ABSTRACT
The function of 6S RNA, a global regulator of transcription, was studied in the photosynthetic α-proteobacterium Rhodobacter sphaeroides. The cellular levels of R. sphaeroides 6S RNA peak toward the transition to stationary phase and strongly decrease during extended stationary phase. The synthesis of so-called product RNA transcripts (mainly 12–16-mers) on 6S RNA as template by RNA polymerase was found to be highest in late exponential phase. Product RNA ≥ 13-mers are expected to trigger the dissociation of 6S RNA:RNA polymerase complexes. A 6S RNA deletion in R. sphaeroides had no impact on growth under various metabolic and oxidative stress conditions (with the possible exception of tert-butyl hydroperoxide stress). However, the 6S RNA knockout resulted in a robust growth defect under high salt stress (0.25 M NaCl). Remarkably, the sspA gene encoding the putative salt stress-induced membrane protein SspA and located immediately downstream of the 6S RNA (ssrS) gene on the antisense strand was expressed at elevated levels in the ΔssrS strain when grown in the presence of 250 mM NaCl.
Introduction
Escherichia coli 6S RNA was one of the first non-coding RNAs to be discovered.Citation1 First insights into its function emerged when Escherichia coli 6S RNA was found to bind to the housekeeping RNA polymerase holoenzyme (σ70-RNAP).Citation2 As the expression of 6S RNA increases more than 10-fold toward stationary phase in E. coli,Citation2 the RNA efficiently represses a multitude of σ70-dependent promoters at the end of exponential growth. This supports the expression of many stationary phase-specific (σ38-dependent) genes as part of the metabolic adaptation at the transition from exponential to stationary phase.Citation3,Citation4,Citation5 6S RNA, which is thought to mimic an open DNA promoter structure, adopts a rod-shaped structure with a large internal loop (termed “central bulge”) flanked by predominantly helical arms on both sides.Citation6,Citation7,Citation8 Its apical arm and parts of the central bulge were inferred to contact the housekeeping sigma factor (σ70 in E. coli),Citation4,Citation9,Citation10 explaining its competition with σ70-dependent DNA promoters for RNAP binding. Part of the central bulge and roughly the internal half of the terminal stem were modeled to be in contact with the β subunit of RNAP.Citation10 6S RNAs are little conserved on the sequence level explaining its only sporadic discoveryCitation11 in other bacteria than E. coli before 2005. Yet, thereafter, computational approaches interrogating conserved secondary structural features accelerated its massive identification in the majority of bacteria.Citation6,Citation7,Citation12 A recent bioinformatic approach identified at least one (and in most cases a single) 6S RNA gene candidate in 1610 bacterial species.Citation12
E. coli (γ-proteobacteria) and Bacillus subtilis (Firmicutes) have served as model organisms to study the growth phase-dependent expression of 6S RNAs and their physiologic function (reviewed inCitation10,Citation13). A milestone in 6S RNA research was the finding that RNAP utilizes 6S RNA as a template for the transcription of short product RNAs (pRNAs) which, if long enough (∼ ≥ 13 nt in E. coli), persistently rearrange the structure of 6S RNA such that RNAP is released from the sequestration by 6S RNA.Citation14,Citation15 Two major types of 6S RNA have been identified, which are represented by the two 6S RNAs (6S-1 and 6S-2 RNA) found in B. subtilis and other Firmicutes. 6S-1 RNA, the functional homolog of E. coli 6S RNA, is expressed at low levels during exponential growth and accumulates toward stationary phase. During outgrowth from stationary phase (reinitiation of exponential growth upon culture dilution in fresh medium), which correlates with elevated NTP levels, RNAP utilizes 6S-1 RNA for massive synthesis of pRNAs whose length pattern is shifted toward longer variants during outgrowth relative to stationary phase.Citation16,Citation17 The second 6S-2 RNA in B. subtilis has a distinct expression profile, with high levels in early exponential phase that either decrease or remain fairly constant toward stationary phase, possibly depending on the growth conditions and/or the type of B. subtilis strain investigated (reviewed inCitation10). Synthesis of pRNAs from 6S-2 RNA is less efficient than from 6S-1 RNA,Citation18,Citation19 a major reason being that 6S-2 pRNA transcription is initiated with ATP which is less efficiently used by B. subtilis σA-RNAP than GTP, the initiator nucleotide for 6S-1 pRNA synthesis.Citation16,Citation20 Such a preference for GTP as initiator nucleotide is an idiosyncrasy of B. subtilis σA-RNAP, it was not observed for E. coli σ70-RNAP.Citation20
Members of the α-proteobacteria have been intensively studied because of their interaction with animal or plant hosts and their metabolic versatility including aerobic or anaerobic respiration and anoxygenic photosynthesis, which allows the efficient adaptation to different environments and growth conditions. Among them, Rhodobacter sphaeroides is an established model organism to study the regulated formation of photosynthetic complexes and the response to (photo)oxidative stresses. Considering the specific metabolic capacities of the bacterium and its complex adaptations to environmental changes, we studied the role of 6S RNA in these processes, as the RNA contributes to the global regulation of the transcriptome. This was addressed by Northern blot and RNA-Seq analyses of 6S RNA and 6S RNA-templated pRNA transcripts. 6S RNA knockout strains were tested for growth and stress response phenotypes and effects on the formation of photosynthetic complexes. This revealed a distinct growth defect of the 6S RNA deletion strain under high salt conditions.
Results
The 6S RNA gene of R. sphaeroides 2.4.1
The 6S RNA of R. sphaeroides, originally termed RSs2363, was first identified in an RNA-Seq-based screen for small non-coding RNAs.Citation21 Bioinformatic analyses later confirmed that R. sphaeroides encodes a single 6S RNA gene (RSP_7709,Citation12), termed ssrS by analogy to the E. coli nomenclature (). The ssrS gene is preceded by a tRNAHis gene (RSP_4336). Downstream of ssrS sits the sspA gene (RSP_0753) which is transcribed from the opposite strand and encodes a putative membrane protein involved in responses to high salt stressCitation22 (). 5′-TTG (-35) and CTATCT (-10) promoter motifsCitation23 upstream of the putative transcriptional start site (, highlighted in gray) suggest that the transcription start corresponds to the mature 5′-end of 6S RNA, which is in line with the RNA-Seq data (see below). The major mature R. sphaeroides 6S RNA species comprises 162 nt, and the canonical 6S RNA structure shown in is within the ensemble of the most stable, nearly isoenergetic 20 structures (93.5 to 100%) predicted by mfold.Citation24 Differential RNA-Seq results are shown for the 6S RNA locus in (the read distribution for the entire region as depicted in is illustrated in Fig. S1). 5′-Terminal reads assignable to mature 6S RNA accumulated upon enrichment of 5′-triphosphate-bearing primary transcripts (, top, + TEX), supporting the notion that the 5′-end of mature 6S RNA is indeed the transcription start site as indicated in . Unlike the 5′-terminal 6S RNA reads, those corresponding to 3′-terminal fragments were more abundant in RNA preparations without TEX treatment (except for the outgrowth phase). An explanation could be that these 3′-fragments with defined 5′-end derived from a processing event that generates 5′-monophosphate ends, which are depleted in RNA pools upon TEX treatment. Remarkably, the 6S RNA gene is followed by 13 repeats of the sequence motif GGGCCAA (). RNA-Seq detected only very few reads extending into this region (Fig. S1).
