ABSTRACT
Transcriptional pauses have been reported in bacterial riboswitches and, in some cases, their specific positioning has been shown to be important for gene regulation. Here, we show that a hairpin structure in the Escherichia coli thiamin pyrophosphate (TPP) thiC riboswitch is involved in transcriptional pausing and ligand sensitivity. Using in vitro transcription kinetic experiments, we show that all three major transcriptional pauses in the thiC riboswitch are affected by NusA, a transcriptional factor known to stimulate hairpin-stabilized pauses. Using a truncated region of the riboswitch, we isolated the hairpin structure responsible for stabilization of the most upstream pause. Destabilization of this structure led to a weaker pause and a decreased NusA effect. In the context of the full-length riboswitch, this same mutation also led to a weaker pause, as well as a decreased TPP binding affinity. Our work suggests that RNA structures involved in transcriptional pausing in riboswitches are important for ligand sensitivity, most likely by increasing the time allowed to the ligand for binding to the riboswitch.
Introduction
Riboswitches are non-coding RNA structures that modulate gene expression in response to a specific metabolite or ion concentrations [Citation1]. They are composed of two domains: the aptamer, which acts as a metabolite sensor, and the expression platform, which is involved in controlling gene expression [Citation2,Citation3]. It has been previously suggested that cotranscriptional RNA folding might play a crucial role in the formation of functional structures of many RNA such as riboswitches [Citation4,Citation5]. The cotranscriptional folding process depends on transcription elongation parameters such as the RNA polymerase (RNAP), the elongation rate and transcriptional pausing [Citation5]. The process of transcriptional pausing is directly involved in cotranscriptional RNA folding as it may allow more time for upstream RNA segments to adopt structures before downstream sequences might interfere [Citation4]. Transcriptional pausing has been reported to be important for the regulation of various riboswitches. For example, transcriptional pause sites have been detected in the Bacillus subtilis ribD [Citation6] and pbuE [Citation7] riboswitches, the Salmonella enterica mgtA [Citation8] as well as the Escherichia coli btuB, lysC, tbpA, ribB, thiC and thiM riboswitches [Citation9–Citation12]. Interestingly, both the S. enterica mgtA [Citation8] and E. coli thiC [Citation9] riboswitches modulate Rho-dependent transcription termination occurring at a transcriptional pause site in the expression platform, suggesting that strategically located pause sites may be used by riboswitches to control gene expression.
NusA is an elongation factor that contributes to RNA folding during transcription by enhancing transcriptional pausing [Citation5,Citation13–Citation16]. NusA has been reported to be involved in transcriptional pausing within the his leader region, which contains a hairpin located 11 nucleotides upstream of the pause site [Citation17]. The role of the hairpin in the his pause has been extensively characterized, and various parameters such as the hairpin stability, the distance between the stem and the pause site, and the sequence of the loop have been shown to be important for NusA-dependent transcriptional pausing [Citation18]. The increase in NusA-dependent transcriptional pausing is due to its N-terminal domain, which interacts with the nascent RNA hairpin located near the RNAP exit channel [Citation19,Citation20]. In this process, NusA stabilizes nascent hairpin structures interacting with RNAP, thereby increasing the half-life of transcriptional pauses [Citation5,Citation21]. Interestingly, the fact that NusA has been shown to modulate riboswitch transcriptional pausing [Citation6,Citation7,Citation11] suggests the presence of hairpin motifs that might be important for transcriptional pausing and genetic regulation. However, due to the small number of known NusA target sites [Citation5], hairpin-stabilized NusA regulatory domains are difficult to predict.
In this study, we have characterized a novel stem-loop structure that interacts with NusA in the E. coli thiC riboswitch and shown that NusA impacts all transcriptional pauses as well as riboswitch metabolite sensing. To identify the structural determinants involved in NusA activity on transcriptional pausing, we isolated the stem-loop upstream of the first transcriptional pause site (A138) and showed that it constitutes an independent hairpin-stabilized pause signal. We also report that the stability of the stem-loop is important for transcriptional pausing and riboswitch activity. Taken together, our results support the notion that NusA and an RNA hairpin structure act together to stimulate transcriptional pausing, which constitutes an important control mechanism of riboswitch activity.
Results
Impact of NusA on thiC riboswitch transcriptional pausing
The thiC riboswitch is located upstream of the thiCEFSGH operon, which is involved in the biosynthesis of thiamin pyrophosphate (TPP) and related metabolites [Citation22]. Upon TPP binding, the thiC riboswitch downregulates gene expression by sequestering the Shine-Dalgarno (SD) sequence and AUG start codon () [Citation23], thereby preventing the initiation of translation [Citation9]. However, in the absence of TPP, it was determined that the RNA adopts an alternative highly structured configuration () in which thiC translation initiation is allowed [Citation9]. Kinetics of transcription elongation previously revealed that the thiC riboswitch expression platform exhibits transcriptional pause sites at positions A138, C158 and C187.[Citation9,Citation11], In contrast to A138 and C158, it was observed that pausing at C187 is increased by ~threefold upon TPP binding, which was found to be important for Rho-dependent transcription termination at position C187. [Citation9]
Figure 1. Structure of the thiC riboswitch.
