ABSTRACT
DIS3L2, in which mutations have been linked to Perlman syndrome, is an RNA-binding protein with 3′-5′ exoribonuclease activity. It contains two CSD domains and one S1 domain, all of which are RNA-binding domains, and one RNB domain that is responsible for the exoribonuclease activity. The 3′ polyuridine of RNA substrates can serve as a degradation signal for DIS3L2. Because DIS3L2 is predominantly localized in the cytoplasm, it can recognize, bind, and mediate the degradation of cytoplasmic uridylated RNA, including pre-microRNA, mature microRNA, mRNA, and some other non-coding RNAs. Therefore, DIS3L2 plays an important role in cytoplasmic RNA surveillance and decay. DIS3L2 is involved in multiple biological and physiological processes such as cell division, proliferation, differentiation, and apoptosis. Nonetheless, the function of DIS3L2, especially its association with cancer, remains largely unknown. We summarize here the RNA substrates degraded by DIS3L2 with its exonucleolytic activity, together with the corresponding biological functions it is implicated in. Furthermore, we discuss whether DIS3L2 can function independently of its 3′-5′ exoribonuclease activity, as well as its potential tumor-suppressive or oncogenic roles during cancer progression.
Introduction
DIS3 like 3′-5′ exoribonuclease 2 (DIS3L2), a member of the highly conserved RNase II/RNB family, is one kind of 3′-5′ exoribonuclease in H.sapiens [Citation1–Citation3], M.musculus [Citation4], S.pombe [Citation5], D.melanogaster [Citation6–Citation8], C.elegans [Citation9,Citation10], and so on. DIS3L2 consists of two cold shock domains (CSD domains) at the N-terminus, a ribonuclease II domain (RNB domain) in the middle, and an S1 domain at the C-terminus () [Citation2]. Both the CSD domains and the S1 domain are predicted RNA-binding domains, but the conserved central RNB domain provides exoribonuclease activity [Citation2,Citation3]. Compared to its human homologs DIS3 and DIS3L1, DIS3L2 does not have the PilT N-terminus (PIN) domain which confers DIS3 and DIS3L1 their endonuclease activity [Citation11–Citation13]. Moreover, the PIN domain is responsible for their binding to the RNA exosome core [Citation13]. Therefore, DIS3L2 cannot degrade RNA in an exosome-dependent manner because it is unable to interact with exosomes [Citation2,Citation3,Citation5].
Both single- and double-stranded RNA substrates can be degraded by DIS3L2 in vitro through its 3′-5′ exoribonuclease activity [Citation2,Citation3]. Biochemical analysis has revealed that polyuridylation in the 3′ end of RNA transcripts can serve as a decay signal for DIS3L2 [Citation3–Citation5]. Furthermore, the crystal structure of DIS3L2 shows that it possesses a conserved active site and an RNA binding path similar to those of Escherichia coli RNase II which facilitate specific binding of the RNA substrates [Citation14,Citation15]. The precursor of let-7 was identified as the first substrate of DIS3L2, which is responsible for the degradation of uridylated pre-let-7 in mouse embryonic stem cells [Citation3,Citation4]. Furthermore, mature microRNA (miRNA) can also be targeted by DIS3L2 for decay [Citation16]. Besides miRNA, aberrant mRNAs [Citation2,Citation17] and a great number of other non-coding RNAs (ncRNAs) [Citation18–Citation20] are also substrates that are recognized and degraded by DIS3L2.
DIS3L2 has been found to be involved in multiple biological and physiological processes, including cell division [Citation1], proliferation [Citation7], differentiation [Citation3,Citation4,Citation21], and apoptosis [Citation17,Citation22]. The link of DIS3L2 to Wilms’ tumor, which is the most common renal tumor of childhood, has been well documented [Citation1,Citation23–Citation25]. Germline mutations in the DIS3L2 gene can lead to the Perlman syndrome of overgrowth and Wilms tumor susceptibility [Citation1,Citation26]. Moreover, mice with deficient DIS3L2 function exhibit some key Perlman-syndrome-associated phenotypes [Citation27]. Additionally, accumulating studies have demonstrated that DIS3L2 is associated with multiple other diseases [Citation28–Citation31]. However, study on DIS3L2’s function in cancer is scarce. We summarize here the RNA substrates degraded by DIS3L2 with its exonucleolytic activity, together with the associated biological functions it is involved in. In addition, we discuss the possibility that DIS3L2 functions independently of its 3′-5′ exoribonuclease activity, as well as its potential tumor-suppressive or oncogenic roles in cancer development.
