ABSTRACT
Mammalian fertility is reduced during heat exposure in the summer, but is regained as temperatures decrease in the autumn again. However, the mechanism underlying the phenomenon remains unknown. We investigated heat stress tolerance of germ cells and their surrounding somatic cells, and discovered that microRNA ssc-ca-1 was upregulated after heat stress in cultured porcine granulosa cells (GCs), but not in serum-starved GCs. Ssc-ca-1 inhibited heat shock protein 70 (Hsp70) expression through its 3′- and 5′-UTRs. Although Hsp70 mRNA transcription was induced in GCs by in vivo exposure to heat in the summer, ssc-ca-1 inhibited Hsp70 expression. In ovarian cultures, heat stress-induced Hsp70 expression in primordial but not in growing follicles; ssc-ca-1 expression did not change in primordial follicles, but increased in growing follicles. Consistently, ssc-ca-1 was present in testicular cells and exhibited the same function as in ovarian cells. It modulated the different Hsp70 expression between spermatogonial stem cells and Sertoli cells after scrotal heat stress. This mechanism is of relevance to mammalian fertility in parts of the world dominated by heat stress associated with global climate change.
Introduction
In most mammals, including humans, normal folliculogenesis and spermatogenesis require suitable environmental conditions. Low fertility in the summer in female [Citation1] and male mammals [Citation2] has been well documented, which is related to seasonal changes in temperature, and detrimental consequences arise from global warming. Heat stress caused by high summer temperatures impairs folliculogenesis, leading to the ovulation of low-quality oocytes [Citation3]. As temperatures drop in the autumn, functional oocyte development is recovered in two to three oestrous cycles after heat stress [Citation4]. Resumption of fertility in female mammals during the autumn indicates that the quiescent primordial follicle, which represents the animal’s lifetime reproductive capacity, is generally resistant to heat-induced damage, thereby protecting the capacity for future cyclic recruitment of follicles and development of oocytes.
Similarly to what happens in females, heat stress has a detrimental effect on the spermatogenesis in male mammals [Citation2]. The observation that germ cell populations are removed after testicular hyperthermia and subsequently, spermatogenesis is gradually recovered [Citation5], implies spermatogonial stem cells (SSCs) survive heat stress. However, the mechanisms underlying the relatively higher tolerance of heat stress by quiescent primordial follicles and SSCs are not clear, and whether resilience can be expected under higher and more longer-lasting temperature challenges is unknown.
Female and male germ cells primarily depend on respectively granulosa cells (GCs) and Sertoli cells (SCs), which are somatic cell types close to germ cells. GCs are the central, essential coordinators of folliculogenesis. They synthesize and secrete a wide variety of hormones and cytokines to regulate follicular growth. When the GCs surrounding an oocyte undergo apoptosis, follicular atresia is initiated and both the somatic and germ cell compartments are lost [Citation6]. Exposure to heat induces GC apoptosis in female rats and attenuates estrogenic activity of growing follicles [Citation7]. Meanwhile, SCs are essential for testicular development, spermatogenesis, and formation of the blood-testis barrier. Heat-induced changes in SCs lead to reversible disruption of this barrier and subsequent failure of spermatogenesis in male monkeys [Citation8]. It is suggested that mammals subfertility in the summer may be due to the ovarian and testicular dysfunction caused by a heat stress–induced decrease in the number or function of GCs and SCs.
Cells respond to stress by undergoing adaptive changes that increase their ability to tolerate normally sublethal conditions. Heat shock proteins 70 (HSP70s) mediate stress tolerance by preventing misfolding and aggregation of nascent polypeptides, facilitating protein folding. They are also powerful antiapoptotic proteins, blocking apoptosis at several different levels. The immediate early induction of Hsp70 protects cells from heat-induced apoptosis [Citation9]. Under heat stress conditions, Hsp70 expression levels increase, either due to posttranscriptional mRNA stabilization or posttranslational modification [Citation10–Citation12]. A novel class of 21- to 25-nucleotide, single-stranded RNA molecules, named microRNA, has been implicated in negative posttranscriptional regulation of gene expression by binding to the three prime untranslated region (3ʹ-UTR) of the target mRNA [Citation13].