Figure 1. (A) Genomic environment of the 6S RNA locus (ssrS, RSP_7709) in R. sphaeroides 2.4.1. RSP_4336 encodes a tRNAHis and sspA (RSP_0753) a putative membrane protein involved in responses to high salt stress. At the bottom, the nucleotide numbering of R. sphaeroides 2.4.1 (NC_007493.2) chromosome 1 is given. (B) Genetic elements in the immediate vicinity of the 6S DNA (in bold capital letters); promoter elements23 are highlighted in gray, the 5′-GGGCCAA repeat region is underlined; the genomic nucleotide numbers are given for the first and last shown nucleotide. (C) Predicted secondary structure of R. sphaeroides 6S RNA; the arrow indicates the initiation site of pRNA transcription; the template region for the first 12 pRNA nucleotides is depicted in green (for more details, see text). (D) Screenshot (Integrated Genome Browser 8.1.9 software, Affymetrix) of cDNA reads representing fragments of R. sphaeroides 6S RNA (top part) as well as antisense reads (bottom part). The sequence below the top part corresponds to 6S DNA (panel C) plus 8 and 9 nucleotides up- and downstream, respectively. The genomic numbering is provided below the 6S DNA sequence. The DNA sequence stretch above the bottom panel corresponds to pRNA reads. For RNA-Seq, total RNA was extracted from microaerobically growing cells in late exponential phase (exp.) at ∼0.5 OD660, at the transition (trans.) to stationary phase (stat.) at ∼1.5 OD660, after 12 h in stationary phase (at ∼2.0 OD660) and after 20 min of outgrowth (OD660 of 0.21 at RNA extraction; see Fig. S5 for details); outgrowth was initiated by diluting cells kept for an extended period (∼60 h) in stationary phase 1:5 (from OD660 ∼1.0 to 0.2) in fresh medium (Fig. S5). RNA samples were split into 2 halves; one half was pretreated with Terminator™ 5′-Phosphate-Dependent Exonuclease (+ TEX) before cDNA library construction to degrade RNAs with 5′-monophosphates, which leads to an enrichment of primary transcripts (RNAs with 5′-triphosphates). The number of cDNA reads is given on the y-axis (1k = 1000 reads).
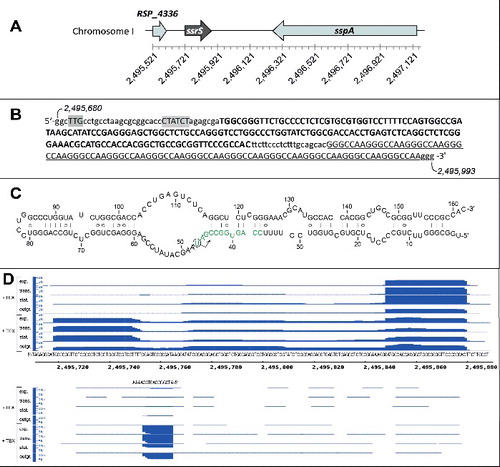
Growth phase-dependent expression of 6S RNA and pRNAs from R. sphaeroides
Expression profiles of R. sphaeroides 6S RNA were analyzed under 3 typical growth conditions: aerobic, microaerobic and phototrophic. Aerobic and microaerobic growth was performed in the dark at 32°C under shaking with 160–180 μM (aerobic) or 25 μM (microaerobic) of dissolved oxygen. Phototrophic growth was maintained by illumination with white light without shaking (for additional details, see Materials and methods). Corresponding growth curves are shown in . In the presence of oxygen, R. sphaeroides performs aerobic respiration, while anoxygenic photosynthesis (phototrophic growth) generates ATP in the presence of light and absence of oxygen. R. sphaeroides can also grow by anaerobic respiration in the absence of oxygen and light when a suitable electron acceptor, such as DMSO, is present. However, growth by anaerobic respiration is even slower than phototrophic growth (see Fig. S2) and was thus not considered further here.
Figure 2. Growth phase-dependent levels of 6S RNA in R. sphaeroides. Growth curves of R. sphaeroides 2.4.1 cultivated under (A) microaerobic, (B) aerobic and (C) phototrophic conditions are shown at the top. The optical density (OD) was measured at 660 nm (log scale). Colored circles, indicating the time points of sample withdrawal for total RNA extraction, are correlated by dashed lines with the corresponding Northern blot lanes in the bottom panels. 6S RNA signal intensities were quantified by the Quantity One Software (Bio-Rad) and normalized to the corresponding 5S rRNA signal intensities. Mean values of normalized 6S RNA intensities were derived from 3 or more independent experiments, with the exception of 18, 27 and 42 h in panel B, which were based on 2 experiments only. The mean values of normalized 6S RNA intensities were finally normalized to the mean value of the sample with the lowest 6S RNA level, which was set to 1, yielding the plotted values of “relative 6S RNA level." Error bars indicate standard deviations. The shown Northern blots are representative example experiments.
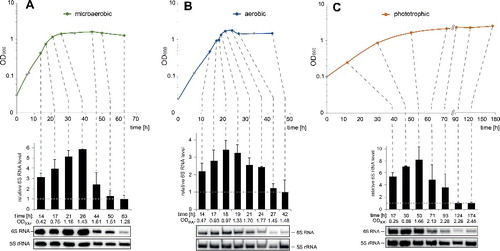
6S RNA levels were quantified by Northern blotting at selected time points along the growth curves (). The radioactive 6S RNA signals were quantified and normalized to the corresponding 5S rRNA signals. In all cases, the weakest 6S RNA signals were obtained at the last time points (extended stationary phase; these values were set to 1 in the graphs above the Northern blot signals in ). Under all 3 growth conditions, 6S RNA levels remained high (or even increased) up to the transition to stationary phase and then progressively decreased during extended stationary phase. When stationary cells enter a new exponential growth phase, cellular 6S RNA levels are built up again, consistent with the finding that the levels are lower at early (time points 1 in Fig. S3) relative to mid or late exponential phase (time points 2 and 3 in Fig. S3).