DIS3L2-mediated decay of miRNA
One identified cellular function of DIS3L2 is to mediate lin28-regulated let-7 miRNA expression. Lin28, one kind of RNA-binding protein that is specifically expressed in undifferentiated embryonic stem cells (ESCs), suppresses let-7 maturation in mouse ESCs [Citation32]. Lin28a, one of lin28’s paralogs, is primarily localized in the cytoplasm and recruits the 3′ terminal uridylyl transferase (TUTase) TUT4 (also know as Zcchc11) or TUT7 (Zcchc6) to pre-let-7 [Citation32–Citation34]. Subsequently, TUT4 and TUT7 catalyze the uridylation of pre-let-7, which leads to the decay of pre-let-7 [Citation34]. As an 3′-5′ exoribonuclease localized in the cytoplasm, DIS3L2 was found to bind with the 3′ polyuridine (poly(U)) and contribute to the degradation of uridylated pre-let-7 in mouse ESCs [Citation4]. In vitro reconstitution assays revealed that tails with a minimum of ten uridines in pre-let-7 RNA substrates can stimulate the ribonucleolytic activity of DIS3L2 [Citation4]. Additionally, DIS3L2 has also been discovered to interact with lin28b, another lin28 paralog, and is required for the degradation of uridylated pre-let-7 in lin28b-expressing cancer cells [Citation35]. Furthermore, the crystal structure of mouse DIS3L2 in complex with 14 nucleotides of poly(U) RNA substrate has been determined [Citation14]. A conserved active site in DIS3L2 ensures its specificity of substrate recognition, which serves to explain the poly(U) preference of DIS3L2.
In addition to pre-miRNA, mature miRNA has also been reported to be degraded by DIS3L2 [Citation16]. Highly complementary target mRNAs of miRNA can regulate its stability by a mechanism involving 3′ terminal tailing and the following trimming of it, which is named target-RNA-directed miRNA degradation (TDMD) [Citation36]. Haas et al. established a biochemical method of inducing TDMD in cell lines and identified TUT1 and DIS3L2 as the two cellular enzymes responsible for the target-RNA-induced decay of mature miRNAs [Citation16]. TUT1, one kind of TUTase, is recruited to the RNA-induced silencing complex (RISC) by Argonaute 2 in an RNA-dependent manner. Furthermore, DIS3L2, another protein that interacts with Argonaute 2, is also recruited to the RISC and is involved in the degradation of mature miRNA, such as miR-27 [Citation16].
However, one recent study demonstrated that DIS3L2 binds with and degrades RNA by a polyuridylation-independent mechanism [Citation21]. Although lin28a suppresses the biogenesis of let-7 by motivating the polyuridylation and degradation of their precursor pre-let-7 [Citation37], lin28a reduces the expression of miR-9 by destabilizing pre-miR-9 independently of the polyuridylation [Citation38]. Through restricting the biogenesis of miR-9, lin28a affects neuronal differentiation of the embryonic carcinoma cell line P19 [Citation38]. DIS3L2 was determined to cooperate with lin28a and regulate levels of miR-9 during neuronal differentiation of P19 cells [Citation21]. Although both pre-let-7a and pre-miR-9 possess the canonical lin28a-binding GGAG motif in their conserved terminal loops, different from pre-let-7a, which binds both CSD and tandem zinc-finger (ZnF) domains – two highly conserved RNA-binding motifs of lin28a – pre-miR-9 primarily interacts with lin28a via its CSD domain. Moreover, DIS3L2 contributes to lin28a-downregulated miR-9 levels by directly binding and degrading pre-miR-9, independent of its polyuridylation [Citation21].
DIS3L2-mediated decay of mRNA
As an exosome-independent 3′-5′ exoribonuclease, DIS3L2 has also been revealed to regulate cytoplasmic mRNA metabolism [Citation2,Citation17,Citation22]. Human DIS3L2 can interact with XRN1, a major 5′-3′ exoribonuclease in the cytoplasm, in an RNA-dependent manner [Citation2]. Moreover, DIS3L2 resembles XRN1, which can stimulate mRNA degradation co-translationally [Citation39], in that it co-sediments with ribosomal monosomes and polysomes. Therefore, DIS3L2 may also function in the decay of mRNA during translation, as validated by a recent study on co-translational nonsense-mediated messenger RNA decay (NMD) [Citation40]. Furthermore, DIS3L2 depletion upregulates the number of p-bodies [Citation2], which are mainly involved in mRNA storage [Citation41]. As a result, DIS3L2 plays an important role in maintaining the homeostasis of cellular RNA, especially AU-rich elements (AREs)-containing mRNA [Citation2]. Additionally, a similar character and effect of DIS3L2 exoribonuclease in degrading cytoplasmic mRNA in the 3′-5′ direction has also been observed in Schizosaccharomyces pombe [Citation5].
Recently, it was uncovered that DIS3L2 mediates rapid and global degradation of mRNA early in apoptosis [Citation17]. Diverse classical apoptotic stimuli can induce mitochondrial outer membrane permeabilization (MOMP) in multiple cell types, resulting in release of cytochrome c from the mitochondria into the cytosol, where it activates the apoptotic caspase cascade [Citation42]. Under the influence of caspase activation, TUT4 and TUT7 uridylate the mRNA decay intermediates near their stop codons. Subsequently, the uridylated mRNAs are digested by DIS3L2, which leads to translation arrest and cell death [Citation17].
