ABSTRACT
The MnmE-MnmG complex of Escherichia coli uses either ammonium or glycine as a substrate to incorporate the 5-aminomethyl or 5-carboxymethylaminomethyl group into the wobble uridine of certain tRNAs. Both modifications can be converted into a 5-methylaminomethyl group by the independent oxidoreductase and methyltransferase activities of MnmC, which respectively reside in the MnmC(o) and MnmC(m) domains of this bifunctional enzyme. MnmE and MnmG, but not MnmC, are evolutionarily conserved. Bacillus subtilis lacks genes encoding MnmC(o) and/or MnmC(m) homologs. The glycine pathway has been considered predominant in this typical gram-positive species because only the 5-carboxymethylaminomethyl group has been detected in tRNALysUUU and bulk tRNA to date. Here, we show that the 5-methylaminomethyl modification is prevalent in B. subtilis tRNAGlnUUG and tRNAGluUUC. Our data indicate that B. subtilis has evolved MnmC(o)- and MnmC(m)-like activities that reside in non MnmC homologous protein(s), which suggests that both activities provide some sort of biological advantage.
Introduction
Transfer RNAs (tRNAs) are by far the most extensively modified RNAs [Citation1,Citation2]. Modifications are post-transcriptionally introduced at precise positions by specific enzymes, and play important roles in folding, stability, identity, and translational and signaling functions of tRNAs [Citation2,Citation3]. In particular, modified nucleosides of the anticodon loop transform the loop architecture and dynamics to meet the requirements that the ribosome places on all tRNAs [Citation2,Citation4,Citation5]. In Escherichia coli, the MnmEG complex, formed by the dimeric proteins MnmE and MnmG [Citation6], modifies the wobble uridine (U34) of tRNALysUUU, tRNAGluUUC, tRNAGlnUUG, tRNALeuUAA, tRNAArgUCU, and tRNAGlyUCC [Citation7,Citation8]. MnmEG catalyzes the incorporation of either an aminomethyl (nm) or a carboxymethylaminomethyl (cmnm) group at position 5 of U34 using ammonium or glycine as substrate () [Citation7]. The MmEG products can be converted into a 5-methylaminomethyl (mnm5) group through the action of the two-domain, bifunctional enzyme MnmC [Citation9]. The oxidoreductase activity of its C-terminal domain, MnmC(o), transforms cmnm5 into nm5 via an FAD-dependent deacetylation, while the methyltransferase activity of the N-terminal domain, MnmC(m), converts nm5 into mnm5 via a SAM-dependent methylation (). However, tRNAGlnUUG and tRNALeuUAA are not substrates for the MnmC(o) domain of MnmC, whereas MnmEG appears to be inefficient in modifying both tRNAs in vivo through the ammonium-dependent reaction [Citation9]. Both features explain why cmnm5 is prevalent in tRNAGlnUUG and tRNALeuUAA. Notably, some tRNA substrates of MnmEG are also substrates of MnmA and TrmL (). MnmA introduces the 2-thiol group into U34 of tRNAGluUUC, tRNALysUUU and tRNAGlnUUG [Citation10], whereas TrmL methylates the 2´-OH group of the U-ribose in tRNALeuUAA [Citation11]. Consequently, the final modifications in U34 are mnm5s2U in tRNALysUUU and tRNAGluUUC, cmnm5s2U and, to a much lesser extent, mnm5s2U in tRNAGlnUUG, and cmnm5Um in tRNALeuUAA [Citation9].
Figure 1. The MnmEG pathways in E. coli.
The MnmEG complex uses the glycine and ammonium pathways to synthesize cmnm5s2U and nm5s2U, respectively. The MnmC(o) and MnmC(m) activities of MnmC transform cmnm5s2U into nm5s2U and nm5s2U into mnm5s2U, respectively. Notably, cmnm5s2U is the prevalent modification in tRNAGlnUUG, as this tRNA is not a substrate for MnmC(o), whereas mnm5s2U is prevalent in tRNALysUUU and tRNAGluUUC.