We also tested the stability of 6S RNA after blocking transcription with rifampicin. No decrease of the 6S RNA level was observed within 4 h after rifampicin addition (Fig. S4). As a control we also probed the sRNA SorYCitation25 whose levels progressively decreased in the same time window after rifampicin addition. Unaltered 6S RNA levels after rifampicin addition were also observed for B. subtilis 6S-1 RNA and were interpreted to reflect protection of 6S RNA from RNases by the housekeeping RNA polymerase holoenzyme. As rifampicin also blocks pRNA transcription from 6S RNA as template, the rapid pRNA-induced 6S RNA release from RNA polymerase cannot occur, which is thought to be a prerequisite for fast 6S RNA decay.Citation17
The synthesis of short pRNA transcripts by RNA polymerase using 6S RNA as a template is a hallmark of 6S RNA function. Cellular pRNA pools were analyzed by RNA-Seq using R. sphaeroides total RNAs extracted from cells in late exponential phase, at the transition to stationary phase, in stationary phase and during outgrowth from stationary phase which corresponds to a new exponential growth phase (for details, see Fig. S5). Total RNAs were additionally split in 2 fractions, of which one was pretreated with Terminator™ 5′-Phosphate-Dependent Exonuclease (TEX) to degrade RNAs carrying 5’-monophosphate ends, which results in an enrichment of primary transcripts carrying 5′-triphosphate ends (differential RNA-Seq,Citation26). Such an enrichment upon TEX pretreatment was previously observed for pRNAs derived from B. subtilis 6S-1 and 6S-2 RNAs.Citation16,Citation18
RNA-Seq indeed identified pRNAs derived from R. sphaeroides 6S RNA, whose transcription is initiated in the 5′-central bulge (at U47, , ) as expected for canonical 6S RNAs.Citation10,Citation27 The number of pRNA length variants in the various libraries are summarized in . Only perfectly matching reads ≥ 9 nt were included in the analysis. All pRNA reads had the same 5′-terminus and TEX treatment substantially increased their number, in line with their nature as genuine pRNA primary transcripts. The majority of reads were 9 to 16-mers; we could not differentiate between 12 to 16-mers, because RNAs were poly(A)-tailed during library construction, such that the 4 A residues at pRNA positions 12 to 16 could not be resolved as originating from the pRNA. Reads corresponding to pRNA 17 to 20-mers were most abundant in the exponential RNA library; reads representing pRNAs longer than 20 nt were generally rare, and among those 36–40-mers predominated, which are close in length to runoff transcripts. The read numbers were lowest in the libraries of cells from stationary phase (). To validate the RNA-Seq data by an independent method, we performed Northern blots using EDC-crosslinking and mixed DNA/LNA probes (; see Material and methods) as well as 32P-labeled DNA oligonucleotide probes (). The Northern blot signals were relatively highest during late exponential growth (, , lanes 1), decreased toward stationary phase (lanes 2 and 3) and substantially decreased during extended stationary phase (lanes 4−6). During outgrowth from stationary phase, the signal increased again (lanes 7) to decline again toward the next stationary phase (lanes 8). The Northern blot signals in the cellular RNA lanes appeared roughly at the same gel position as a synthetic R. sphaeroides pRNA 14-mer (, , lanes PRsp), indicating that primarily pRNAs > 12 nt contributed to the Northern blot signals. This can be explained by smaller pRNAs dissociating from the probe during wash steps and hybridization at elevated temperature. If one counts the reads ≥ 12 nt in the “+ TEX” columns of , the sums are 16076 for the exponential, 14909 for the transition, 6759 for the stationary and 7720 for the outgrowth library; the time points of cell withdrawal for the RNA-Seq library preparations roughly corresponded to time points 1, 2, 3 and 7, respectively, in . Thus, the signal intensities in and read numbers in show the same trends, but a quantitative comparison remains coarse, as pRNA 12 to 16-mers were not resolved by RNA-Seq (16-mers will contribute to Northern signals more than 12-mers owing to slower pRNA:probe dissociation rates). The Northern blot and RNA-Seq results clearly indicate that the synthesis of longer pRNAs decreases toward stationary phase, which can be attributed to the shortage of the NTP pool and a general reduction of transcriptional activity during stationary phase.
Table 1. Overview of the pRNA reads identified by RNA-Seq in RNA extracts of R. sphaeroides 2.4.1 prepared at different growth states (microaerobic conditions). No mismatches were allowed. Total RNA was extracted from microaerobic cultures at different stages; for details, see the legend to and Fig. S5. RNA samples were split into 2 halves; one half was pretreated with Terminator™ 5′-Phosphate-Dependent Exonuclease (+ TEX) before cDNA library construction to degrade RNAs with 5′-monophosphates, which leads to an enrichment of primary transcripts (RNAs with 5′-triphosphates) including pRNAs. 3′-Terminal A residues of pRNA length variants are given as small letters on the right, as they could not be unequivocally assigned to the respective pRNA variant because poly(A)-tailing was used for library construction. Above the table, the time point of sample withdrawal and RNA preparation is correlated with the corresponding time points in the growth curve of
Figure 3. pRNA expression profiles at different growth stages of R. sphaeroides 4.2.1 cells grown under microaerobic conditions, analyzed by Northern blotting. (A) Growth curve; time points at which samples were withdrawn for RNA extraction are numbered and marked by black circles. (B) Northern blots for the detection of pRNAs using the TRIzol RNA extraction protocol, non-denaturing PAGE (12%), EDC crosslinking and digoxigenin-labeled hybridization probes as described in Materials and methods; 4 μg RNA was loaded in each lane: lane pRsp, a chemically synthesized R. sphaeroides pRNA 14-mer (5′OH-AUC GGC CAC UGG AA-3′); lane pBsu, a pRNA 14-mer complementary to B. subtilis 6S-1 RNA (5′OH -GUU CGG UCA AAA CU-3′)34; lanes 1 to 8, total RNAs from cell samples specified in panel A. (C) Analysis of the same sample set as in panel B, but using only 2.4 μg RNA in each lane and denaturing (7 M urea) 12% PAGE, UV-crosslinking for RNA immobilization on membranes and a 5′-32P-labeled DNA probe for hybridization (for details, see Materials and methods). Note that migration of the synthetic pRNA 14-mer (pRsp) and endogenous pRNAs is not directly comparable, as the former carriers a 5′-OH end, while the latter are assumed to carry 5′-triphosphates.
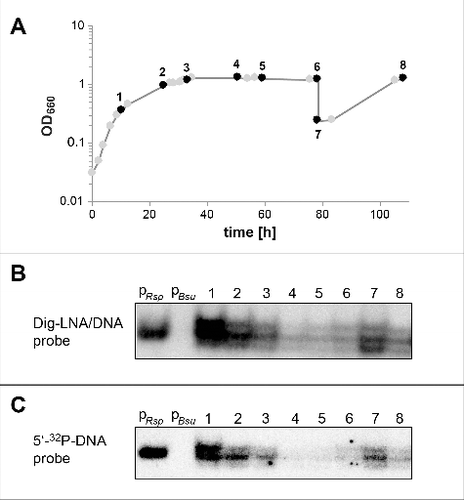
Deletion of 6S RNA affects growth under high salt
The accumulation of 6S RNA at the transition from exponential to stationary phase suggests a role in adaptation to stationary phase conditions. To test this hypothesis, we deleted the 6S RNA gene and compared the growth behavior of this mutant strain and the parental strain 2.4.1. The 6S RNA was initially replaced by a kanamycin cassette without transcriptional terminator generating strain 2.4.1 ΔssrS ( and S6). Later, we also constructed a second ssrS knockout (termed ΔssrS Ω) with the inserted kanamycin cassette including a Rho-independent transcription terminator to prevent transcriptional interference with the downstream sspA gene (). For the ΔssrS strain, we were unable to observe robust growth differences relative to the wild-type strain under standard growth conditions (Fig. S2).