Furthermore, Liu et al. revealed the mechanism of how mRNA decay is triggered by MOMP upon apoptotic stimuli and what kinds of RNAs are degraded [Citation22]. Polyribonucleotide nucleotidyltransferase 1 (PNPT1), a 3′-5′ exoribonuclease of mitochondrial intermembrane space, is released to the cytosol during MOMP and initiates the RNA decay. Cytosolic mRNAs, as well as non-coding RNAs with poly(A), are preferentially bound and attacked at their 3′ ends by PNPT1. Subsequently, TUTases TUT4 and TUT7 may recognize and uridylate the partially degraded RNA fragment, providing RNA substrates for DIS3L2-mediated degradation. Therefore, PNPT1 and DIS3L2 play nonredundant and contextual roles during MOMP-mediated RNA decay and cell death [Citation22].
DIS3L2-mediated decay of other ncRNAs
In addition to miRNA and mRNA, numerous long non-coding RNAs (lncRNAs) and short non-coding RNAs have also been discovered to be targets of DIS3L2 [Citation18–Citation20]. Pirouz et al. systematically identified RNA targets that were directly bound by a mutant version of mouse DIS3L2 (D389N substitution), which lacks 3′-5′ exoribonuclease activity, in DIS3L2-knockout ESCs [Citation18]. Their study showed that the majority of DIS3L2-associated RNA species are non-coding RNAs including Rmrp and 7SL, snRNAs such as U1 and U2, 5s rRNA, several snoRNAs, and so on. Although Rmrp is a primarily nuclear RNA [Citation43], the uridylated Rmrp transcripts accumulate in the cytoplasm of DIS3L2-deficient mouse ESCs. Furthermore, deep sequencing analysis showed that the oligouridylation tail is specifically added to Rmrp transcripts with an imprecise 3′ end. This study indicates that TUTase-dependent DIS3L2-mediated decay is a quality-control pathway that clears away the aberrant non-coding RNAs [Citation18].
Another study also performed a global analysis of DIS3L2’s substrates by overexpressing a wild type or catalytically inactive mutant (D391N) of human DIS3L2 in stable cell lines with DIS3L2 deficiency [Citation19]. RNA-seq analysis demonstrated that DIS3L2 affects the abundance of various types of RNA, including vault RNAs, Y RNAs, BC200 RNA (an Alu-homologue derived RNA), FTL_short RNA (a stem loop from the 5′-UTR of ferritin mRNA), snRNAs, and histone-coding mRNAs. These cytoplasmic RNAs are significantly upregulated in cells with DIS3L2 knockdown. Moreover, all these RNA substrates of DIS3L2 are uridylated by TUT4 or TUT7 in vivo. The snRNAs are a group of non-polyadenylated transcripts whose maturation process consists of multiple nuclear and cytoplasmic regulatory steps, including transcription and generation of snRNA precursors (pre-snRNAs) in the nucleus, export to the cytoplasm for maturation by cap trimethylation and 3′ end trimming, assembly of functional complexes, and re-import into the nucleus [Citation44,Citation45]. However, DIS3L2 deficiency results in the accumulation of snRNA transcripts that are longer than canonical pre-snRNAs. Therefore, DIS3L2 is regarded as a nuclease that is involved in the surveillance of maturing snRNA transcripts [Citation19].
Besides this, Ustianenko et al. also identified in vivo RNA targets of human DIS3L2 by the method of cross-linking and immunoprecipitation followed by RNA sequencing (CLIP-seq) in a HEK293T cell line stably expressing the catalytically inactive DIS3L2 (D391N) [Citation20]. It was discovered that besides vault RNAs, Y RNAs, snRNAs, miRNAs, and mRNAs, mutant DIS3L2 can also bind rRNAs, snoRNAs, tRNAs, transcription start site-associated short RNAs (TSSas), and so on. Moreover, TUTase–DIS3L2 functions as a surveillance pathway that leads to turnover of aberrant structured ncRNAs in the cytoplasm [Citation20]. The RNA substrates whose degradation is mediated by DIS3L2, the TUTases as partners of DIS3L2, and the corresponding biological functions they are involved in are summarized in .
Table 1. DIS3L2-mediated RNA decay and corresponding biological function it impacts.
Can DIS3L2 function independently of its 3′-5′ exoribonuclease activity?
The current studies show that the predominant function of DIS3L2 is to degrade RNA by its 3′-5′ exoribonuclease activity in an exosome-independent manner [Citation2,Citation3,Citation5]. Furthermore, the overwhelming majority of RNA decay mediated by DIS3L2 depends on the polyuridylation in the 3′ end of the RNA substrates [Citation3–Citation5]. However, DIS3L2 has also been reported to degrade RNA in a polyuridylation-independent manner [Citation21]. Therefore, it may exert its exoribonuclease activity via distinct functional mechanisms. Moreover, it is possible that through its CSD or S1 RNA-binding domain, DIS3L2 can bind RNA targets via motifs other than poly(U). Consequently, it would be worthwhile to explore whether DIS3L2 can function beyond its 3′-5′ exoribonuclease activity. By directly binding to the RNA transcripts, including mRNAs and ncRNAs, RNA-binding proteins can regulate various aspects of RNA metabolism such as RNA transcription [Citation46], splicing [Citation47,Citation48], transport [Citation49,Citation50], stability [Citation51,Citation52], translation [Citation53,Citation54], and modification [Citation55–Citation57]. Therefore, as an RNA-binding protein, DIS3L2 may be involved in the modulation of these RNA metabolism processes, except for the RNA stability or degradation that has been identified in previous studies. This research direction should be more strongly emphasized in future investigations of DIS3L2’s undiscovered functions.