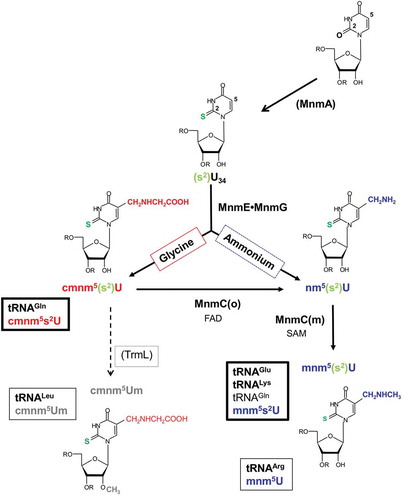
The feasibility of the MnmEG pathways (the glycine and ammonium pathways) not only depends on the tRNA species, but also on the growth conditions [Citation9]. The use of a null mnmC mutant growing in a relatively rich medium revealed that the product of the glycine pathway, cmnm5s2U, was the prevailing modification in bulk tRNA and tRNALysUUU in the exponential phase, whereas the product of the ammonium pathway, nm5s2U, was the overriding modification in both tRNAs as the culture entered the stationary phase [Citation9]. In contrast, cmnm5s2U was the predominant modification at the wobble position of tRNAGlnUUG in all phases of growth. Notably, the ammonium pathway was inefficient when E. coli cells were grown in minimal medium [Citation9]. Altogether these data support the proposal that the performance of each MnmEG pathway depends on both environmental conditions and features of the tRNA molecule [Citation9].
MnmE and MnmG, but not MnmC, are conserved evolutionarily from bacteria to humans. In fact, MnmE and MnmG have been included in the minimal set of proteins required for a functional translation apparatus in bacteria [Citation12]. By contrast, putative orthologs of the E. coli bi-functional MnmC protein are conserved only in γ-proteobacteria and a few members of other bacterial classes, although orthologs of a single domain have been identified in several genomes [Citation13]. This observation, together with the ability of the E. coli MnmC(o) and MnmC(m) domains to function independently of one another, suggests that the origin of the full MnmC protein present in γ-Proteobacteria likely occurred by domain fusion [Citation9].
Very little information is available on the use of the MnmEG pathways in bacteria other than E. coli. The ammonium pathway has been proposed to be the dominant route in Aquifex aeolicus because this species has a homolog of MnmC(m) but not of MnmC(o), and mnm5s2U, but not cmnm5s2U, has been HPLC-detected in bulk A. aeolicus tRNA hydrolysates [Citation14]. Conversely, the glycine pathway has been considered the predominant route in Bacillus subtilis and Mycoplasma capricolum given that the genomes of both species lack the genes encoding the bifunctional MnmC protein or a monofunctional MnmC(m), and only cmnm5(s2)U(m)-type derivatives have been detected in certain MnmEG-substrate tRNAs (e.g., B. subtilis tRNALysUUU) or bulk tRNA to date [Citation14–Citation19]. Curiously back in the mid-1970s, Vold and colleagues reported the probable presence of mnm5s2U in B. subtilis tRNA [Citation20,Citation21] but they did not find evidence for this nucleoside in a subsequent study [Citation16].
The initial purpose of this work was to explore the performance of the glycine and ammonium pathways of MnmEG in B. subtilis. Unexpectedly, we found that mnm5s2U is present in bulk tRNA purified from a B. subtilis wild-type strain, but not from a null mnmG mutant, which clearly reveals both the activity of MnmEG and the subsequent activity of an MnmC(m)-like enzyme. Our data demonstrate that B. subtilis extracts contain MnmC(o)- and MnmC(m)-like activities that catalyze in vitro the reactions cmnm5s2U→nm5s2U→mnm5s2U on E. coli tRNAs.
Results and discussion
In order to assess whether B. subtilis bulk tRNA has a similar cmnm5s2U/nm5s2U profile to that found in E. coli mnmC null mutants, tRNA hydrolysates from overnight-grown cultures of B. subtilis wild-type (wt) and ΔmnmG strains were HPLC analyzed and compared with data from E. coli wt and ΔmnmC strains (). Strikingly, apart from the expected cmnm5s2U nucleoside, we detected mnm5s2U, but not nm5s2U, in the B. subtilis tRNA hydrolysate ()). Formation of mnm5s2U and cmnm5s2U in the B. subtilis wt strain was MnmEG-dependent because both nucleosides were absent in tRNA purified from a ΔmnmG strain, which exhibited accumulation of s2U ()), the product of MnmA (see ). The finding of mnm5s2U in the wt hydrolysate indicates that an MnmC(m)-like activity is present in B. subtilis. Nucleoside mnm5s2 may derive from nm5s2U synthesized by MnmEG via the ammonium pathway, and/or from cmnm5s2U formed through the glycine pathway. In this case, however, the formation of mnm5s2U would require the participation of both MnmC(o)-like and MnmC(m)-like activities (see ).