Secondary structures of the thiC riboswitch representing the TPP-bound (A) and the TPP-free (B) states[Citation9]. Transcriptional pause sites are indicated by black circles[Citation9]. The nomenclature of the helical domains is based on a previous study[Citation23].
![Figure 1. Structure of the thiC riboswitch.Secondary structures of the thiC riboswitch representing the TPP-bound (A) and the TPP-free (B) states[Citation9]. Transcriptional pause sites are indicated by black circles[Citation9]. The nomenclature of the helical domains is based on a previous study[Citation23].](/cms/asset/cd357563-b547-433b-b684-1a77abedccd6/krnb_a_1616354_f0001_oc.jpg)
To determine if NusA modulates transcriptional pausing in the context of the thiC riboswitch, transcription elongation reactions were monitored over time in the presence of NusA. For these experiments, we performed single-round in vitro transcription assays and analysed transcripts at various time points (). Analysis of our data, when compared with our previous study, showed that NusA increased by ~3 to ~fourfold the half-life of all pauses in the absence of TPP (), Table S1, Rows 1, 3 and 5). However, in the presence of TPP, we observed that NusA increased the half-life of pause sites A138 and C158 – but not C187 – by ~4 to ~5-fold (), Table S1, Rows 2, 4 and 6). We also observed that NusA only had a minor effect (~1.3-fold) on the C187 pause half-life in the presence of TPP (), Table S1, Row 6). Together, our results indicate that NusA significantly modulates transcriptional pausing in the thiC riboswitch for all sites in the absence of TPP, suggesting that NusA may affect the riboswitch regulation mechanism.
Figure 2. NusA-dependent transcriptional pausing in the thiC riboswitch.
(A) Transcription kinetics experiments performed on the thiC riboswitch using 25 µM NTP, in presence of 50 nM NusA, and in absence and presence of 10 µM TPP. This specific NTP concentration was used to facilitate detection of pausing. Pause sites A138, C158 and C187, and full-length products (FL) are indicated on the right, and sampling times are indicated on top of the gel. (B) Quantification of the half-lives of all three transcriptional pauses in the presence of 50 nM NusA. Experiments were performed using 100 µM NTP in absence and presence of 10 µM TPP. Values obtained in the absence of NusA [Citation9] are shown for comparison. (C) Kswitch determination experiment using the SD probe [Citation9] in absence (-NusA) and presence (+NusA) of 50 nM NusA during transcription reactions (1 mM NTP). In vitro transcription experiments were performed using increasing TPP concentrations ranging from 100 pM to 100 µM. The full-length (FL) and cleavage products (P) are indicated on the right of the gel. (D) Quantification analysis of experiments shown in (C), yielding a Kswitch value of 653 ± 42 nM in absence of NusA and 300 ± 21 nM in presence of 50 nM NusA.
![Figure 2. NusA-dependent transcriptional pausing in the thiC riboswitch.(A) Transcription kinetics experiments performed on the thiC riboswitch using 25 µM NTP, in presence of 50 nM NusA, and in absence and presence of 10 µM TPP. This specific NTP concentration was used to facilitate detection of pausing. Pause sites A138, C158 and C187, and full-length products (FL) are indicated on the right, and sampling times are indicated on top of the gel. (B) Quantification of the half-lives of all three transcriptional pauses in the presence of 50 nM NusA. Experiments were performed using 100 µM NTP in absence and presence of 10 µM TPP. Values obtained in the absence of NusA [Citation9] are shown for comparison. (C) Kswitch determination experiment using the SD probe [Citation9] in absence (-NusA) and presence (+NusA) of 50 nM NusA during transcription reactions (1 mM NTP). In vitro transcription experiments were performed using increasing TPP concentrations ranging from 100 pM to 100 µM. The full-length (FL) and cleavage products (P) are indicated on the right of the gel. (D) Quantification analysis of experiments shown in (C), yielding a Kswitch value of 653 ± 42 nM in absence of NusA and 300 ± 21 nM in presence of 50 nM NusA.](/cms/asset/1e05a180-7e91-4ba8-b55b-7f92923552a2/krnb_a_1616354_f0002_oc.jpg)
Impact of NusA on thiC riboswitch metabolite sensing
Since the thiC riboswitch was previously shown to perform metabolite sensing within a narrow transcriptional window enclosing A138 and C158 pause sites [Citation9], we postulated that the NusA-dependent-increased transcriptional pausing at these pause sites might favour the cotranscriptional recognition of TPP. To demonstrate this, we monitored the TPP-dependent riboswitch structural transition () in the presence of NusA by using an RNase H cleavage assay [Citation9,Citation24] with a DNA oligonucleotide designed to monitor the accessibility of the SD region. As previously observed [Citation9], increasing the concentration of TPP led to a protection of the SD region (), -NusA), indicative of the riboswitch adopting the OFF state upon TPP binding (). Analysis of the cleaved fraction as a function of TPP concentration yielded a Kswitch value of 653 ± 42 nM and 300 ± 21 nM in the absence and in presence of NusA, respectively (). This NusA-induced ~2.2-fold increase in affinity is consistent with NusA allowing more time for the thiC riboswitch to bind TPP within a narrow transcriptional window before the elongating RNAP reaches the C187 pause site, a position where nascent riboswitches possess a low TPP affinity [Citation9].