The major stress-inducible HSP70s are HspA1A and HspA1B. They only differ by two amino acids and are collectively termed Hsp70 [Citation9]. Through binding to the HspA1A 3ʹ-UTR, hsa-miR-223 can regulate Hsp70 protein levels, thus modulating osteosarcoma cell apoptosis under cisplatin stress [Citation14]. Decreased expression of HspA1B-targeting microRNAs (miR-378* and miR-711) contributes to Hsp70 upregulation in mouse myocardium subjected to ischemic stress [Citation15], and could potentially protect the heart cells against stress-induced damage. It is suggested that microRNA functioning in the stress response depends on the stress type and tissue; however, Hsp70-targeting microRNA in ovarian or testicular tissues subjected to heat has not been studied until now.
Based on these observations, we hypothesized that the difference in heat stress tolerance of quiescent primordial and growing follicles is related to differences in posttranscriptional regulation of Hsp70 by microRNA in GCs, and that the same mechanism exists in the testis. To investigate this, we exposed in vitro cultures of porcine GCs, primordial and growing follicles to heat stress and assessed the posttranscriptional regulation of Hsp70 expression by different microRNAs. We also looked at the seasonal variations of these molecules in ovaries collected from sows in the summer and autumn. Finally, we validated the functions of these microRNAs in testicular cells from male piglets after scrotal heating.
Results
Dicer modulates Hsp70 expression in granulosa cells after exposure to lethal heat stress
To establish a cellular model of heat-induced apoptosis, we assessed the effect of heat stress on the expression of Hsp70 and markers of apoptosis in porcine GCs in vitro. Exposure to 47°C for 2 h was lethal for GCs, whereas exposure to a slightly lower temperature (42°C) for 2 h was sublethal. Both sublethal and lethal heat stress-induced Hsp70 expression in cultured GCs, but Hsp70 levels were higher after sublethal heat stress compared with those in control cells or in cells exposed to lethal heat stress (). After exposure to lethal heat stress followed by 24 h of recovery at 37°C, phosphorylation of proapoptotic BimEL protein was induced and the expression of antiapoptotic Bcl-2 was downregulated (). Twelve hours after exposure to lethal heat stress, mean caspase-3 activity was significantly increased (). Overexpression of Hsp70 in GCs significantly attenuated the effect of lethal heat stress on caspase-3 activation ( and s1). Collectively, overexpression of stress-inducible Hsp70 protected cells from lethal heat stress–induced apoptotic changes. Lethal heating induced phosphorylation of c-Jun N-terminal kinase (JNK) protein, and treatment with a JNK-specific inhibitor (SP600125) inhibited BimEL phosphorylation (). Expression of BimEL, Bcl-2 and caspase-3 activity did not change after cells were exposed to sublethal heat stress ( and ).
Figure 1. Exposure to lethal heat stress induces expression and activity of apoptotic markers in granulosa cells in vitro.
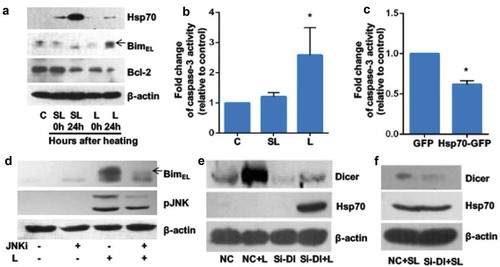
Recent studies have shown that microRNAs regulate cellular behaviour under stress or disease conditions [Citation16]. The expression of Dicer, a ribonuclease that plays a central role in mature microRNA production, increased following lethal heat stress (, lane 2 versus lane 1). To test the hypothesis that microRNAs target Hsp70 mRNA in GCs under heat stress, we used small interfering RNA (siRNA) to knock down DICER and measured Hsp70 levels in GCs. Knockdown of DICER led to upregulation of Hsp70 expression 24 h after lethal heat stress (, lane 4 versus lane 3, and S2). There was no effect of DICER knockdown on sublethal heat stress–induced Hsp70 expression (). These data suggest that microRNAs play a vital role, either directly or indirectly, in the suppression of Hsp70 expression in response to lethal heat stress, but not to sublethal heat stress.
Lethal heat stress increases microRNA expression to suppress Hsp70 expression
To identify the microRNAs that regulate Hsp70 expression, we performed small RNA deep sequencing of GCs after exposure to the regular culture temperature (37°C), sublethal heat stress, or lethal heat stress. edgeR analysis showed there were no significant differences in baseline expression levels of known porcine microRNAs among the three treatment groups ( and s3). Compared with control cells and cells exposed to sublethal heat stress, cells exposed to lethal heat stress showed upregulation of four novel microRNAs (ssc-ca-1, −2, −3 and −4) (). Overexpression of ssc-ca-1 or −3 in GCs inhibited upregulation of Hsp70 expression after exposure to sublethal heat stress (). Conversely, ssc-ca-1 or −3 inhibition led to an increase in Hsp70 expression in GCs after lethal heat stress ().