Both endogenous and exogenous cellular localization of DIS3L2 have been examined by immunofluorescence assay in several cell lines, such as HeLa [Citation1–Citation3], COS7[Citation1], and HEK293T [Citation2,Citation3]. It was demonstrated that DIS3L2 is predominantly expressed in the cytoplasm [Citation1–Citation3]. However, a recent study reported variation of DIS3L2’s localization in intranuclear inclusion body disease (INIBD), a neurodegenerative disease characterized by neuronal and glial nuclear inclusions [Citation31]. By immunohistochemical assay, it was observed that DIS3L2 localizes in neuronal cytoplasm in the brains of normal control subjects. However, it is sequestrated to the neuronal and glial nuclear inclusions in the brains of patients with INIBD, which may be associated with the pathogenesis of INIBD [Citation31]. Can DIS3L2 localize in other organelles such as the endoplasmic reticulum, Golgi apparatus, or mitochondria? It has been shown that RNA substrates can undergo uridylation in the nucleus, such as U6 snRNA [Citation58,Citation59], and in the mitochondria, such as guide RNAs (gRNAs) and mRNAs [Citation60,Citation61]. Therefore, it is possible that DIS3L2 exerts its exoribonuclease activity in these subcellular organelles, resulting in a novel cellular effect. In addition, subcellular localization may confer DIS3L2 a potential subcellular compartment-specific role outside of the ability of 3′-5′ exoribonuclease. Consequently, future experimental studies would be needed to clarify whether DIS3L2 can function within an organelle, dependent on or independent of its exoribonuclease activity.
Does DIS3L2 function as an inhibitor or a driver during cancer progression?
Although a minority of studies have linked DIS3L2 to cancers [Citation1,Citation62], the regulatory role of DIS3L2 as a tumor suppressor or an oncogene in carcinogenesis and cancer development is inconclusive. Research findings have shown that knockdown of DIS3L2 promotes cell proliferation [Citation7] and cancer cell growth [Citation1]. The expression of cell cycle regulator cyclin D1 [Citation63] is increased in cells with DIS3L2 deficiency but decreased in cells with DIS3L2 overexpression. However, the expression of cell cycle inhibitors P27 [Citation64] and p21 [Citation65] is elevated in cells over-expressing DIS3L2 [Citation1]. Therefore, it is suggested that DIS3L2 has a negative regulatory effect on cell cycle progression. Moreover, one recent study showed that DIS3L2 may mediate an inhibitive effect of lncRNA-AC105461.1 on colorectal cancer’s stem-cell-like characteristics [Citation62], which are related to the maintenance and growth of tumors [Citation66]. Consequently, these findings indicate that DIS3L2 plays a tumor suppressive role in cancer ().
However, the finding that DIS3L2 cooperates with lin28 to suppress let-7 maturation suggests an oncogenic role; that is because lin28 functions as an oncogene, whereas the let-7 family of microRNAs function as tumor suppressors in many cancers [Citation67–Citation69]. It is possible that DIS3L2 promotes cancer development by degrading pre-let-7. In addition, the association between DIS3L2 and oncogene lin28 has been investigated on the basis of the CCLE database, which compiles genome-wide expression profiles from diverse cancer cell lines [Citation70]. The clustering analysis of expression profiles shows high expression of TUT4 and Dis3l2 as well as let-7 targets in lin28b-positive cancer cells [Citation35]. Moreover, an analysis based on The Cancer Genome Atlas (TCGA) data also supports a positive correlation between the expression of lin28b and DIS3L2 in human hepatocellular carcinoma [Citation35]. Taken together, these studies shed light on a potential oncogenic role of DIS3L2 in cancer ().
Figure 1. Structure of human DIS3L2. Human DIS3L2 contains two CSD domains (CSD1, CSD2), one RNB domain, and one S1 domain. Both the CSD domain and the S1 domain are RNA binding domains. The RNB domain provides 3ʹ-5ʹ exoribonuclease activity and the aspartic acid at position 391 (D391) is a catalytic site, whose mutation leads to inhibition of DIS3L2’s exoribonuclease activity.

Figure 2. Function of DIS3L2 during cancer progression. (a) DIS3L2 suppresses the expression of cyclin D1 but enhances the expression of p27 and p21, therefore promoting the cell cycle progression and functioning as an inhibitor during cancer progression. (b) DIS3L2 promotes lin28-mediated suppression of pre-let-7, results in reduced let-7 biogenesis, and facilitates cancer progression.