Isolation of the hairpin-stabilized pause signal responsible for the effect of NusA on the pause at position A138
To study the influence of NusA on thiC riboswitch activity, we characterized the pause site at position A138, which represents the first transcriptional pause site within the riboswitch expression platform. Close inspection of the thiC riboswitch secondary structure in the TPP-free conformation () suggests that the stem-loop encompassing residues 105 to 126 might be in close proximity to the RNAP when pausing occurs at A138. To determine if this stem-loop (A138 stem-loop) could be involved in transcriptional pausing at A138, a construct corresponding to the region comprising nucleotides 87 to 155 of the thiC riboswitch was designed (see Materials and Methods). Kinetics of transcription elongation revealed that a pause corresponding to position A138 site was present (), confirming that the region including nucleotides 87 to 155 was sufficient to induce transcriptional pausing. Similar transcription elongation reactions in the presence of NusA, showed that the A138 pause half-life was increased by ~1.8-fold (), Table S1, Rows 7 and 8), in agreement with the data obtained with the full-length thiC riboswitch (). To determine if the transcriptional pausing was caused by the presence of the stem-loop encompassing residues 105–126, we introduced mutations to destabilize the stem structure by changing residues 119–122 for their Watson-Crick counterpart (stem mutant). Kinetics of transcription elongation () showed that the pause half-life in the stem mutant was reduced by ~1.9-fold compared to the wild-type construct (), Table S1, Rows 7 and 9), consistent with the integrity of the stem-loop being important for transcriptional pausing. Furthermore, we observed that NusA decreased its effect on pausing by ~2.2-fold, when comparing the mutant and the wild-type constructs (), Table S1, Rows 7, 8, 9 and 10). These results show that the stability of the stem-loop is important both for hairpin- and NusA-dependent pausing. ()). Together, these results show that the isolated stem-loop is sufficient to observe stabilization of the pause at position A138 by NusA and that the stability of the stem is directly related to the efficiency of transcriptional pausing.
Figure 3. Transcriptional pausing in the isolated A138 stem-loop region.
(A) Transcription kinetics experiments performed on the A138 stem-loop region of the thiC riboswitch using 25 µM NTP, in absence and presence of 50 nM NusA. The A138 pause site and full-length product (FL) are indicated on the right, and sampling times are indicated on top of the gel. (B) Quantification of the half-life of the A138 pause in absence and presence of 50 nM NusA for the wild-type (WT) and mutant (Stem mutant) construction.
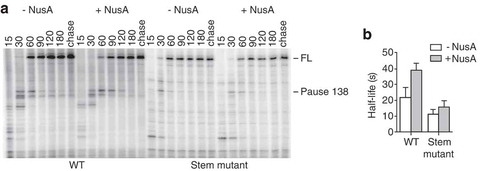
Impact of the A138 stem-loop structure on thiC riboswitch transcription and activity
To determine if the stability of the A138 stem-loop is important in the context of the thiC riboswitch for transcriptional pausing at position A138, we performed kinetics of transcription elongation () by using a version of the riboswitch containing the same mutations as those used in the stem mutant (). Analysis of transcription reactions data showed that the half-life of the A138 pause site in the mutant was reduced by ~2.7-fold in absence of TTP and ~2.0-fold in presence of TPP compared to the wild-type (), Table S1, Rows 11, 12, 15 and 16), a result consistent with the stability of the A138 stem-loop being important for transcriptional pausing. The half-life of the C158 pause was also reduced by ~2.3-fold and ~1,8-fold in absence and presence of TPP, respectively (Table S1, Rows 13, 14, 17 and 18), suggesting that A138 and C158 pauses might be interdependent and/or potentially controlled by the same RNA signal. On the other hand, we were unable to characterize the pause at position C187 when transcriptions reactions were performed under the same conditions.