Table 1. Normalized expression of newly identified microRNAs in granulosa cells after exposure to normal temperature, sublethal heat stress, or lethal heat stress
Figure 2. Lethal heat stress increases microRNA expression to suppress Hsp70 expression
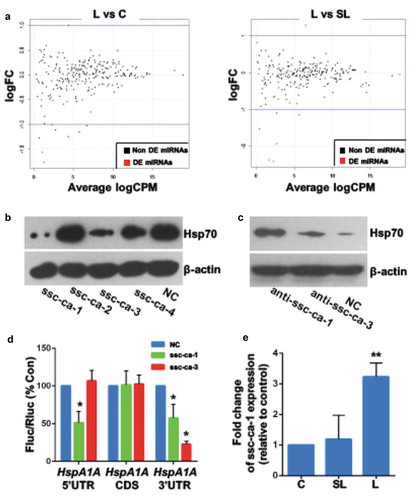
To determine whether microRNAs have a direct effect on heat stress–induced Hsp70 expression, we inserted the 5′-UTR, coding sequence, or 3′-UTR of the porcine HspA1A gene into a luciferase reporter construct. Cotransfection of GCs with ssc-ca-1 and the HspA1A 5′- or 3′-UTR chimeric constructs resulted in a significantly reduced mean luciferase activity relative to cells cotransfected with control microRNA (). Cotransfection of GCs with ssc-ca-3 and the HspA1A 3′-UTR chimeric construct resulted in a lower mean luciferase activity relative to cells cotransfected with control microRNA. Quantitative RT-PCR of ssc-ca-1 microRNA confirmed its upregulation in GCs after exposure to lethal heat stress (). These data suggest that ssc-ca-1 regulates Hsp70 expression through the 3′- and 5′-UTR of HspA1A, whereas ssc-ca-3 regulates Hsp70 expression through the 3′-UTR only.
Resistance to heat-induced apoptosis in serum-starved cells is increased by inhibition of ssc-ca-1 expression
Previous studies demonstrated that Dicer expression was downregulated upon serum withdrawal in human umbilical vein endothelial cells [Citation17]. We compared the difference in Dicer expression between serum-starved and control (10% FBS) GCs, and the results showed that Dicer protein expression was decreased in serum-starved GCs compared with control GCs (). Immunoblotting () and Immunocytochemistry () confirmed elevated Hsp70 expression in serum-starved GCs after lethal heat stress, suggesting that Hsp70-targeting microRNAs are involved in the increase in stress-induced Hsp70 expression in serum-starved GCs. The mean expression of ssc-ca-1 was significantly downregulated in serum-starved GCs, and exposure to lethal heat did not induce this expression (). We also compared the difference in heat stress tolerance between serum-starved and control GCs. Flow cytometric analysis demonstrated that a smaller proportion of serum-starved GCs underwent heat stress–induced apoptosis than proliferative GCs did ( and ). These data support the hypothesis that in serum-starved cells, lethal heating reduces Dicer expression, which inhibits ssc-ca-1 expression. This, in turn, upregulates Hsp70 expression and increases the cells’ tolerance of heat.
Figure 3. Serum starvation protects granulosa cells from heat-induced apoptosis
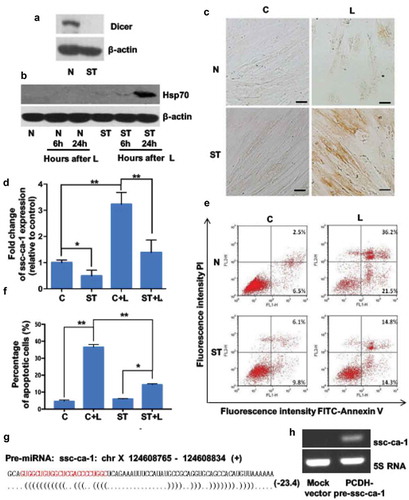
ssc-ca-1 is located on X chromosome and is conserved across pig breeds
The microRNAs are transcribed from the genome as long primary transcripts (pri-microRNA); excision of hairpin regions to form a 60–80 nt precursor (pre-microRNA) followed by cleavage yield approximately 22-nt RNA duplexes [Citation13]. miRanalyzer software [Citation18] predicted that the precursor of ssc-ca-1 is encoded on chromosome X 124,608,765–124,608,834 (+) in the porcine genome and folds into a stem-loop structure (). We used RT-PCR with specific primers to detect the expression of the chromosome X pre-ssc-ca-1 locus in GCs. The expected RT-PCR amplification product was confirmed by sequencing. RT-PCR results for the total RNA sample showed the PCR products of pre-microRNA and pri-microRNA, but the results for small RNAs (<200 nt) showed only the PCR product of the precursor (Figure S4). These results indicate that the locus on chromosome X was transcribed and might produce ssc-ca-1.