Figure 4. (A) Genetic context of the ssrS/sspA region in the parental R. sphaeroides 4.2.1 strain (wt, top) and changes in the ΔssrS derivative strains (bottom). The 6S RNA sequence is followed by a region enriched in U residues on the transcript level (). It is assumed that the terminal stem of 6S RNA, resulting from pairing of the RNA's 5′- and 3′-terminal nucleotide stretches, forms cotranscriptionally and thus functions as a Rho-independent terminator (indicated by the dotted hairpin). Transcription of the sspA gene encoding the putative salt stress-induced membrane protein SspA from the antisense strand is terminated by a canonical strong Rho-independent terminator ∼25 nt downstream of the stop codon (dotted hairpin). In the strain ΔssrS, the 5′-proximal 125 nucleotides of the 6S DNA sequence are deleted, which abolishes the formation of the terminal 6S RNA stem and its assumed function in transcription termination. The inserted kanamycin cassette lacked a transcription terminator at its 3′-end in strain ΔssrS, while such a terminator was included in the kanamycin gene used for the construction of strain ΔssrS Ω (the difference is illustrated by the dotted hairpin in parentheses). Bottom scale: arbitrary numbering (in bp). (B, C) Deletion of the 6S RNA gene impairs growth of R. shpaeroides under high salt conditions. (B) Growth of the parental R. sphaeroides 2.4.1 wt strain and the 2 derived ΔssrS strains was monitored under microaerobic conditions in the presence of 250 mM NaCl. The optical density at 660 nm (OD660) was determined over time. Each time point represents the mean of 3 independent experiments, and error bars indicate standard deviations. (C) Estimation of 6S RNA levels in the wt strain under high salt stress. Northern blot analysis of total RNAs isolated from exponentially growing wt (lanes 1 and 3) and ΔssrS (lanes 2 and 4; used as probe specificity controls) cells 2.5 h after NaCl addition (+ NaCl) or mock-treated (- NaCl). (D) Deletion of the ssrS gene affects sspA gene expression levels under hyperosmotic conditions, as measured by real time qRT-PCR; relative changes of sspA mRNA levels in wt, ΔssrS and ΔssrS Ω cells in the presence relative to absence of 250 mM NaCl; cells were grown under microaerobic conditions for 2.5 h; sspA mRNA levels were normalized to those of the rpoZ (RNA polymerase ω subunit) gene under the respective condition. Note that a 2-ΔΔCT value of 1 would correspond to equal sspA mRNA levels at 250 and 0 mM NaCl; thus, sspA mRNA levels in the wt are even slightly decreased at 250 mM NaCl. Each experiment was based on 3 independent biologic replicates (each technical duplicates). Error bars indicate standard deviations. P values (unpaired t test) were 0.0093 for the ΔssrS and 0.002 for the ΔssrS Ω strain in comparison with the wild-type (wt).
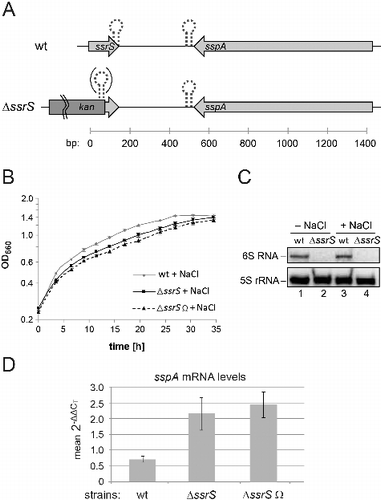
It was reported that upon entry into stationary phase bacteria become more resistant to environmental stresses including oxidative stress (e.g.,Citation28). We therefore tested the role of the 6S RNA in resistance against hydrogen peroxide, paraquat, methylene blue (MB) and tertiary butyl alcohol (tBOOH). Paraquat leads to the formation of superoxide radicals, methylene blue acts as a photosensitizer and generates singlet oxygen in the presence of light; tBOOH represents organic hydroperoxides which are generated during the reaction of singlet oxygen with cellular components. We did not observe a substantial difference in the accumulation of intracellular reactive oxygen species (ROS) for wild-type versus ΔssrS bacteria, at least under microaerobic conditions (Fig. S7A), nor in the sensitivity to H2O2, MB or paraquat (Fig. S7B). We saw a trend toward increased sensitivity of the ΔssrS strain to tBOOH treatment (Fig. S7B), but not in all sets of experiments (data not shown). Thus, we do not consider this to represent a robust effect. Also, there is no evidence for a defect in the formation of photosynthetic complexes in R. sphaeroides upon deletion of 6S RNA (Fig. S7C).
Growth analysis under salt stress (250 mM NaCl, microaerobic conditions) revealed a reduced growth rate for the ΔssrS strain relative to the parental wild-type strain (). 6S RNA levels in wild-type bacteria remained unchanged in the absence or presence of 250 mM NaCl (). We took into consideration that insertion of the terminator-less kanamycin cassette in the ΔssrS strain might have affected expression of the downstream gene sspA encoding a putative salt stress-induced membrane protein. As a consequence, the phenotype might have been due to transcriptional interference rather than ssrS deletion. However, the retardation in growth rate was also manifest for the ΔssrS Ω strain (), whose kanamycin cassette contains a transcription terminator () to prevent such transcriptional interference. We then considered the possibility that the salt stress phenotype of the ΔssrS and ΔssrS Ω strains may be related to 6S RNA-mediated changes in expression of sspA. We thus performed real time qRT-PCR experiments to quantify the levels of sspA mRNA in the 6S RNA knockouts. Both, the ΔssrS and the ΔssrS Ω strains, showed increased levels of sspA mRNA under high salt conditions (), indicating that the 6S RNA gene or 6S RNA itself is responsible for attenuated expression of sspA under salt stress conditions in the wild-type strain.
Discussion
In the α-proteobacterium R. sphaeroides, highest 6S RNA levels were observed at the transition from exponential to stationary phase, followed by a strong decrease during prolonged stationary phase. In the γ-proteobacterium E. coli, 6S RNA levels were shown to steadily increase from exponential to stationary phase.Citation2 A similar expression pattern was described for 6S RNA from Legionella pneumophila, another member of the γ-proteobacteria.Citation29 6S RNA from the ϵ-proteobacterium Helicobacter pylori increased from early to mid-exponential phase and remained constant up to late stationary phase.Citation26 In the cyanobacterium Synechococcus sp. strain PCC6301, 6S RNA levels decreased in stationary phase,Citation30 indicative of a 6S RNA function in actively dividing cells. In Prochlorococcus MED4, another phototrophic cyanobacterium, 6S RNA levels are rather constant from exponential into stationary phase. However, upon cell cycle synchronization by light–dark cycles, 6S RNA levels were observed to decrease during night periods.Citation31 Within rhizobia (α-proteobacteria), agriculturally important soil bacteria capable of nitrogen fixation in symbiosis with plants, 6S RNA levels were found to increase only 1.4-fold from exponential to stationary phase in Sinorhizobium meliloti and to remain essentially constant in the distantly related Bradyrhizobium japonicum; yet, 6S RNA levels were found to be elevated relative to free-living cells when B. japonicum formed bacteroids in root nodules.Citation32,Citation33 6S-1 RNA of B. subtilis reaches highest abundance just before entry into stationary phase, after which its levels remain high, similar to E. coli 6S RNA.Citation16 However, B. subtilis 6S-2 RNA was found to be most abundant in early to mid-exponential phase and to decrease toward extended stationary phase.Citation16 In conclusion, R. sphaeroides has evolved a specific expression pattern for its 6S RNA, with cellular levels peaking at the transition to stationary phase followed by a strong decrease during prolonged stationary phase. The large decrease in the level of R. sphaerodies 6S RNA during stationary phase removes an inhibitory regulator of RNAP and thereby contributes to an altered transcription profile in stationary vs. exponential growth phase. The drop of 6S RNA levels in stationary phase is a feature shared with B. subtilis 6S-2 RNA and Synechococcus 6S RNA.