Figure 4. Importance of the A138 stem-loop for riboswitch transcriptional pausing.
(A) Transcription kinetics experiments performed on the wild-type and stem mutant thiC riboswitch using 25 µM NTP, in absence and presence of 10 µM TPP. Pause sites A138, C158 and C187, and full-length product (FL) are indicated on the right, and sampling times are indicated on top of the gel. (B) Quantification of the half-life of the A138 transcriptional pause in the context of the wild-type and stem mutant thiC riboswitch, in absence and presence of 10 µM TPP.
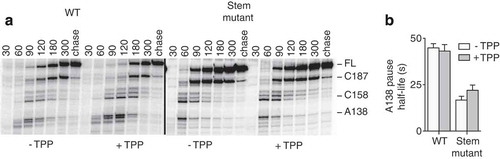
Following RNase H cleavage assays performed on the mutated riboswitch (), we obtained a Kswitch value of 1454 ± 110 nM (), which represents a decrease of ~2.2-fold in affinity when compared to the wild-type riboswitch (), -NusA). These data are consistent with a model where the stability of the A138 stem-loop is important for transcriptional pausing at A138 and for the riboswitch ligand sensing. To determine the effect of the mutant in an in vivo context, transcriptional lacZ chromosomal E. coli constructs including the thiC riboswitch sequence and the first 10 codons of the thiC open reading frame (ORF) were designed. In the presence of 1 mM TPP, the β-galactosidase activity was repressed by 38% for the wild-type construct and 52% for the stem mutant construct ().
Figure 5. Effect of the A138 stem-loop on riboswitch TPP sensing.
(A) Kswitch determination experiment using the SD probe[Citation9]. In vitro transcription experiments (1 mM NTP) were performed using increasing TPP concentrations ranging from 100 pM to 100 µM. The full length (FL) and cleavage products (P) are indicated on the right of the gel. (B) Quantification analysis of experiments shown in (A), yielding a Kswitch value 1454 ± 110 nM. (C) β-Galactosidase assays of transcriptional thiC-LacZ (trX) fusions for the wild-type and stem mutant. Values were normalized to the activity obtained for the wild-type construct in absence of TPP. The average values of two independent experiments consisting of three technical replicates each, with standard deviations, are shown.
![Figure 5. Effect of the A138 stem-loop on riboswitch TPP sensing.(A) Kswitch determination experiment using the SD probe[Citation9]. In vitro transcription experiments (1 mM NTP) were performed using increasing TPP concentrations ranging from 100 pM to 100 µM. The full length (FL) and cleavage products (P) are indicated on the right of the gel. (B) Quantification analysis of experiments shown in (A), yielding a Kswitch value 1454 ± 110 nM. (C) β-Galactosidase assays of transcriptional thiC-LacZ (trX) fusions for the wild-type and stem mutant. Values were normalized to the activity obtained for the wild-type construct in absence of TPP. The average values of two independent experiments consisting of three technical replicates each, with standard deviations, are shown.](/cms/asset/d9b20d0a-3047-40b1-a423-a3bf2cbe4b8e/krnb_a_1616354_f0005_oc.jpg)
Structural analysis of the thiC riboswitch elongation complex at the A138 pause site
To probe the structure of the thiC riboswitch when RNAP is paused at position A138, we performed in-line probing assays on transcriptional complexes stalled at the A138 pause site using a biotin-streptavidin complex () [Citation9]. Control experiments showed that elongation complexes were stably formed during the in-line probing assays (Fig. S1), thus ensuring that probing data were obtained from intact transcriptional complexes. Upon TPP binding, variations in nucleotide flexibility were observed for positions A15, G33, G52, U60, C68, U93, U97 and C128, consistent with the formation of a TPP-riboswitch complex [Citation23]. Importantly, no change in probing was observed in the A138 pause hairpin (), suggesting that this region is not involved in the TPP-induced conformational change. This result is consistent with the hypothesis that the loss of riboswitch ligand affinity observed in the stem mutant () is due to a change in the A138 pause half-life rather than to a structural change caused by the mutation. However, we cannot exclude that the mutation causes undesirable structural rearrangements (see Discussion).
Figure 6. In-line probing assays of EC-138.
In-line probing of the thiC riboswitch in absence (-) or presence (+) of 10 µM TPP. Reactions were either quenched immediately (non-treated, NT) or after 40 hours (in-line probing, IL). Regions showing significant cleavage changes are indicated to the right. The determination of in-line probing cleavage sites was performed using 3ʹ-OMe NTPs in the transcription reaction (lanes A, U, C and G) The A138 stem-loop region is shown in the box.