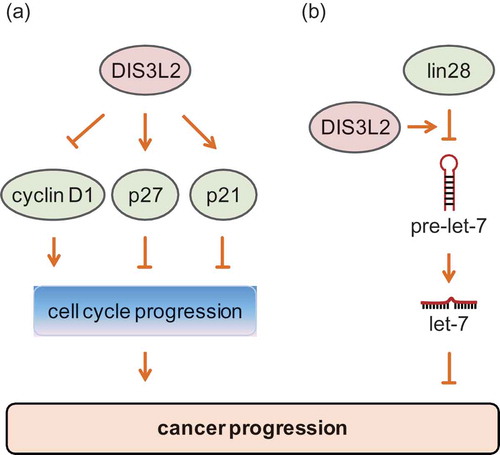
Therefore, there is no conclusion on whether DIS3L2 functions as an inhibitor or a driver during cancer progression. DIS3L2 can be a tumor suppressor in one cancer type, but functions as an oncogene during the development of another cancer type. In other words, the suppressive or enhancive function of DIS3L2 in cancer progression may depend on the type of cancer it resides in. Additionally, it cannot be overlooked that the RNA substrates targeted by DIS3L2, including oncogenes and tumor suppressors, may determine what kind of function it exerts. Future investigation on the aberrant expression of DIS3L2 in clinical specimens, as well as the elucidation of its function and functional mechanism in cancer development, would serve to uncover whether DIS3L2 plays an oncogenic or a tumor-suppressive role in the progression of any specific cancer type.
It is noteworthy that DIS3L2 has been found to facilitate the rapid turnover of aberrant mRNA in response to multiple stresses, including retroviral infections [Citation71] and apoptosis [Citation17]. Does DIS3L2 function in response to other cellular stresses? One question that deserves to be addressed is whether DIS3L2 plays a role in confronting various environmental stresses such as hypoxia and nutrient depletion, both of which are characteristics of the cancer microenvironment [Citation72]. To adapt to the harsh microenvironment, cancer cells can usually work out various effective strategies, including altering the expression of lncRNAs and their following functions [Citation73–Citation75]. It is possible that DIS3L2 can regulate the expression, function, or life cycle of this kind of lncRNAs, maybe in a manner that does not rely on its exoribonuclease activity, and can confer cancer cells the ability to proliferate and survive in the tough microenvironment. Functional experimental research about DIS3L2’s response to the cancer microenvironment may help in clarifying whether DIS3L2 functions as an inhibitor or a driver in any specific cancer type.
Conclusions
DIS3L2 has received more attention since it was confirmed to have exoribonuclease activity in 2012 [Citation1]. 3′ RNA uridylation, the non-templated addition of uridines to the terminal end of RNA transcripts, is a signal for DIS3L2-mediated RNA decay. DIS3L2 plays a crucial role in cytoplasmic RNA surveillance and degradation by virtue of its 3′-5′ exoribonuclease activity [Citation2,Citation5,Citation6,Citation19,Citation20]. Moreover, DIS3L2 dysfunction disturbs transcriptome homeostasis [Citation19]. However, the current understanding of DIS3L2’s function is limited: we know only that it can bind to and promote the decay of RNA in the cytoplasm, including miRNAs, mRNAs, and some other long or short ncRNAs (). It would be worthwhile to explore whether DIS3L2 can exert its 3′-5′ exoribonuclease activity in the nucleus or other organelles, or whether it can function independently of its exoribonuclease activity in the cytosol or organelles.
In addition, research on the roles of DIS3L2 in carcinogenesis and cancer development is scarce. Emerging evidence indicates that DIS3L2 can not only act as an oncogene, but can also function as a tumor suppressor. It may play distinct or even opposite roles during the progression of different cancer types. Although DIS3L2 has been reported to regulate the turnover of cancer-related RNAs such as vault RNAs and Y RNAs [Citation19,Citation76,Citation77], it may also affect cancer progression by regulating the expression, function, or metabolism of other RNAs, possibly through an unknown mechanism that does not depend on its 3′-5′ exoribonuclease activity. In sum, the cellular functions and functional mechanisms of DIS3L2 remain far from being elucidated. Future studies should aim at discovering other undetected biological and physiological functions of DIS3L2, especially its association with cancer.
Authors’ contributions
SYL, JYL, and HL collected references and wrote the manuscript. ZYL revised and completed the final manuscript.
Acknowledgments
This work was supported by the National Natural Science Foundation of China (81772552, 81572714, 81372215) and the Fundamental Research Funds for the Central Universities of China (531107051117).
Disclosure statement
No potential conflict of interest was reported by the authors.
References
- Astuti D, Morris MR, Cooper WN, et al. Germline mutations in DIS3L2 cause the Perlman syndrome of overgrowth and Wilms tumor susceptibility. Nat Genet. 2012;44:277–284.
- Lubas M, Damgaard CK, Tomecki R, et al. Exonuclease hDIS3L2 specifies an exosome-independent 3ʹ-5ʹ degradation pathway of human cytoplasmic mRNA. Embo J. 2013;32:1855–1868.