Figure 2. Bacillus subtilis tRNA contains both cmnm5s2U and mnm5s2U.
HPLC analysis of total tRNA from E. coli wild-type (A), and E. coli ΔmnmC strains (B), B. subtilis wild-type (C), B. subtilis ∆mnmG (D). Absorbance was monitored at 314 nm to maximize the detection of thiolated nucleosides. Positions of relevant nucleosides are indicated. The identities of selected nucleosides were established by their ultraviolet adsorption spectra (E) and relative retention times in comparison with peaks of synthetic markers. The arrows with asterisks indicate the positions where some relevant nucleosides should migrate although they were undetectable on the corresponding chromatogram.
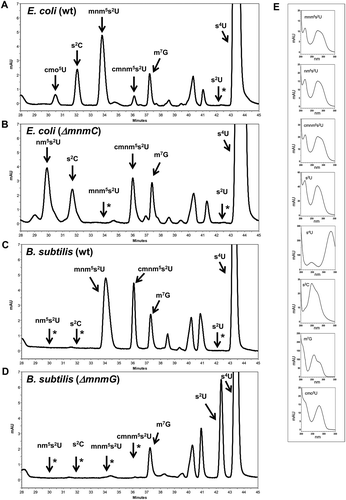
It is noteworthy that, as stated for E. coli, the MnmEG function is relevant for B. subtilis growth because the B. subtilis mnmE- and mnmG-knockout strains grew more slowly than the wt strain (doubling times in LBT: 37.8 ± 1.3, 45.1 ± 1.0 and 44.1 ± 1.3 for the wt, mnmG and mnmE strains, respectively).
A comparison of the mnm5s2U/cmnm5s2U ratio along the growth curve of the B. subtilis wt strain in rich medium (LBT) showed that the proportion of cmnm5s2U (i.e., the product of the glycine pathway) diminished as the culture entered the stationary phase (). The relative reduction in cmnm5s2U is consistent with a previous report indicating that the percentage of labeled cmnm5s2U represented in the total [35S]tRNA purified from B. subtilis grown in rich medium lowered in the stationary phase [Citation16].
Table 1. mnm5s2U/cmnm5s2U ratio in B. subtilis tRNAs along the growth curve.
As we considered that the observed decline in cmnm5s2U was ultimately due to a lower relative ratio of cmnm5s2U-containing tRNA species in the stationary phase, we analyzed the modification profile of native, individual tRNAs presumed to be substrates of MnmEG and MnmA (tRNALysUUU, tRNAGlnUUG, and tRNAGluUUC). In both the exponential and stationary phases, nucleoside cmnm5s2U was the prevalent U34 modification in tRNALysUUU, whereas mnm5s2U was prevalent in tRNAGlnUUG and tRNAGluUUC ( and Supplementary Figure 1). Therefore, B. subtilis tRNALysUUU and tRNAGlnUUG exhibit a U34 modification pattern opposite to that found in their E. coli counterparts (). Our data clearly indicate that: 1) B. subtilis tRNALysUUU is not a substrate of the putative MnmC(o)-like activity and does not use effectively the ammonium pathway under the experimental conditions utilized in this study; 2) tRNAGlnUUG and tRNAGluUUC are substrates for the MnmC(m)-like activity as nucleoside mnm5s2U accumulates in both tRNAs.
In an attempt to identify a protein with at least MnmC(m)-like activity, we used biocomputational approaches to select candidate proteins (see Materials and Methods), and analyzed the presence of mnm5s2U in bulk tRNA purified from the available B. subtilis mutant strains lacking the corresponding genes (Supplementary Table S1). In addition, we analyzed other B. subtilis mutant strains lacking proteins annotated as (putative) RNA methyltransferases (Supplementary Table S1). Nucleoside mnm5s2U was present in tRNA hydrolysates from all the tested strains, while no s2U accumulation was observed (data not shown). Therefore, none of the selected genes was responsible for the synthesis of mnm5s2U and, specifically, for the MnmC(m)-like activity detected in B. subtilis.