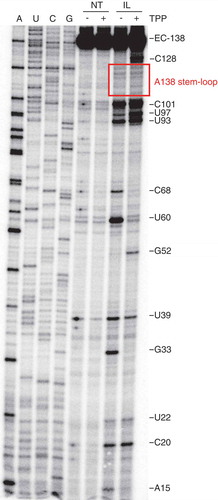
Discussion
In this work, we have characterized transcriptional pausing occurring within the expression platform of the E. coli thiC riboswitch. We have shown that the transcription factor NusA enhances all three riboswitch transcriptional pause sites in the absence of TPP (). Since NusA has mainly been shown to modulate RNAP pausing in the presence of nascent stem-loop structures [Citation5,Citation14,Citation15,Citation21], it suggests that pausing at positions A138, C158 and C187 is modulated at least in part by RNA structural domains such as RNA hairpins that would form inside the RNA exit channel of the RNAP, leading to pausing [Citation21,Citation25]. Interestingly, NusA also increased transcriptional pausing at C187 only in absence of TPP (), suggesting that the TPP-induced pausing at this position is due to a NusA-independent feature [Citation9]. In addition, we report here that NusA allowed the thiC riboswitch to sense TPP at lower concentrations (). These results suggest that the presence of NusA allows more time for the riboswitch to bind TPP within a narrow transcriptional window located upstream of C187.[Citation9] Therefore, in addition to the B. subtilis ribD and pbuE riboswitches, the thiC riboswitch constitutes the third occurrence for which the riboswitch metabolite sensing activity is affected by the transcription factor NusA [Citation6,Citation7]. Interestingly, while the downstream limit of the operational window of these two examples is the formation of a terminator hairpin, it is the formation of the anti-P1 stem that constitutes the same limit in the thiC riboswitch. While regulation of thiC is partly transcriptional (Rho-dependent), the fact that the anti-P1 stem constitutes the end of the transcriptional window suggests that the kinetic mode of regulation might not be limited to transcriptional riboswitches only.
We have determined here that the presence of a stem-loop comprising residues 105–126 (the A138 stem-loop) was involved in RNAP pausing at position A138. The NusA-dependent increase of transcriptional pausing by ~4-fold at position A138 both in the absence and presence of TPP () [Citation11] suggests that NusA stabilizes the A138 stem-loop in the context of a transcriptional complex paused at this position. Transcriptional pausing at position A138 was observed when using a region corresponding to positions 87–155, suggesting that the stem-loop is sufficient to induce pausing at position A138. Furthermore, we found that destabilizing the stem-loop decreased the pause half-life by ~1.9-fold and ~2.7-fold in the context of the isolated stem-loop () and the riboswitch (), respectively, suggesting that the integrity of the stem-loop is important for the half-life of the pause at position A138. The fact that the mutation did not affect regulation in vivo () could be due to a multitude of factors, one of which being the high concentration of TPP (1 mM) used in the in vivo experiments. Since the TPP concentration used was substantially higher than the observed Kswitch value, relatively subtle effects of transcriptional pausing might not be detectable under these conditions.
Given that transcriptional pausing was found to be unaffected by the loop size of a stem-loop but strongly affected by the stability of the stem in the his leader region [Citation26,Citation27], the reduced half-life of the A138 pause might primarily be due to the introduction of mutations destabilizing the stem. However, the stem-loop mutant is predicted to be less stable than the his leader region stem-loop due to a fewer number of base pairs and the presence of a C-bulge (). We speculate that, in the case of a weaker stem, the loop size may have a stronger influence on the formation of the stem-loop. Further experiments will be required to determine if other secondary structure elements – such as those in the aptamer domain – are important for transcriptional pausing at A138.
The thiC riboswitch metabolite sensing and regulatory activity were previously found to occur cotranscriptionally [Citation9], suggesting that the fate of gene expression is determined during a short time window. In agreement with this, we found that the rate of transcription elongation is important for TPP sensing [Citation9]. The results reported here extend these previous results by establishing that the rate of transcription elongation is controlled by the presence of transcriptional pausing within the sensing window by the presence of pause sites A138 and C158, which are in turn modulated by the presence of NusA. The stability of the A138 stem-loop was found to be particularly important since a riboswitch containing a mutated stem-loop required ~2.2-fold more TPP to undergo cotranscriptional folding (). An alternative explanation could be that the disruption of the stem could favour the formation of the TPP-free structure (). However, several lines of evidence suggest that it is unlikely to be the case. First, the A138 stem-loop is predicted to be formed in the TPP-free as well as TPP-bound structures. In addition, no change in probing was reported for this region upon TPP binding. These two observations are consistent with the hairpin not being involved directly in riboswitch conformational change. Thus, these lines of evidences suggest that the stem mutation does not affect the riboswitch structure.