We further analyzed the levels of pRNA transcripts synthesized by RNAP holoenzymes on 6S RNA as template.Citation10 According to our Northern blot analyses, the steady-state levels of longer pRNAs (≥ 12 nt) transcribed from R. sphaeroides 6S RNA are highest in mid-to-late exponential phase and largely decrease toward prolonged stationary phase (). This trend is basically also seen in the pRNA-Seq data (see Results section and ); pRNAs ≥ 12 nt are a major pRNA length fraction during mid-to-late exponential phase (∼ 0.5 OD660; time point 1 in and ) and, based on studies of B. subtilis 6S-1 RNA,Citation17,Citation34 are assumed to be long enough to remain bound to 6S RNA and to rearrange its structure and trigger RNAP release. We thus predict that, during exponential growth, many RNAP molecules undergo sequestration by 6S RNA as well as release from the RNA via pRNA synthesis. The released 6S RNAs are assumed to remain largely inactive owing to only slow dissociation of bound pRNAs ≥ 12 nt (note that a hybrid helix of 6S RNA and a pRNA 12-mer involves 8 G:C base pairs in R. sphaeroides; see also Citation34). These 6S RNA:pRNA hybrids may then be degraded by RNases due to the loss of protection by RNAP, as suggested for E. coli 6S and B. subtilis 6S-1 RNAs.Citation17,Citation35 The transient sequestration of a substantial fraction of RNAP molecules by 6S RNA during exponential growth may tighten the competition between weak and strong DNA promoters in favor of the latter, and might also down-regulate spurious transcription (transcriptional noise). If 6S RNA binds specifically to the housekeeping RNAP holoenzyme (σ93-RNAP in R. sphaeroides;Citation36) as in E. coli or B. subtilis,Citation6 remains to be seen. High pRNA levels during exponential growth phase is a feature that distinguishes R. sphaeroides 6S RNA from B. subtilis 6S-2 RNA for which pRNA levels are hard to detect at all,Citation16,Citation18,Citation19 although 6S-2 RNA accumulates to substantial levels as well during exponential growth.Citation16
Knowledge is still fragmentary how the diverse expression patterns of 6S RNAs, as well as their structural and mechanistic idiosyncrasies, relate to their biologic functions in different organisms. E. coli cells lacking 6S RNA are at a disadvantage for survival during extended stationary phase but better survive at high pH,,Citation3,Citation37 and a 6S RNA knockout was further shown to affect the level of ppGpp,Citation38 supporting a role in general stress adaptation (reviewed inCitation10). In B. subtilis, deletion of both 6S RNA genes caused an accelerated decrease of optical density toward extended stationary phase and a faster outgrowth from stationary phase under extreme alkaline stress conditions (pH 9.8Citation39). Several proteins deregulated in single or double 6S RNA knockouts of B. subtilis are involved in metabolism and stress responses.Citation39 Lack of 6S-1 RNA, considered to be the functional homolog of E. coli 6S RNA,Citation10 caused a delay in outgrowth of cells from stationary phase and resulted in an earlier onset of sporulation, which was attributed to faster exhaustion of nutrients.Citation19,Citation40 Based on the aforementioned evidences for 6S RNA roles in the regulation of stress responses, we particularly looked into the sensitivity of the R. spaeroides ΔssrS strain to photooxidative stress. However, we did not observe a generally increased sensitivity of the ΔssrS strain to ROS-inducing chemicals, with the possible exception of tBOOH (Fig. S7). As the tBOOH effect was not robust in all experimental repetitions, it is a possibility that the 6S RNA deletion may increase tBOOH sensitivity in a fraction of cells, and the size of this fraction (and thus the detectability of the phenotype) may vary owing to a certain transcriptomal instability of the ΔssrS strain relative to the parental wild-type strain.
During our searches for phenotypes of the R. sphaeroides ΔssrS deletion strains, we detected the sensitivity to high salt stress. At 250 mM NaCl, growth of the mutant strains was retarded, which correlated with elevated sspA mRNA levels (). Complementation of the ΔssrS strain with a plasmid expressing R. sphaeroides 6S RNA from its native promoter (resulting in ∼ 1.3-fold higher 6S RNA expression compared with the wild type; Fig. S8A) did not rescue growth of the ΔssrS strain under high salt stress (Fig. S8B). Considering the spatial vicinity of ssrS and sspA genes, this finding may imply the following mechanistic scenario: as the local concentration of 6S RNA will be particularly high at its site of transcription, newly transcribed 6S RNAs may bind with high frequency to RNAP enzymes in the vicinity of the ssrS locus (including those by which they were synthesized), and this local RNAP sequestration may lower the rate of transcripion at the nearby sspA promoter. This would be consistent with a role of 6S RNA in dampening transcription of certain genes to avoid their overshooting expression, as observed before in the E. coli and B. subtilis model systems.Citation37,Citation39,Citation40 An alternative explanation for our findings, not excludable at present, might be that the ssrS deletion exerts its effect on the DNA level by somehow affecting DNA:protein interaction or DNA accessibility/superhelicity in this genomic region under high salt stress, such that the rate of sspA transcription and cell growth is altered.
Material and methods
Bacterial strains and growth conditions
R. sphaeroides strains were cultivated in minimal-salt malate mediumCitation41 or on corresponding solid medium containing 1.6% (w/v) agar at 32°C in the dark (except for phototrophic growth). Aerobic growth conditions (160–180 μM of dissolved oxygen) were adjusted by using Erlenmeyer flasks with extra deep baffles filled with culture up to 30% of the maximum volume measuring scale and shaking at 140 rpm (GFL Orbital Shaker 3017). For microaerobic growth (about 25 μM of dissolved oxygen) standard Erlenmeyer flasks without baffles were filled up to 80% of the maximum volume measuring scale; shaking was performed at 140 rpm. For phototrophic growth, cells were cultured without agitation in completely filled screw-cap Meplat bottles which were illuminated with white light at 60 Wm−2. E. coli strains were grown in Luria–Bertani medium at 37°C with shaking (180 rpm) or on solid growth medium containing 1.6% (w/v) agar. When required, kanamycin (25 μg/ml) was added to liquid or solid growth media. Addition of kanamycin was avoided in cultures and agar plates used for inhibition zone assays to ensure identical growth conditions. To induce a high salt stress response, a sterile NaCl stock solution was added (final concentration: 250 mM) to liquid cultures at an OD660 of 0.2.
RNA isolation and quality assignment
As the standard procedure, R. sphaeroides was grown in triplicate cultures inoculated from single colonies. At time points of interest, withdrawn cell samples were rapidly chilled on ice and harvested by centrifugation (8,000 to 10,000 x g, 4°C). For the analysis of 6S RNA, 5S rRNA and SorY RNA levels, total RNA was isolated by the hot phenol method, followed by chloroform/isoamyl alcohol treatment and precipitation with sodium acetate and ethanol.Citation42 For quantitative real-time RT-PCR (qRT-PCR) and detection of pRNAs, total RNA was isolated using the TRIzol® reagent (Thermo Scientific Invitrogen) as described by the manufacturer. For qRT-PCR, total RNA was further treated with DNase I (Thermo Scientific Invitrogen) to remove contaminating DNA. After DNA digestion, RNA was purified by standard procedures using a mixture of phenol/chloroform/isoamyl alcohol (25:24:1 (v/v)). After ethanol precipitation, air-dried RNA pellets were dissolved in RNase-free water (Roth) and RNA concentrations were determined using a NanoDrop 1000 Spectrophotometer (Peqlab). For qRT-PCR, the absence of genomic DNA contaminations was confirmed by PCR using primers targeting the gloB gene (RSP_0799) as described previously.Citation43 For all types of experiments, the quality of extracted total RNAs was also checked by denaturing (7 M urea) PAGE.