In order to directly analyze whether both MnmC-like activities are present in B. subtilis, we ran in vitro modification reactions using extracts from B. subtilis or E. coli ΔmnmG strains (which contain the putative MnmC-like or MnmC enzymes, respectively, but no MnmEG activity), and bulk tRNA purified from exponentially growing cells of an E. coli ΔmnmC strain (in which modification cmnm5s2U is prevalent). As shown in and Supplementary Figure 2, addition of the B. subtilis extract to the tRNA solution (‘Control’) promoted a decrease in the amount of cmnm5s2U and a concomitant increase in nm5s2U and, to a lesser extent, in mnm5s2U. Therefore, the extract exhibited MnmC(o)- and MnmC(m)-like activities capable of catalyzing the reactions cmnm5s2U→nm5s2U→mnm5s2U using E. coli tRNA as a substrate. Similar proportions of the three nucleosides were obtained when FAD was added to the reaction mix, which suggests that the amount of FAD already present in the B. subtilis extract and/or bound to the MnmC(o)-like protein was appropriate for the efficient transformation of cmnm5s2U into nm5s2U. In contrast, addition of SAM resulted in the disappearance of nm5s2U and a concomitant increase in the mnm5s2U levels, which indicates that the B. subtilis MnmC(m)-like protein requires the addition of SAM to efficiently methylate E. coli tRNA. These results were somewhat different from those obtained with the E. coli extract: most cmnm5s2U in E. coli tRNA (‘Control’) was directly transformed into mnm5s2U when the E. coli extract was added to the reaction mix, as no build-up of nm5s2U was observed in the assays ( and Supplementary Figure 2). These data indicate that the MnmC(m) domain of E. coli MnmC does not require the addition of SAM to catalyze the conversion of nm5s2U into mnm5s2U on E. coli tRNA. Potential explanations for the differences observed when using B. subtilis or E. coli extract are that the B. subtilis MnmC(m)-like enzyme has a low affinity for SAM (and competes poorly with other SAM-dependent enzymes present in the B. subtilis extract) and/or requires higher SAM concentrations to modify heterologous tRNAs. In any case, the in vitro modification reactions clearly indicated that B. subtilis possesses MnmC-like activities.
Table 2. B. subtilis extracts display MnmC(o)- and MnmC(m)-like activities.
To obtain further information about the ability of B. subtilis (Bs) tRNAs to use the ammonium and glycine pathways, and to be recognized by U34 modification enzymes, Bs-tRNALysUUU and Bs-tRNAGlnUUG were separately overexpressed in E. coli strains lacking MnmC(o) and/or MnmC(m) activity. HPLC analysis revealed the simultaneous presence of cmnm5s2U and mnm5s2U or nm5s2U in Bs-tRNALysUUU and Bs-tRNAGlnUUG purified from the ΔmnmC(o) or mnmC-W131stop strains (), which indicates that both Bs-tRNAs can be modified in vivo by the E. coli MnmEG complex through the glycine and ammonium pathways. Therefore, the fact that a particular tRNA, such as Bs-tRNALysUUU, can be effectively modified by the ammonium pathway seems to depend on the biological context (E. coli or B. subtilis), which could include factors like the affinity of a particular MnmEG-tRNA complex to ammonium and the sensitivity of tRNAs containing nm5s2U (instead of cmnm5s2U or mnm5s2U) to endogenous nucleases. Interestingly, the complex formed by the human MnmE and MnmG homologs (named GTPBP3 and MTO1, respectively), which usually incorporates taurine instead of glycine into mitochondrial tRNAs, can use glycine when HeLa cells are grown under taurine-depleted conditions [Citation22]. Moreover, the E. coli MnmEG complex catalyzes the in vitro incorporation of taurine into in vitro synthesized E. coli tRNAGlyUCC, albeit inefficiently [Citation22]. Altogether these data uphold the view that the affinity of MnmEG and its homologs to ammonium, glycine and taurine in the presence of different substrate tRNAs may be a key factor for regulating the modification reaction. In this respect, the conformational dynamics of MnmG could play a relevant role in the interaction with the reaction substrates [Citation23], as conformational dynamics can be crucial in tuning the affinity and specificity of molecular interactions [Citation24].