Although no direct experimental results have been obtained to show that transcriptional pausing at C158 is important for TPP sensing, the fact that this pause is located in the binding window suggests that this pause is important for TPP recognition. Since no hairpin upstream of the C158 position is apparent from the thiC ON or OFF structure (), further work will be required to dissect which part(s) of the riboswitch is involved in C158 transcriptional pausing, thus allowing to determine the importance of the pause site in the riboswitch metabolite sensing activity.
Overall, our data demonstrate that transcriptional pausing is important for the E. coli thiC riboswitch activity and suggests that this effect is mediated by the NusA transcription factor. It has previously been observed that E. coli riboswitches exhibit several transcriptional pause sites in their expression platform [Citation9–Citation11]. Further work will be required to elucidate to which extent E. coli riboswitches rely on transcriptional pausing to modulate metabolite sensing and genetic control, and whether such pause sites may be involved in additional cellular mechanisms [Citation28].
Materials and methods
DNA oligonucleotides and bacterial strains
DNA oligonucleotides were purchased from Integrated DNA Technologies. Strains used in this study are derived from E. coli MG1655, described previously [Citation29]. Strain genotypes are listed in Table S3. The thiC transcriptional fusions, containing the riboswitch domain with the first 10 codons of the ThiC ORF, were constructed by inserting a PCR product into pFRΔ. The PCR strategy is described in Table S4 and the oligonucleotides are described in Table S5. Mutations in thiC were introduced using a three step PCR strategy. Briefly, PCR1 and PCR2 were performed using genomic DNA and PCR3 was performed using PCR1 and PCR2 products. PCR products were ligated into EcoRI/BamHI-digested pFRΔ using NE Builder HiFi DNA Assembly and transformed into EC100 Chemically Competent E. coli using the NE Builder HiFi DNA Assembly Transformation Protocol, selecting for ampicillin resistance (100 µg/mL). Insertion of pfrΔ-PLacUV5-thiC10cd-LacZ (WT and stem mutant) into the bacterial chromosome of MG1655 was achieved by delivering a single copy of the thiC transcriptional fusion at the λatt site [Citation30]. Stable lysogens were screened for single insertion by PCR reaction [Citation31]. All obtained lacZ fusions were sequenced to ensure the integrity of the constructs.
Single-round in vitro transcription assays
The PCR strategy to obtain DNA templates used for transcription reactions is described in Table S2. Oligonucleotides are described in Table S5. The full-length thiC riboswitch containing the stem-loop destabilizing mutations was prepared using a three-step PCR strategy. Briefly, PCR1 and PCR2 were performed using genomic DNA and PCR3 was performed using PCR1 and PCR2 products. In vitro transcriptions were performed in 20 mM Tris-HCl pH 8.0, 20 mM MgCl2, 20 mM NaCl, 14 mM 2-mercaptoethanol and 0.1 mM EDTA. The DNA template (200 nM), sigma70 factor (200 nM) and RNAP (100 nM) were incubated at 37°C for 5 min. The corresponding trinucleotide (UAA for the riboswitch and CAA for the synthetic stem-loop, both purchased from IBA Lifesciences) (10 µM), a set of two unlabelled nucleotides (CTP/GTP for the whole riboswitch and ATP/GTP for the synthetic stem-loop) (2.5 µM) and [α-32P] UTP (0.3 µCi/µL) were then added and the reaction incubated at 37°C for 8 min, yielding synchronized halted complexes stalled at position 14 for the riboswitch and at position 11 for the synthetic stem-loop. The sample was then filtered through a G50 column to remove free nucleotides. Transcription reactions were completed by adding all four nucleotides (25 µM or 100 µM) with heparin (0.45 mg/mL) to allow only one round of transcription. When needed, TPP and NusA were added at 10 µM and 50 nM, respectively. Aliquots were taken at indicated times. A chase reaction was done by adding 1 mM NTP for an additional 5 min at the end of the time course experiment. The half-life of transcriptional pausing was found by determining the fraction of each RNA species compared with the transcription read through for each time point, which was then analysed with pseudo-first-order kinetics [Citation32]. For each determination, the background signal was subtracted. The experiments were performed at least two times and the average values with standard deviations are reported. Images have been cropped for presentation.