- Ustianenko D, Hrossova D, Potesil D, et al. Mammalian DIS3L2 exoribonuclease targets the uridylated precursors of let-7 miRNAs. RNA. 2013;19:1632–1638.
- Chang HM, Triboulet R, Thornton JE, et al. A role for the Perlman syndrome exonuclease DIS3L2 in the Lin28-let-7 pathway. Nature. 2013;497:244–248.
- Malecki M, Viegas SC, Carneiro T, et al. The exoribonuclease DIS3L2 defines a novel eukaryotic RNA degradation pathway. Embo J. 2013;32:1842–1854.
- Reimao-Pinto MM, Manzenreither RA, Burkard TR, et al. Molecular basis for cytoplasmic RNA surveillance by uridylation-triggered decay in Drosophila. Embo J. 2016;35:2417–2434.
- Towler BP, Jones CI, Harper KL, et al. A novel role for the 3ʹ-5ʹ exoribonuclease DIS3L2 in controlling cell proliferation and tissue growth. RNA Biol. 2016;13:1286–1299.
- Lin CJ, Wen J, Bejarano F, et al. Characterization of a TUTase/RNase complex required for Drosophila gametogenesis. RNA. 2017;23:284–296.
- Weaver BP, Zabinsky R, Weaver YM, et al. CED-3 caspase acts with miRNAs to regulate non-apoptotic gene expression dynamics for robust development in C. elegans. Elife. 2014;3:e04265.
- Zhou X, Feng X, Mao H, et al. RdRP-synthesized antisense ribosomal siRNAs silence pre-rRNA via the nuclear RNAi pathway. Nat Struct Mol Biol. 2017;24:258–269.
- Lebreton A, Tomecki R, Dziembowski A, et al. Endonucleolytic RNA cleavage by a eukaryotic exosome. Nature. 2008;456:993–996.
- Schaeffer D, Tsanova B, Barbas A, et al. The exosome contains domains with specific endoribonuclease, exoribonuclease and cytoplasmic mRNA decay activities. Nat Struct Mol Biol. 2009;16:56–62.
- Schneider C, Leung E, Brown J, et al. The N-terminal PIN domain of the exosome subunit Rrp44 harbors endonuclease activity and tethers Rrp44 to the yeast core exosome. Nucleic Acids Res. 2009;37:1127–1140.
- Faehnle CR, Walleshauser J, Joshua-Tor L. Mechanism of Dis3l2 substrate recognition in the Lin28-let-7 pathway. Nature. 2014;514:252–256.
- Frazao C, McVey CE, Amblar M, et al. Unravelling the dynamics of RNA degradation by ribonuclease II and its RNA-bound complex. Nature. 2006;443:110–114.
- Haas G, Cetin S, Messmer M, et al. Identification of factors involved in target RNA-directed microRNA degradation. Nucleic Acids Res. 2016;44:2873–2887.
- Thomas MP, Liu X, Whangbo J, et al. Apoptosis triggers specific, rapid, and global mRNA decay with 3ʹ uridylated intermediates degraded by DIS3L2. Cell Rep. 2015;11:1079–1089.
- Pirouz M, Du P, Munafo M, et al. DIS3L2-mediated decay is a quality control pathway for noncoding RNAs. Cell Rep. 2016;16:1861–1873.
- Labno A, Warkocki Z, Kulinski T, et al. Perlman syndrome nuclease DIS3L2 controls cytoplasmic non-coding RNAs and provides surveillance pathway for maturing snRNAs. Nucleic Acids Res. 2016;44:10437–10453.
- Ustianenko D, Pasulka J, Feketova Z, et al. TUT-DIS3L2 is a mammalian surveillance pathway for aberrant structured non-coding RNAs. Embo J. 2016;35:2179–2191.
- Nowak JS, Hobor F, Downie Ruiz Velasco A, et al. Lin28a uses distinct mechanisms of binding to RNA and affects miRNA levels positively and negatively. RNA. 2017;23:317–332.
- Liu X, Fu R, Pan Y, et al. PNPT1 release from mitochondria during apoptosis triggers decay of Poly(A) RNAs. Cell. 2018;174:187–201 e12.
- Wegert J, Ishaque N, Vardapour R, et al. Mutations in the SIX1/2 pathway and the DROSHA/DGCR8 miRNA microprocessor complex underlie high-risk blastemal type Wilms tumors. Cancer Cell. 2015;27:298–311.
- Soma N, Higashimoto K, Imamura M, et al. Long term survival of a patient with Perlman syndrome due to novel compound heterozygous missense mutations in RNB domain of DIS3L2. Am J Med Genet A. 2017;173:1077–1081.
- Higashimoto K, Maeda T, Okada J, et al. Homozygous deletion of DIS3L2 exon 9 due to non-allelic homologous recombination between LINE-1s in a Japanese patient with Perlman syndrome. Eur J Hum Genet. 2013;21:1316–1319.