Table 3. Overexpression of B. subtilis tRNALysUUU and tRNAGlnUUG in E. coli mnmC(o) and mnmC(m) mutant strains.
Our data on the heterologous expression of Bs-tRNAs in E. coli strains () also indicated that the cmnm5s2U formed on Bs-tRNALysUUU through the glycine route (detectable in strains ΔmnmC(o) and mnmC-W131stop) was fully transformed into nm5s2 in the E. coli mnmC(m)-G68D mutant, which reveals that Bs-tRNALysUUU is a good substrate for the E. coli MnmC(o) domain. This result contrasts with the observation that Bs-tRNALysUUU is not a substrate for the B. subtilis MnmC(o)-like enzyme (), and suggests that both oxidoreductases differ in the tRNA recognition mechanism. Notably, Bs-tRNAGlnUUG, unlike Ec-tRNAGlnUUG [Citation9], is a substrate for E. coli MnmC(o) activity but is, apparently, not as good as Bs-tRNALysUUU given that a certain amount of cmnm5s2 on Bs-tRNAGlnUUG remained unprocessed in the E. coli mnmC(m)-G68D strain ().
Although our data indicate that Bs-tRNAs can be modified through the ammonium pathway in E. coli, we found no evidence that the ammonium pathway works in B. subtilis. The study of the functionality of this pathway in vivo will require the prior isolation of a B. subtilis mutant lacking the MnmC(o)-like function.
The finding that B. subtilis has evolved MnmC-like activities suggests that the presence of mnm5s2U instead of cmnm5s2U or nm5s2U in certain tRNAs confers them with some kind of biological advantage. This proposal falls in line with our previous report indicating that the impairment of either of the two activities of E. coli MnmC has a biological cost [Citation9]. The fact that B. subtilis MnmC-like activities reside in non MnmC homologous protein(s) calls for caution in assuming that cmnm5s2U may be the final modification in organisms lacking MnmC homologs. Moreover, the proposal that the ammonium pathway is responsible for the mnm5s2U synthesis in organisms that, like A. aeolicus [Citation14], lack an MnmC(o) homolog, should be re-evaluated.
In brief, we uncover a new example of convergent evolution whereby different enzymes are responsible for catalyzing the same deacetylation and methylation reactions that give rise to nucleoside mnm5 at U34, which in turn highlights the biological importance of this modification. The identification of the B. subtilis proteins with MnmC-like activities in future studies will help to determine their degree of evolutionary conservation, as well as the effectiveness of the glycine and ammonium routes through the construction of suitable mutants.
Materials and methods
Bacterial strains, plasmids, and oligonucleotides
E. coli and B. subtilis 168 strains and plasmids are listed in Supplementary Table S1. The B. subtilis tRNALysUUU and tRNAGlnUUG were cloned in pBSKrna digested with EcoRI and PstI. A list of the oligonucleotides used in this study is provided in Supplementary Table S2.
Bacterial growth and preparation of crude extracts
E. coli and B. subtilis 168 strains were grown at 37ºC in LBT medium (LB broth containing 40 mg/ml thymine) with shaking. Cell growth was monitored by measuring the optical density of the cultures at 600 nm (OD600). To prepare crude extracts, strains were grown in 500 mL of LBT overnight at 37ºC. Cells were harvested, resuspended in 5 mL of buffer containing 50 mM Tris-HCl (pH 7.5), 1 mM EDTA, 5 mM MgCl2, and 2 mM phenylmethanesulfonyl fluoride (PMSF), and sonicated for 5 min in an ice bath. Extracts were clarified by centrifugation at 4ºC, and directly used for in vitro tRNA modification reactions. The protein concentration of the lysate was determined by Bradford assay.
Purification of tRNAs and reverse-phase HPLC analysis of nucleosides
Bulk tRNA was purified as described [Citation11]. Specific true native tRNAs and tRNAs overexpressed from pBSKrna-derived plasmids were purified from bulk tRNA by the Chaplet Column Chromatography method using biotinylated DNA probes immobilized on a HiTrap Streptavidin HP column [Citation9,Citation25]. The probes were complementary to the specific sequence of each tRNA (Supplementary Table S2). Analysis of nucleosides by reverse-phase HPLC was performed as described [Citation11]. The nucleosides were identified according to their UV spectra, relative retention times, and by comparison with appropriate controls, including synthetic markers [Citation7,Citation9,Citation26].