RNase H cleavage assays
The PCR strategy to obtain DNA templates used for transcription reactions is described in Table S2. Transcriptions were performed using 1 mM NTP for 5 min to ensure the completion of the transcription reaction and increasing TPP concentrations (from left to right on the gels: No TPP, 100 pM, 250 pM, 500 pM, 1 nM 2.5 nM, 5 nM, 10 nM, 25 nM, 50 nM, 100 nM, 250 nM, 500 nM, 1 µM, 2.5 µM, 5 µM, 10 µM, 25 µM, 50 µM, 100 µM). The different transcription reactions were mixed separately with 20 µM DNA oligonucleotides for 5 min to allow hybridization with the RNA target. RNase H cleavage was initiated by adding an equal volume of a solution of RNase H (0.12 U/µL) in 5 mM Tris-HCl, pH 8.0, 20 mM MgCl2, 100 mM KCl, 50 mM EDTA and 10 mM 2-mercaptoethanol at 37°C for 5 min. Reactions were stopped by adding an equal volume of a stop solution (95% formamide, 20 mM EDTA and 0.4% SDS). Images have been cropped for presentation.
β-galactosidase assays
Kinetic assays for β-galactosidase experiments were performed as described previously [Citation9,Citation33–Citation35]. Briefly, an overnight bacterial culture grown in M63 0.2% glucose with 1 µM FeSO4 minimal medium at 37 °C were diluted to an OD600 of 0.03 in 50 ml of fresh medium. The culture was incubated at 37 °C until an OD600 of 0.1 was obtained. TPP (1 mM) was then added when indicated. Vmax were measured using a BioTek Synergy™ HT multi-mode microplate reader. Specific activities were calculated using the formula Vmax/OD600. Each specific β-galactosidase activity has been relativized to the specific activity of the WT construct obtained in the absence of TPP. The experiments, consisting of three technical replicates, were performed in duplicate and the average values with standard deviations (s.d.) are reported.
In-line probing assays
To stall the transcription complexes at the desired position, we used DNA templates containing a biotin moiety inserted by PCR at the 5ʹ end of the template strand [Citation9]. Prior to transcription, the modified DNA template was incubated with streptavidin with a 1:2.5 ratio to ensure that all DNA templates are bound to streptavidin. The biotin-streptavidin acts as a roadblock for the RNAP, blocking its progression with no release or dissociation of the transcription complex. Transcription of the streptavidin-bound DNA templates was performed as described above to form the EC stalled at the corresponding position. Then, the reaction was either stopped (non-treated) or incubated at room temperature for 40 hours for the in-line probing reaction. For control experiments presented in Supplementary Figure 1, reactions were performed on avidin beads [Citation36], then the non-treated or the in-line probing reactions were submitted to separation between the beads and the supernatant, then stopped by adding an equal volume of a stop solution (95% formamide, 20 mM EDTA and 0.4% SDS).
Supplemental Material
Download Zip (136.5 KB)Acknowledgments
We thank members of the Lafontaine laboratory for discussion and for critical reading of the manuscript.
Disclosure statement
No potential conflict of interest was reported by the authors.
Supplementary material
Supplemental data can be accessed here.
Additional information
Funding
References
- Serganov A, Nudler E. A decade of riboswitches. Cell. 2013;152:17–24.
- Mccown PJ, Corbino KA, Stav S, et al. Riboswitch diversity and distribution. Rna. 2017;23:995–1011.
- Montange RK, Batey RT. Riboswitches: emerging themes in RNA structure and function. Annu Rev Biophys. 2008;37:117–133.
- Pan T, Sosnick T. RNA folding during transcription. Annu Rev Biophys Biomol Struct. 2006;35:161–175.
- Zhang J, Landick R. A two-way street: regulatory interplay between RNA polymerase and nascent RNA structure. Trends Biochem Sci. 2016;41:293–310.
- Wickiser JK, Winkler WC, Breaker RR, et al. The speed of RNA transcription and metabolite binding kinetics operate an FMN riboswitch. Mol Cell. 2005;18:49–60.
- Lemay JF, Desnoyers G, Blouin S, et al. Comparative study between transcriptionally- and translationally-acting adenine riboswitches reveals key differences in riboswitch regulatory mechanisms. PLoS Genet. 2011;7:e1001278.
- Hollands K, Proshkin S, Sklyarova S, et al. Riboswitch control of Rho-dependent transcription termination. Proc Natl Acad Sci U S A. 2012;109:5376–5381.
- Chauvier A, Picard-Jean F, Berger-Dancause J-C, et al. Transcriptional pausing at the translation start site operates as a critical checkpoint for riboswitch regulation. Nat Commun. 2017;8:13892.
- Perdrizet GA, Artsimovitch I, Furman R, et al. Transcriptional pausing coordinates folding of the aptamer domain and the expression platform of a riboswitch. Proc Natl Acad Sci. 2012;109:3323–3328.
- Guedich S, Puffer-Enders B, Baltzinger M, et al. Quantitative and predictive model of kinetic regulation by E. coli TPP riboswitches. RNA Biol. 2016;13:373–390.
