in mouse fibroblasts cultured in a three-dimensional matrix. Nucleic Acids Res 2000; 28:641-7; PMID:10606666; http://dx.doi.org/10.1093/nar/28.2.641
- Stefanovic B, Schnabl B, Brenner DA. Inhibition of collagen alpha 1(I) expression by the 5' stem-loop as a molecular decoy. J Biol Chem 2002; 277:18229-37; PMID:11889120; http://dx.doi.org/10.1074/jbc.M108065200
- Vukmirovic M, Manojlovic Z, Stefanovic B. Serine-threonine kinase receptor-associated protein (STRAP) regulates translation of type I collagen mRNAs. Mol Cell Biol 2013; 33:3893-906; PMID:23918805; http://dx.doi.org/10.1128/MCB.00195-13
- Lindquist JN, Parsons CJ, Stefanovic B, Brenner DA. Regulation of alpha1(I) collagen messenger RNA decay by interactions with alphaCP at the 3'-untranslated region. J Biol Chem 2004; 279:23822-9; PMID:14973140; http://dx.doi.org/10.1074/jbc.M314060200
- Wang ZF, Whitfield ML, Ingledue TC, 3rd, Dominski Z, Marzluff WF. The protein that binds the 3' end of histone mRNA: a novel RNA-binding protein required for histone pre-mRNA processing. Genes Dev 1996; 10:3028-40; PMID:8957003; http://dx.doi.org/10.1101/gad.10.23.3028
- Ke Y, Wu J, Leibold EA, Walden WE, Theil EC. Loops and bulge/loops in iron-responsive element isoforms influence iron regulatory protein binding. Fine-tuning of mRNA regulation? J Biol Chem 1998; 273:23637-40; PMID:9726965; http://dx.doi.org/10.1074/jbc.273.37.23637
- Rana TM, Jeang KT. Biochemical and functional interactions between HIV-1 Tat protein and TAR RNA. Arch Biochem Biophys 1999; 365:175-85; PMID:10328810; http://dx.doi.org/10.1006/abbi.1999.1206
- Zhang J, Tan D, DeRose EF, Perera L, Dominski Z, Marzluff WF, Tong L, Hall TM. Molecular mechanisms for the regulation of histone mRNA stem-loop-binding protein by phosphorylation. Proc Natl Acad Sci U S A 2014; 111:E2937-46; PMID:25002523; http://dx.doi.org/10.1073/pnas.1406381111
- Tan D, Marzluff WF, Dominski Z, Tong L. Structure of histone mRNA stem-loop, human stem-loop binding protein, and 3'hExo ternary complex. Science 2013; 339:318-21; PMID:23329046; http://dx.doi.org/10.1126/science.1228705
- Wang H, Stefanovic B. Role of LARP6 and Nonmuscle Myosin in Partitioning of Collagen mRNAs to the ER Membrane. PLoS One 2014; 9:e108870; PMID:25271881; http://dx.doi.org/10.1371/journal.pone.0108870
- Chen C, Michelini-Norris B, Stevens S, Rowsey J, Ren X, Goldstein M, Schultz G. Measurement of mRNAs for TGFss and extracellular matrix proteins in corneas of rats after PRK. Invest Ophthalmol Vis Sci 2000; 41:4108-16; PMID:11095603
- Ford CM, Li S, Pickering JG. Angiotensin II stimulates collagen synthesis in human vascular smooth muscle cells. Involvement of the AT(1) receptor, transforming growth factor-beta, and tyrosine phosphorylation. Arterioscler Thromb Vasc Biol 1999; 19:1843-51; PMID:10446062; http://dx.doi.org/10.1161/01.ATV.19.8.1843
- Kelkar A, Dobberstein B. Sec61beta, a subunit of the Sec61 protein translocation channel at the endoplasmic reticulum, is involved in the transport of Gurken to the plasma membrane. BMC Cell Biol 2009; 10:11; PMID:19226464; http://dx.doi.org/10.1186/1471-2121-10-11
- Stefanovic B, Stefanovic L, Schnabl B, Bataller R, Brenner DA. TRAM2 protein interacts with endoplasmic reticulum Ca2+ pump Serca2b and is necessary for collagen type I synthesis. Mol Cell Biol 2004; 24:1758-68; PMID:14749390; http://dx.doi.org/10.1128/MCB.24.4.1758-1768.2004
- Cheng Z. Protein translocation through the Sec61/SecY channel. Biosci Rep 2010; 30:201-7; PMID:20156192; http://dx.doi.org/10.1042/BSR20090158
- Park E, Rapoport TA. Mechanisms of Sec61/SecY-mediated protein translocation across membranes. Annu Rev Biophys 2012; 41:21-40; PMID:22224601; http://dx.doi.org/10.1146/annurev-biophys-050511-102312
- Miles CA, Sims TJ, Camacho NP, Bailey AJ. The role of the alpha2 chain in the stabilization of the collagen type I heterotrimer: a study of the type I homotrimer in oim mouse tissues. J Mol Biol 2002; 321:797-805; PMID:12206762; http://dx.doi.org/10.1016/S0022-2836(02)00703-9
- Sims TJ, Miles CA, Bailey AJ, Camacho NP. Properties of collagen in OIM mouse tissues. Connect Tissue Res 2003; 44(Suppl 1):202-5; PMID:12952198; http://dx.doi.org/10.1080/03008200390181663
- Malfait F, Symoens S, Coucke P, Nunes L, De Almeida S, De Paepe A. Total absence of the alpha2(I) chain of collagen type I causes a rare form of Ehlers-Danlos syndrome with hypermobility and propensity to cardiac valvular problems. J Med Genet 2006; 43:e36; PMID:16816023; http://dx.doi.org/10.1136/jmg.2005.038224
- He TC, Zhou S, da Costa LT, Yu J, Kinzler KW, Vogelstein B. A simplified system for generating recombinant adenoviruses. Proc Natl Acad Sci U S A 1998; 95:2509-14; PMID:9482916; http://dx.doi.org/10.1073/pnas.95.5.2509
- Griffiths CM, Page MJ. Production of heterologous proteins using the baculovirus/insect expression system. Methods Mol Biol 1997; 75:427-40; PMID:9276289
- Yamada NA, Castro A, Farber RA. Variation in the extent of microsatellite instability in human cell lines with defects in different mismatch repair genes. Mutagenesis 2003; 18:277-82; PMID:12714694; http://dx.doi.org/10.1093/mutage/18.3.277
- Shih P, Holland DR, Kirsch JF. Thermal stability determinants of chicken egg-white lysozyme core mutants: hydrophobicity, packing volume, and conserved buried water molecules. Protein Sci 1995; 4:2050-62; PMID:8535241; http://dx.doi.org/10.1002/pro.5560041010