Assays for in vitro nm5s2U and mnm5s2U synthesis
The assay system consisted of 50 mM Tris-HCl (pH 8.0), 50 mM NH4CH3CO2, 5% glycerol, 50 μg bulk tRNA isolated from an exponentially grown E. coli ΔmnmC strain (IC6010), and 50 μL (~ 10 μg) of E. coli or B. subtilis crude extract in a final volume of 200 μL. FAD (0.5 mM) and SAM (0.5 mM) were added when required. No bacterial extract was added to control reactions done in parallel. After incubation for 2.5 h at 37ºC with shaking (750 rpm), tRNA was phenol extracted, ethanol precipitated, degraded to nucleotides with nuclease P1 and, finally, treated with bacterial alkaline phosphatase. The resulting hydrolysate was HPLC analyzed.
Biocomputational approach for searching an MnmC(m)-like protein in B. subtilis
Searching for a potential homolog of MnmC(m) in B. subtilis was done by probabilistic inference methods implemented in HMMER3 [Citation27]. The N-terminal sequence (~ 240 aa) of E. coli MnmC (Uniprot id P77182) was used as a bait to recover bacterial homologous from RefSeq database [Citation28]. A multiple sequence alignment of the MnmC(m) domain with 70 non-redundant sequences was constructed using iterative refinement methods [Citation29]. The probabilistic model was used for an hmmscan search across the 4,175 protein encoding genes contained in the B. subtilis 168 genome (GenBank id NC_000949). The analysis indicated that no true ortholog of the MnmC(m) domain exists in B. subtilis, which was in agreement with a previous report [Citation13]. An additional approach was then performed [Citation30]. Briefly, probabilistic models of other RNA methylases acting on 5ʹ of pyrimidines such as RlmC, RlmD, RlmI, RsmB, RsmD, RsmF, and TrmA were built and used to track amino acid conserved motifs in all B. subtilis ORFs [Citation30]. We selected a list of proteins that iteratively appeared to align against short segments (~ 100 aa in length) of HMM profiles from the 5ʹ-pyrimidine methyltransferases. Thus, we recovered a list of candidates (Supplementary Table 1), all of which, except YbxB, were analyzed for the MnmC-like tRNA modification activity.
Supplemental Material
Download PDF (429.3 KB)Disclosure statement
No potential conflict of interest was reported by the authors.
Supplementary material
Supplemental data for this article can be accessed here.
Additional information
Funding
References
- Boccaletto P, Machnicka MA, Purta E, et al. MODOMICS: a database of RNA modification pathways. 2017 update. Nucleic Acids Res. 2018;46:D303–d7.
- Vare VY, Eruysal ER, Narendran A, et al. Chemical and conformational diversity of modified nucleosides affects tRNA structure and function. Biomolecules. 2017;7:29.
- Nachtergaele S, He C. The emerging biology of RNA post-transcriptional modifications. RNA Biol. 2017;14:156–163.
- Agris PF, Eruysal ER, Narendran A, et al. Celebrating wobble decoding: half a century and still much is new. RNA Biol. 2018;15:537–553.
- Agris PF, Narendran A, Sarachan K, et al. The importance of being modified: the role of RNA modifications in translational fidelity. Enzymes. 2017;41:1–50.
- Yim L, Moukadiri I, Björk GR, et al. Further insights into the tRNA modification process controlled by proteins MnmE and GidA of Escherichia coli. Nucleic Acids Res. 2006;34:5892–5905.
- Moukadiri I, Prado S, Piera J, et al. Evolutionarily conserved proteins MnmE and GidA catalyze the formation of two methyluridine derivatives at tRNA wobble positions. Nucleic Acids Res. 2009;37:7177–7193.
- Armengod ME, Meseguer S, Villarroya M, et al. Modification of the wobble uridine in bacterial and mitochondrial tRNAs reading NNA/NNG triplets of 2-codon boxes. RNA Biol. 2014;11:1495–1507.
- Moukadiri I, Garzón MJ, Björk GR, et al. The output of the tRNA modification pathways controlled by the Escherichia coli MnmEG and MnmC enzymes depends on the growth conditions and the tRNA species. Nucleic Acids Res. 2014;42:2602–2623.