RNA-Seq experiments
To analyze pRNA and 6S RNA expression in R. sphaeroides at single nucleotide resolution throughout different growth phases we freshly inoculated 400 ml of medium in 500 ml Erlenmeyer flasks in biologic triplicates and grew the cells as described above for the microaerobic conditions. Samples for RNA isolation by the hot phenol method were taken in exponential phase (OD660 0.5−0.6), at the transition to stationary phase (OD660 0.9−1.2), after 12 h of stationary phase (OD660 1.7−2.0) and during outgrowth (20 min after dilution of stationary cultures in fresh medium to an OD660 of 0.2). Half of each DNA-free RNA sample was treated with TEX (Epicentre #TER51020) to enrich for primary transcripts.Citation26 Illumina cDNA libraries were prepared by vertis Biotechnology AG, Germany (http://www.vertis-biotech.com/) as described before without prior RNA fragmentation or size fractionation;Citation41 cDNA libraries were sequenced on a HiSeq 2000 machine in the single-read mode with 100 cycles. Raw data files have been deposited at the National Center for Biotechnology Information Gene Expression Omnibus (GEO)Citation44 and are accessible via the GEO accession GSE71844 (http://www.ncbi.nlm.nih.gov/geo/query/acc.cgi?acc = GSE71844).
Bioinformatic data analysis
Reads were trimmed using the FASTX Toolkit v0.0.14 (http://hannonlab.cshl.edu/fastx_toolkit/) at a quality threshold of 20. Poly(A)-tails were removed by truncating trailing A residues; reads were also truncated at internal sequence stretches of ≥ 5 consecutive A residues. Reads smaller than 8 nt were discarded. Mapping of cDNA reads to the R. sphaeroides 2.4.1 genome (NC_007488, NC_007490, NC_007494, NC_009008, NC_007489, NC_007493, NC_009007) was done with segemehl v0.2.0Citation45 with an e-value of 0.1 and 90% accuracy. For pRNA reads, we applied a separate mapping step with perfect matching. Visualization was done using the Integrated Genome Browser.Citation46
Northern blot analysis
After hot phenol extraction, total RNA (1–10 μg per sample) was separated by denaturing (7 M urea) 10% PAGE, followed by RNA transfer onto Biodyne B 0.45 mm Nylon Transfer membranes (Pall) by semi-dry electroblotting (Novex, Thermo Scientific). Synthetic oligodeoxynucleotides used as probes for the detection of 6S, 5S and SorY RNAs are listed in Table S2. After standard 5′-[32P]-endlabeling using T4 polynucleotide kinase (Thermo Scientific) and [γ-32P]ATP (Hartmann Analytik), 2–6 pmol of the labeled DNA probe (0.5– 1.3 × 106 Cherenkov counts) were used for the hybridization in low stringency Church bufferCitation47 at 42°C overnight. Hybridized membranes were washed twice (30 s each time) with 5 x SSC (Saline-Sodium Citrate) buffer containing 0.01% (w/v) SDS to remove unspecifically bound probe. For reuse in hybridization experiments with a second probe, membranes were stripped at 95°C in 0.1% SDS solution for 10–20 min to remove the first probe. After exposure of phosphoimaging screens (Bio-Rad), images were analyzed by the 1D-Quantity One software (Bio-Rad). The intensity of the 6S RNA signal was normalized to that of the corresponding 5S rRNA signal. Mean values of normalized 6S RNA intensities derived from independent experiments (with error bars indicating standard deviations) were calculated and finally normalized to the mean value of the sample with the lowest 6S RNA level which was set to 1, yielding the plotted values of “relative 6S RNA level." Detection of pRNAs in total RNA extracts was performed by using a Northern blot technique with increased sensitivity involving EDC-crosslinking and digoxigenin-labeled mixed DNA/LNA probes as described.Citation48,Citation49
Construction of R. sphaeroides ssrS deletion strains
Deletion strains were generated via pPHU281-derived suicide plasmids (Table S1) and screening for insertion of a kanamycin resistance cassette into the chromosome by homologous recombination. Briefly, upstream and downstream sequences of the ssrS gene were amplified by PCR using the primer pairs 5′PstI_ssrSup/3′BamHI_ssrSup and 5′BamHI_ssrSdown/3′EcoRI_ssrSdown (Table S2). Resulting PCR products were digested with the corresponding restriction enzymes. Final DNA fragments, 442 bp (upstream) and 563 bp (downstream) in length, were ligated simultaneously into the PstI and EcoRI sites of linearized pPHU281.Citation50 The resulting plasmid pPHUssrS was linearized with BamHI to allow insertion of a kanamycin resistance cassette excised from pUC4K or pHP45ΩKm by BamHI cleavage. The generated suicide plasmids pPHUssrS::Km and pPHUssrS::ΩKm were sequenced to ensure the absence of any mutations in the inserted regions. After transformation of E. coli S17–1, the suicide plasmids were transferred to R. sphaeroides 2.4.1 by diparental conjugation. Conjugants were selected on malate minimal salt agar plates containing kanamycin. Chromosomal insertion was verified by PCR and absence of 6S RNA on Northern blots. In the resulting deletion strains R. sphaeroides 2.4.1ΔssrS and ΔssrS Ω, positions +1 to +125 of the 6S gene (ssrS) are replaced with the inserted kanamycin resistance cassette (including a transcription terminator in the case of strain ΔssrS Ω).
Quantitative real-time PCR
The One-Step Brilliant III qRT-PCR Master Mix Kit (Agilent) was used for reverse transcription followed by PCR as described in the manufacturer's manual. RT-PCR samples containing 20 ng/μl of total RNA were run in a c1000 thermal real-time PCR cycler (Bio-Rad) for relative quantification of mRNAs in 2 technical replicate experiments. The primer sequences used for amplification are listed in Table S2. 2-ΔΔCT values in were based on 3 biological replicates (each based on 2 technical replicates) using the housekeeping gene rpoZ encoding the omega subunit of RNA polymerase for normalization; the mean values of the technical replicates were used to calculate the mean 2-ΔΔCT values and their standard deviations. In detail, we first calculated ΔCT (sspA - rpoZ) in the absence vs. presence of NaCl for the wild-type and ΔssrS strains, followed by calculating ΔΔCT (ΔCT values at 250 mM NaCl minus those at 0 mM NaCl) and the resulting mean 2-ΔΔCT ± SD values. The real-time PCR efficiencies were between 2.02 and 2.05 for sspA and rpoZ.
Inhibition zone assays
Measurements of R. sphaeroides sensitivity to reactive oxygen species (ROS) or reagents leading to ROS formation were performed as described earlier.Citation51 Cells were grown under microaerobic conditions to an OD660 of 0.4–0.8. Then, a part of the culture was diluted in fresh medium to an OD660 of 0.1 and 100 μl of the dilution was added to 6 ml of warm (∼42°C) top agar medium (minimal-salt malate medium containing 0.8% (w/v) agar). Then the mixture was poured onto top of an agar plate containing 1.6% (w/v) agar in minimal-salt malate medium. Filter paper disks soaked with 5 μl of 10 mM methylene blue (MB), 500 mM N,N′-dimethyl-4,4′-bipyridinium dichloride (paraquat), 700 mM tert-butyl hydroperoxide (tBOOH) or 2.5 M hydroperoxide (H2O2) were placed on top of the plates. The diameter of the inhibition zones was measured after incubation for 60–62 h at 32°C in the dark or with illumination by a fluorescent tube (model NL 36 W/860 daylight; Spectralux Plus, Radium, Wipperfuerth, Germany) in the case of MB.