- Morris MR, Astuti D, Maher ER. Perlman syndrome: overgrowth, Wilms tumor predisposition and DIS3L2. Am J Med Genet C Semin Med Genet. 2013;163C:106–113.
- Hunter RW, Liu Y, Manjunath H, et al. Loss of DIS3L2 partially phenocopies Perlman syndrome in mice and results in up-regulation of Igf2 in nephron progenitor cells. Genes Dev. 2018;32:903–908.
- Tassano E, Buttgereit J, Bader M, et al. Genotype-Phenotype correlation of 2q37 deletions including NPPC gene associated with skeletal malformations. PLoS One. 2013;8:e66048.
- Reis FP, Pobre V, Silva IJ, et al. The RNase II/RNB family of exoribonucleases: putting the ‘Dis’ in disease. Wiley Interdiscip Rev RNA. 2013;4:607–615.
- Pashler AL, Towler BP, Jones CI, et al. The roles of the exoribonucleases DIS3L2 and XRN1 in human disease. Biochem Soc Trans. 2016;44:1377–1384.
- Mori F, Tanji K, Miki Y, et al. Immunohistochemical localization of exoribonucleases (DIS3L2 and XRN1) in intranuclear inclusion body disease. Neurosci Lett. 2018;662:389–394.
- Hagan JP, Piskounova E, Gregory RI. Lin28 recruits the TUTase Zcchc11 to inhibit let-7 maturation in mouse embryonic stem cells. Nat Struct Mol Biol. 2009;16:1021–1025.
- Heo I, Joo C, Kim YK, et al. TUT4 in concert with Lin28 suppresses microRNA biogenesis through pre-microRNA uridylation. Cell. 2009;138:696–708.
- Thornton JE, Chang HM, Piskounova E, et al. Lin28-mediated control of let-7 microRNA expression by alternative TUTases Zcchc11 (TUT4) and Zcchc6 (TUT7). RNA. 2012;18:1875–1885.
- Suzuki HI, Katsura A, Miyazono K. A role of uridylation pathway for blockade of let-7 microRNA biogenesis by Lin28B. Cancer Sci. 2015;106:1174–1181.
- de la Mata M, Gaidatzis D, Vitanescu M, et al. Potent degradation of neuronal miRNAs induced by highly complementary targets. EMBO Rep. 2015;16:500–511.
- Heo I, Joo C, Cho J, et al. Lin28 mediates the terminal uridylation of let-7 precursor MicroRNA. Mol Cell. 2008;32:276–284.
- Nowak JS, Choudhury NR, de Lima Alves F, et al. Lin28a regulates neuronal differentiation and controls miR-9 production. Nat Commun. 2014;5:3687.
- Hu W, Sweet TJ, Chamnongpol S, et al. Co-translational mRNA decay in Saccharomyces cerevisiae. Nature. 2009;461:225–229.
- Kurosaki T, Miyoshi K, Myers JR, et al. NMD-degradome sequencing reveals ribosome-bound intermediates with 3ʹ-end non-templated nucleotides. Nat Struct Mol Biol. 2018;25:940–950.
- Standart N, Weil D. P-bodies: cytosolic droplets for coordinated mRNA storage. Trends Genet. 2018;34:612–626.
- Pena-Blanco A, Garcia-Saez AJ. Bax, Bak and beyond - mitochondrial performance in apoptosis. FEBS J. 2018;285:416–431.
- Jacobson MR, Cao LG, Wang YL, et al. Dynamic localization of RNase MRP RNA in the nucleolus observed by fluorescent RNA cytochemistry in living cells. J Cell Biol. 1995;131:1649–1658.
- Matera AG, Wang Z. A day in the life of the spliceosome. Nat Rev Mol Cell Biol. 2014;15:108–121.
- Guiro J, Murphy S. Regulation of expression of human RNA polymerase II-transcribed snRNA genes. Open Biol. 2017;7.
- Reuter LM, Meinel DM, Strasser K. The poly(A)-binding protein Nab2 functions in RNA polymerase III transcription. Genes Dev. 2015;29:1565–1575.
- Chang X, Li B, Rao A. RNA-binding protein hnRNPLL regulates mRNA splicing and stability during B-cell to plasma-cell differentiation. Proc Natl Acad Sci USA. 2015;112:E1888–1897.
- Maatz H, Jens M, Liss M, et al. RNA-binding protein RBM20 represses splicing to orchestrate cardiac pre-mRNA processing. J Clin Invest. 2014;124:3419–3430.
- Zhang P, Abdelmohsen K, Liu Y, et al. Novel RNA- and FMRP-binding protein TRF2-S regulates axonal mRNA transport and presynaptic plasticity. Nat Commun. 2015;6:8888.
- Wang J, Rajbhandari P, Damianov A, et al. RNA-binding protein PSPC1 promotes the differentiation-dependent nuclear export of adipocyte RNAs. J Clin Invest. 2017;127:987–1004.
- Zhou XJ, Wu J, Shi L, et al. PTEN expression is upregulated by a RNA-binding protein RBM38 via enhancing its mRNA stability in breast cancer. J Exp Clin Cancer Res. 2017;36:149.