- Ikeuchi Y, Shigi N, Kato J, et al. Mechanistic insights into sulfur relay by multiple sulfur mediators involved in thiouridine biosynthesis at tRNA wobble positions. Mol Cell. 2006;21:97–108.
- Benitez-Paez A, Villarroya M, Douthwaite S, et al. YibK is the 2ʹ-O-methyltransferase TrmL that modifies the wobble nucleotide in Escherichia coli tRNA(Leu) isoacceptors. RNA. 2010;16:2131–2143.
- Grosjean H, Breton M, Sirand-Pugnet P, et al. Predicting the minimal translation apparatus: lessons from the reductive evolution of mollicutes. PLoS Genet. 2014;10:e1004363.
- Bujnicki JM, Oudjama Y, Roovers M, et al. Identification of a bifunctional enzyme MnmC involved in the biosynthesis of a hypermodified uridine in the wobble position of tRNA. RNA. 2004;10:1236–1242.
- Kitamura A, Nishimoto M, Sengoku T, et al. Characterization and structure of the Aquifex aeolicus protein DUF752: a bacterial tRNA-methyltransferase (MnmC2) functioning without the usually fused oxidase domain (MnmC1). J Biol Chem. 2012;287:43950–43960.
- Yamada Y, Murao K, Ishikura H. 5-(carboxymethylaminomethyl)-2-thiouridine, a new modified nucleoside found at the first letter position of the anticodon. Nucleic Acids Res. 1981;9:1933–1939.
- Vold BS, Longmire ME, Keith DE Jr. Thiolation and 2-methylthio- modification of Bacillus subtilis transfer ribonucleic acids. J Bacteriol. 1981;148:869–876.
- Andachi Y, Yamao F, Muto A, et al. Codon recognition patterns as deduced from sequences of the complete set of transfer RNA species in Mycoplasma capricolum. Resemblance to mitochondria. J Mol Biol. 1989;209:37–54.
- Anton BP, Russell SP, Vertrees J, et al. Functional characterization of the YmcB and YqeV tRNA methylthiotransferases of Bacillus subtilis. Nucleic Acids Res. 2010;38:6195–6205.
- Kang BI, Miyauchi K, Matuszewski M, et al. Identification of 2-methylthio cyclic N6-threonylcarbamoyladenosine (ms2ct6A) as a novel RNA modification at position 37 of tRNAs. Nucleic Acids Res. 2017;45:2124–2136.
- Singhal RP, Vold B. Changes in transfer ribonucleic acids of Bacillus subtilis during different growth phases. Nucleic Acids Res. 1976;3:1249–1262.
- Vold B. Modified nucleosides of Bacillus subtilis transfer ribonucleic acids. J Bacteriol. 1976;127:258–267.
- Asano K, Suzuki T, Saito A, et al. Metabolic and chemical regulation of tRNA modification associated with taurine deficiency and human disease. Nucleic Acids Res. 2018;46:1565–1583.
- Ruiz-Partida R, Prado S, Villarroya M, et al. An alternative homodimerization interface of MnmG reveals a conformational dynamics that is essential for its tRNA modification function. J Mol Biol. 2018;430:2822–2842.
- Liu X, Speckhard DC, Shepherd TR, et al. Distinct roles for conformational dynamics in protein-ligand interactions. Structure. 2016;24:2053–2066.
- Suzuki T. Chaplet column chromatography: isolation of a large set of individual RNAs in a single step. Methods Enzymol. 2007;425:231–239.
- Gehrke CW, Kuo KC. Ribonucleoside analysis by reversed-phase high-performance liquid chromatography. J Chromatogr. 1989;471:3–36.
- Eddy SR. Profile hidden Markov models. Bioinformatics. 1998;14:755–763.
- Pruitt KD, Tatusova T, Brown GR, Maglott DR. NCBI Reference Sequences. (RefSeq): current status, new features and genome annotation policy. Nucleic Acids Res. 2012;40:D130–5.
- Do CB, Mahabhashyam MS, Brudno M, et al. ProbCons: probabilistic consistency-based multiple sequence alignment. Genome Res. 2005;15:330–340.
- Mosquera-Rendon J, Cardenas-Brito S, Pineda JD, et al. Evolutionary and sequence-based relationships in bacterial AdoMet-dependent non-coding RNA methyltransferases. BMC Res Notes. 2014;7:440.