Intracellular ROS detection assay
Accumulation of intracellular ROS was measured using an oxidation-sensitive fluorescent probe – 2,7-dihydrodichlorofluorescein diacetate (DCFH-DA; Thermo Scientific) – which reacts with ROS and forms highly fluorescent dichlorofluorescein. 95 µl of an exponentially grown culture (OD660 = 0.4–0.5, microaerobic conditions) were withdrawn and transferred to a 96-well plate followed by addition of 5 µl 200 mM DCFH-DA (final concentration of 10 mM) and incubation for 1 h at 32°C in the dark. The fluorescence intensities were measured at λ = 492 nm (excitation) and λ = 525 nm (emission) in a microplate reader (Infiniti M200, Tecan).
Disclosure of potential conflicts of interest
No potential conflicts of interest were disclosed.
Supplemental_Material.docx
Download MS Word (2.7 MB)Acknowledgment
We like to thank Falk Schulte for helpful discussions on qRT-PCR analyses and Katrin Müller for valuable support during the revision process.
Funding
This project was funded by the Deutsche Forschungsgemeinschaft (IRTG 1384) and the and the Russian Scientific Foundation under grant N 14–24–00061 (to D. E., O B. and E. K; and Fig. S2, S3).
References
- Hindley J. Fractionation of 32P-labelled ribonucleic acids on polyacrylamide gels and their characterization by fingerprinting. J Mol Biol. 1967;30(1):125–36. doi:10.1016/0022-2836(67)90248-3.
- Wassarman KM, Storz G. 6S RNA regulates E. coli RNA polymerase activity. Cell. 2000;101(6):613–23. doi:10.1016/S0092-8674(00)80873-9.
- Trotochaud AE, Wassarman KM. 6S RNA function enhances long-term cell survival. J Bacteriol. 2004;186(15):4978–85. doi:10.1128/JB.186.15.4978-4985.2004.
- Cavanagh AT, Klocko AD, Liu X, Wassarmann KM. Promoter specificity for 6S RNA regulation of transcription is determined by core promoter sequences and competition for region 4.2 of σ70. Mol Microbiol. 2008;67(6):1242–56. doi:10.1111/j.1365-2958.2008.06117.x.
- Neusser T, Polen T, Geissen R, Wagner R. Depletion of the non-coding regulatory 6S RNA in E. coli causes a surprising reduction in the expression of the translation machinery. BMC Genomics. 2010;11:165. doi:10.1186/1471-2164-11-165.
- Trotochaud AE, Wassarman KM. A highly conserved 6S RNA structure is required for regulation of transcription. Nat Struct Mol Biol. 2005;12(4):313–9. doi:10.1038/nsmb917.
- Barrick JE, Sudarsan N, Weinberg Z, Ruzzo WL, Breaker RR. 6S RNA is a widespread regulator of eubacterial RNA polymerase that resembles an open promoter. RNA. 2005;11:774–84. doi:10.1261/rna.7286705.
- Willkomm DK, Hartmann RK. 6S RNA - an ancient regulator of bacterial RNA polymerase rediscovered. Biological Chemistry. 2005;386(12):1273–7. doi:10.1515/BC.2005.144.
- Steuten B, Setny P, Zacharias M, Wagner R. Mapping the spatial neighborhood of the regulatory 6S RNA bound to Escherichia coli RNA polymerase holoenzyme. J Mol Biol. 2013;425(19):3649–61. doi:10.1016/j.jmb.2013.07.008.
- Steuten B, Hoch PG, Damm K, Schneider S, Köhler K, Wagner R, Hartmann RK. Regulation of transcription by 6S RNAs: insights from the Escherichia coli and Bacillus subtilis model systems. RNA Biol. 2014;11(5):508–21. doi:10.4161/rna.28827.
- Vogel DW, Hartmann RK, Struck JC, Ulbrich N, Erdmann VA. The sequence of the 6S RNA gene of Pseudomonas aeruginosa. Nucleic Acids Res. 1987;15(11):4583–91. doi:10.1093/nar/15.11.4583.
- Wehner S, Damm K, Hartmann RK, Marz M. Dissemination of 6S RNA among bacteria. RNA Biol. 2014;11(11):1467–78. doi:10.4161/rna.29894.
- Cavanagh AT, Wassarman KM. 6S RNA, a global regulator of transcription in Escherichia coli, Bacillus subtilis, and beyond. Annu Rev Microbiol. 2014;68:45–60. doi:10.1146/annurev-micro-092611-150135.
- Wassarman KM, Saecker RM. Synthesis-mediated release of a small RNA inhibitor of RNA polymerase. Science. 2006;314(5805):1601–3. doi:10.1126/science.1134830.
- Panchapakesan SS, Unrau PJ. E. coli 6S RNA release from RNA polymerase requires sigma70 ejection by scrunching and is orchestrated by a conserved RNA hairpin. RNA. 2012;18(12):2251–9. doi:10.1261/rna.034785.112.
- Beckmann BM, Burenina OY, Hoch PG, Kubareva EA, Sharma CM, Hartmann RK. In vivo and in vitro analysis of 6S RNA-templated short transcripts in Bacillus subtilis. RNA Biology. 2011;8(5):839–49. doi:10.4161/rna.8.5.16151.
- Beckmann BM, Hoch PG, Marz M, Willkomm DK, Salas M, Hartmann RK. A pRNA-induced structural rearrangement triggers 6S-1 RNA release from RNA polymerase in Bacillus subtilis. EMBO J. 2012;31(7):1727–38. doi:10.1038/emboj.2012.23.
- Hoch PG, Schlereth J, Lechner M, Hartmann RK. Bacillus subtilis 6S-2 RNA serves as a template for short transcripts in vivo. RNA. 2016;22(4):614–22. doi:10.1261/rna.055616.115.
- Cavanagh AT, Sperger JM, Wassarman KM. Regulation of 6S RNA by pRNA synthesis is required for efficient recovery from stationary phase in E. coli and B. subtilis. Nucleic Acids Res. 2012;40(5):2234–46. doi:10.1093/nar/gkr1003.
- Cabrera-Ostertag IJ, Cavanagh AT, Wassarmann KM. Initiating nucleotide identity determines efficiency of RNA synthesis from 6S RNA templates in Bacillus subtilis but not Escherichia coli. Nucleic Acids Res. 2013;41(15):7501–11. doi:10.1093/nar/gkt517.
- Berghoff BA, Glaeser J, Sharma CM, Vogel J, Klug G. Photooxidative stress-induced and abundant small RNAs in Rhodobacter sphaeroides. Mol Microbiol. 2009;74(6):1497–512. doi:10.1111/j.1365-2958.2009.06949.x.
- Tsuzuki M1, Xu XY, Sato K, Abo M, Arioka M, Nakajima H, Kitamoto K, Okubo A. SspA, an outer membrane protein, is highly induced under salt-stressed conditions and is essential for growth under salt-stressed aerobic conditions in Rhodobacter sphaeroides f. sp. denitrificans. Appl Microbiol Biotechnol. 2005;68(2):242–50. doi:10.1007/s00253-004-1852-7.
- Nuss AM, Glaeser J, Berghoff BA, Klug G. Overlapping alternative sigma factor regulons in the response to singlet oxygen in Rhodobacter sphaeroides. J Bacteriol. 2010;192(10):2613–23. doi:10.1128/JB.01605-09.
- Zuker M. Mfold web server for nucleic acid folding and hybridization prediction. Nucleic Acids Res. 2003;31(13):3406–15. doi:10.1093/nar/gkg595.