- Hou P, Li L, Chen F, et al. PTBP3-mediated regulation of ZEB1 mRNA stability promotes epithelial-mesenchymal transition in breast cancer. Cancer Res. 2018;78:387–398.
- Roilo M, Kullmann MK, Hengst L. Cold-inducible RNA-binding protein (CIRP) induces translation of the cell-cycle inhibitor p27Kip1. Nucleic Acids Res. 2018;46:3198–3210.
- Andrade JM, Dos Santos RF, Chelysheva I, et al. The RNA-binding protein Hfq is important for ribosome biogenesis and affects translation fidelity. Embo J. 2018;37.
- Luo J, Liu H, Luan S, et al. Aberrant regulation of mRNA m(6)A modification in cancer development. Int J Mol Sci. 2018;19.
- Liu J, Yue Y, Han D, et al. A METTL3-METTL14 complex mediates mammalian nuclear RNA N6-adenosine methylation. Nat Chem Biol. 2014;10:93–95.
- Zheng G, Dahl JA, Niu Y, et al. ALKBH5 is a mammalian RNA demethylase that impacts RNA metabolism and mouse fertility. Mol Cell. 2013;49:18–29.
- Trippe R, Sandrock B, Benecke BJ. A highly specific terminal uridylyl transferase modifies the 3ʹ-end of U6 small nuclear RNA. Nucleic Acids Res. 1998;26:3119–3126.
- Trippe R, Guschina E, Hossbach M, et al. Identification, cloning, and functional analysis of the human U6 snRNA-specific terminal uridylyl transferase. RNA. 2006;12:1494–1504.
- Aphasizhev R, Aphasizheva I, Simpson L. A tale of two TUTases. Proc Natl Acad Sci USA. 2003;100:10617–10622.
- Aphasizheva I, Maslov D, Wang X, et al. Pentatricopeptide repeat proteins stimulate mRNA adenylation/uridylation to activate mitochondrial translation in trypanosomes. Mol Cell. 2011;42:106–117.
- Liu W, Yu Q, Ma J, et al. Knockdown of a DIS3L2 promoter upstream long noncoding RNA (AC105461.1) enhances colorectal cancer stem cell properties in vitro by down-regulating DIS3L2. Onco Targets Ther. 2017;10:2367–2376.
- Qie S, Diehl JA. Cyclin D1, cancer progression, and opportunities in cancer treatment. J Mol Med (Berl). 2016;94:1313–1326.
- Abbastabar M, Kheyrollah M, Azizian K, et al. Multiple functions of p27 in cell cycle, apoptosis, epigenetic modification and transcriptional regulation for the control of cell growth: A double-edged sword protein. DNA Repair (Amst). 2018;69:63–72.
- El-Deiry WS. p21(WAF1) mediates cell-cycle inhibition, relevant to cancer suppression and therapy. Cancer Res. 2016;76:5189–5191.
- Beck B, Blanpain C. Unravelling cancer stem cell potential. Nat Rev Cancer. 2013;13:727–738.
- Jiang S, Baltimore D. RNA-binding protein Lin28 in cancer and immunity. Cancer Lett. 2016;375:108–113.
- Thornton JE, Gregory RI. How does Lin28 let-7 control development and disease? Trends Cell Biol. 2012;22:474–482.
- Balzeau J, Menezes MR, Cao S, et al. The LIN28/let-7 pathway in cancer. Front Genet. 2017;8:31.
- Barretina J, Caponigro G, Stransky N, et al. The cancer cell line encyclopedia enables predictive modelling of anticancer drug sensitivity. Nature. 2012;483:603–607.
- Eckwahl MJ, Sim S, Smith D, et al. A retrovirus packages nascent host noncoding RNAs from a novel surveillance pathway. Genes Dev. 2015;29:646–657.
- Pavlova NN, Thompson CB. The emerging hallmarks of cancer metabolism. Cell Metab. 2016;23:27–47.
- Liu X, Xiao ZD, Han L, et al. LncRNA NBR2 engages a metabolic checkpoint by regulating AMPK under energy stress. Nat Cell Biol. 2016;18:431–442.
- Xiao ZD, Han L, Lee H, et al. Energy stress-induced lncRNA FILNC1 represses c-Myc-mediated energy metabolism and inhibits renal tumor development. Nat Commun. 2017;8(1):783.
- Khan MR, Xiang S, Song Z, et al. The p53-inducible long noncoding RNA TRINGS pro0tects cancer cells from necrosis under glucose starvation. Embo J. 2017;36:3483–3500.
- Chen J, OuYang H, An X, et al. Vault RNAs partially induces drug resistance of human tumor cells MCF-7 by binding to the RNA/DNA-binding protein PSF and inducing oncogene GAGE6. PLoS One. 2018;13:e0191325.
- Tolkach Y, Niehoff EM, Stahl AF, et al. YRNA expression in prostate cancer patients: diagnostic and prognostic implications. World J Urol. 2018;36:1073–1078.