- Wong TN, Pan T. RNA folding during transcription: protocols and studies. Methods Enzym. 2009;468:167–193.
- Artsimovitch I, Landick R. Pausing by bacterial RNA polymerase is mediated by mechanistically distinct classes of signals. Proc Natl Acad Sci U S A. 2000;97:7090–7095.
- Sen R, Chalissery J, Qayyum MZ, et al. Nus factors of Escherichia coli. EcoSal Plus. 2013. DOI:10.1128/ecosalplus.4.5.3.1
- Yakhnin AV, Babitzke P. NusA-stimulated RNA polymerase pausing and termination participates in the Bacillus subtilis trp operon attenuation mechanism invitro. Proc Natl Acad Sci U S A. 2002;99:11067–11072.
- Mondal S, Yakhnin AV, Sebastian A, et al. NusA-dependent transcription termination prevents misregulation of global gene expression. Nat Microbiol. 2016;1:15007.
- Pan T, Artsimovitch I, Fang XW, et al. Folding of a large ribozyme during transcription and the effect of the elongation factor NusA. Proc Natl Acad Sci U S A. 1999;96:9545–9550.
- Toulokhonov I, Artsimovitch I, Landick R. Allosteric control of RNA polymerase by a site that contacts nascent RNA hairpins. Science. 2001;292:730–733.
- Shankar S, Hatoum A, Roberts JW. A transcription antiterminator constructs a NusA-dependent shield to the emerging transcript. Mol Cell. 2007;27:914–927.
- Ha KS, Toulokhonov I, Vassylyev DG, et al. The NusA N-terminal domain is necessary and sufficient for enhancement of transcriptional pausing via interaction with the RNA exit channel of RNA polymerase. J Mol Biol. 2010;401:708–725.
- Guo X, Myasnikov AG, Chen J, et al. Structural basis for NusA stabilized transcriptional pausing. Mol Cell. 2018;69:802–819.e1.
- Begley TP, Downs DM, Ealick SE, et al. Thiamin biosynthesis in prokaryotes. Arch Microbiol. 1999;171:293–300.
- Winkler W, Nahvi A, Breaker RR. Thiamine derivatives bind messenger RNAs directly to regulate bacterial gene expression. Nature. 2002;419:952–956.
- Chauvier A, Lafontaine D. Probing of nascent riboswitch transcript. In: DNA-protein interactions: principles and protocols. 4th ed. Humana Press. 2015. p. 1–347. https://www.springer.com/cn/book/9781493928767
- Kang JY, Mishanina TV, Bellecourt MJ, et al. RNA polymerase accommodates a pause RNA hairpin by global conformational rearrangements that prolong pausing. Mol Cell. 2018;69:802–815.
- Chan CL, Landick R. Dissection of the his leader pause site by base substitution reveals a multipartite signal that includes a pause RNA hairpin. J Mol Biol. 1993;233:25–42.
- Landick R, Yanofsky C. Stability of an RNA secondary structure affects in vitro transcription pausing in the trp operon leader region. J Biol Chem. 1984;259:11550–11555.
- Larson MH, Mooney RA, Peters JM, et al. A pause sequence enriched at translation start sites drives transcription dynamics in vivo. Science. 2014;344:1042–1047.
- Massé E, Gottesman S. A small RNA regulates the expression of genes involved in iron metabolism in Escherichia coli. Proc Natl Acad Sci U S A. 2002;99:4620–4625.
- Simons RW, Houman F, Kleckner N. Improved single and multicopy lac-based cloning vectors for protein and operon fusions. Gene. 1987;53:85–96.
- Powell BS, Courtl DL, Nakamura3 Y, et al. Rapid confirmation of single copy lambda prophage integration by PCR. Nucleic Acid Research. 1994;22:5765–5766.
- Landick R, Wang D, Chan CL. Quantitative analysis of transcriptional pausing by Escherichia coli RNA polymerase: his leader pause site as paradigm. Methods Enzymol. 1996;274:334–353.
- Caron M-P, Bastet L, Lussier A, et al. Dual-acting riboswitch control of translation initiation and mRNA decay. Proc Natl Acad Sci U S A. 2012;109:E3444–53.
- Prévost K, Salvail H, Desnoyers G, et al. The small RNA RyhB activates the translation of shiA mRNA encoding a permease of shikimate, a compound involved in siderophore synthesis. Mol Microbiol. 2007;64:1260–1273.
- Zhou Y, Gottesman S. Regulation of proteolysis of the stationary-phase sigma factor RpoS. J Bacteriol. 1998;180:1154–1158.
- Nudler E, Gusarov I, Bar-Nahum G. Methods of walking with the RNA polymerase. Methods Enzymol. 2003;371:160–169.