- Adnan F, Weber L, Klug G. The sRNA SorY confers resistance during photooxidative stress by affecting a metabolite transporter in Rhodobacter sphaeroides. RNA Biol. 2015;12(5):569–77. doi:10.1080/15476286.2015.1031948.
- Sharma CM, Hoffmann S, Darfeuille F, Reignier J, Findeiss S, Sittka A, Chabas S, Reiche K, Hackermüller J, Reinhardt R, et al. The primary transcriptome of the major human pathogen Helicobacter pylori. Nature. 2010;464(7286):250–5. doi:10.1038/nature08756.
- Burenina OY, Elkina DA, Hartmann RK, Oretskaya TS, Kubareva EA. Small Noncoding 6S RNAs of bacteria. Biochemistry (Mosc). 2015;80(11):1429–46. doi:10.1134/S0006297915110048.
- Roop RM, 2nd, Gee JM, Robertson GT, Richardson JM, Ng WL, Winkler ME. Brucella stationary-phase gene expression and virulence. Annu Rev Microbiol. 2003;57:57–76. doi:10.1146/annurev.micro.57.030502.090803.
- Faucher SP, Friedlander G, Livny J, Margalit H, Shuman HA. Legionella pneumophila 6S RNA optimizes intracellular multiplication. Proc Natl Acad Sci U S A. 2010;107(16):7533–8. doi:10.1073/pnas.0911764107.
- Watanabe T, Sugiura M, Sugita M. A novel small stable RNA, 6Sa RNA, from the cyanobacterium Synechococcus sp. strain PCC6301. FEBS Letter. 1997;416(3):302–6. doi:10.1016/S0014-5793(97)01237-4.
- Axmann IM, Holtzendorff J, Voss B, Kensche P, Hess WR. Two distinct types of 6S RNA in Prochlorococcus. Gene. 2007;406(1-2):69–78. doi:10.1016/j.gene.2007.06.011.
- Voss B, Hölscher M, Baumgarth B, Kalbfleisch A, Kaya C, Hess WR, Becker A, Evguenieva-Hackenberg E. Expression of small RNAs in Rhizobiales and protection of a small RNA and its degradation products by Hfq in Sinorhizobium meliloti. Biochem Biophys Res Commun. 2009;390(2):331–6. doi:10.1016/j.bbrc.2009.09.125.
- Madhugiri, Pessi G, Voss B, Hahn J, Sharma CM, Reinhardt R, Vogel J, Hess WR, Fischer HM, Evguenieva-Hackenberg E. Small RNAs of the Bradyrhizobium/Rhodopseudomonas lineage and their analysis. RNA Biol. 2012;9(1):47–58. doi:10.4161/rna.9.1.18008.
- Burenina OY, Hoch PG, Damm K, Salas M, Zatsepin TS, Lechner M, Oretskaya TS, Kubareva EA, Hartmann RK. Mechanistic comparison of Bacillus subtilis 6S-1 and 6S-2 RNAs – commonalities and differences. RNA. 2014;20(3):348–59. doi:10.1261/rna.042077.113.
- Wassarman KM. 6S RNA: a regulator of transcription. Mol Microbiol. 2007;65(6):1425–31. doi:10.1111/j.1365-2958.2007.05894.x.
- Karls, RK, Jin DJ, Donohue TJ. Transcription properties of RNA polymerase holoenzymes isolated from the purple nonsulfur bacterium Rhodobacter sphaeroides. J Bacteriol. 1993;175(23):7629–38. doi:10.1128/jb.175.23.7629-7638.1993.
- Trotochaud AE, Wassarman KM. 6S RNA regulation of pspF transcription leads to altered cell survival at high pH. J Bacteriol. 2006;188(11):3936–43. doi:10.1128/JB.00079-06.
- Cavanagh AT, Chandrangsu P, Wassarman KM. 6S RNA regulation of relA alters ppGpp levels in early stationary phase. Microbiology. 2010;156(Pt 12):3791–800. doi:10.1099/mic.0.043992-0.
- Hoch PG, Burenina OY, Weber MH, Elkina DA, Nesterchuk MV, Sergiev PV, Hartmann RK, Kubareva EA. Phenotypic characterization and complementation analysis of Bacillus subtilis 6S RNA single and double deletion mutants. Biochimie. 2015;117:87–99. doi:10.1016/j.biochi.2014.12.019.
- Cavanagh AT, Wassarman KM. 6S-1 RNA function leads to a delay in sporulation in Bacillus subtilis. J Bacteriol. 2013;195(9):2079–86. doi:10.1128/JB.00050-13.
- Remes B, Berghoff BA, Forstner KU, Klug G. Role of oxygen and the OxyR protein in the response to iron limitation in Rhodobacter sphaeroides. BMC Genomics. 2014;15:794. doi:10.1186/1471-2164-15-794.
- Janzon L, Löfdahl S, Arvidson S. Evidence for a coordinate transcriptional control of alpha-toxin and protein a synthesis in Staphylococcus aureus. Restricted Access FEMS Microbiol Lett. 1986;33(2-3):193–8. doi:10.1111/j.1574-6968.1986.tb01270.x.
- Glaeser J, Klug G. Photo-oxidative stress in Rhodobacter sphaeroides: protective role of carotenoids and expression of selected genes. Microbiology. 2005;151(Pt6):1927–38. doi:10.1099/mic.0.27789-0.
- Edgar R, Domrachev M, Lash AE. Gene Expression Omnibus: NCBI gene expression and hybridization array data repository. Nucleic Acids Res. 2002;30(1):207–10. doi:10.1093/nar/30.1.207.
- Hoffmann S, Otto C, Kurtz S, Sharma CM, Khaitovich P, Vogel J, Stadler PF, Hackermueller J. Fast mapping of short sequences with mismatches, insertions and deletions using index structures. PLoS Comput Biol. 2009;5(9):e1000502. doi:10.1371/journal.pcbi.1000502.
- Nicol JW, Helt GA, Blanchard SG, Jr, Raja A, Loraine AE. The Integrated Genome Browser: free software for distribution and exploration of genome-scale datasets. Bioinformatics. 2009;25(20):2730–1. doi:10.1093/bioinformatics/btp472.
- Church GM, Gilbert W. Genomic sequencing. Proc Natl Acad Sci U S A. 1984;81(7):1991–5. doi:10.1073/pnas.81.7.1991.
- Beckmann BM, Grünweller A, Weber MH, Hartmann RK. Northern blot detection of endogenous small RNAs (approximately 14 nt) in bacterial total RNA extracts. Nucleic Acids Res. 2010;38(14):e147. doi:10.1093/nar/gkq437.
- Damm K, Bach S, Müller KM, Klug G, Burenina OY, Kubareva EA, Grünweller A, Hartmann RK. Improved Northern blot detection of small RNAs using EDC crosslinking and DNA/LNA probes. Methods Mol Biol. 2015;1296:41–51. doi:10.1007/978-1-4939-2547-6_5.
- Hübner P, Willison JC, Vignais PM, Bickle TA. Expression of regulatory nif gnes in Rhodobacter capsulatus. J Bacteriol. 1991;173(9):2993–9. doi:10.1128/jb.173.9.2993-2999.1991.
- Nuss AM, Glaeser J, Klug G. RpoH(II) activates oxidative-stress defense systems and is controlled by RpoE in the singlet oxygen-dependent response in Rhodobacter sphaeroides. J Bacteriol. 2009;191(1):220–30. doi:10.1128/JB.00925-08.