We then tested whether the genomically predicted stem-loop region including pre-ssc-ca-1 is processed to mature ssc-ca-1 by transiently expressing its precursor in 293T cells. The mature form of ssc-ca-1 was detected in the transfected cells, but was absent in cells transfected with a mock vector (). Lethal heating for 0.5 h significantly downregulated the expression of the ssc-ca-1 transcript (pri-ssc-ca-1) (Figure S5). Subsequently, the pri-ssc-ca-1 were cleaved into mature microRNA, and ssc-ca-1 expression was upregulated in GCs exposed to lethal heat for 1 h (Figure S6). These data confirmed that ssc-ca-1 is a product of the genomic locus described earlier.
Next, we analysed whole-genome resequencing data of 180 pigs from 16 different breeds, both wild and domestic, using the neighbour-joining method. The results showed that the gene coding for ssc-ca-1 was highly conserved among different breeds, except for one primitive boar (Sus verrucosus) (Figure S6). In addition, the gene encoding its precursor was highly conserved among different breeds, except in three primitive boars (Sumatran, S. barbatus and S. verrucosus) (Figure S7).
Primordial follicles are more heat stress tolerant than growing follicles
In a previous study, 41.5°C was the upper limit of the physiological range for ovary function [Citation19]. Therefore, we removed ovaries from prepubertal gilts and cultured them at 41.5°C for 2 h. Compared with growing follicles from ovaries cultured at 37°C, growing follicles from the heat-stressed ovaries appeared damaged (), with a significantly higher mean percentage of dead oocytes (). In contrast, primordial (quiescent) follicles were relatively resistant to the heat stress culture condition ( and ). Immunoblot assays confirmed that Hsp70 expression was not induced by heat stress in growing follicles, but was strongly activated by heat stress in primordial follicles ( and s8). Dicer expression in mouse primordial follicles is lower than that in growing follicles [Citation20]. Immunoblot analysis of follicles isolated from porcine ovaries confirmed lower Dicer expression in primordial follicles than in growing follicles (), suggesting that microRNAs mediate the response to heat stress in growing follicles. For growing follicles, mean ssc-ca-1 expression was significantly higher after exposure to 41.5°C than after exposure to 37°C (). By contrast, there was no difference in mean ssc-ca-1 expression in primordial follicles cultured at either 41.5°C or 37°C (). Together, these data suggested that primordial follicles are more tolerant of heat stress and express lower levels of heat stress–induced ssc-ca-1 than growing follicles.
Figure 4. Primordial follicles are more tolerant of heat stress than growing follicles
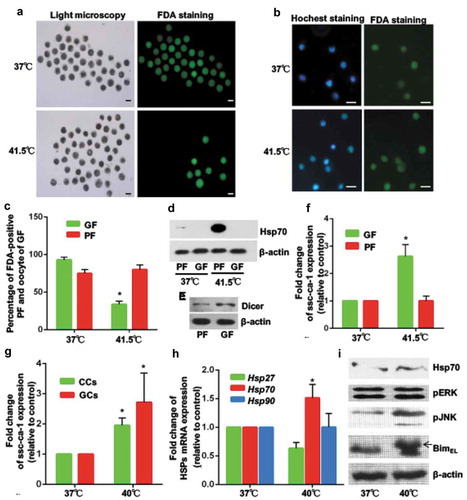
A temperature of 40°C is within the range of rectal temperatures observed in pigs subjected to heat stress [Citation21]. To assess changes in ssc-ca-1 expression in GCs of growing follicles exposed to physiologically relevant hyperthermia, ovaries were exposed to 40°C for 2 h. Compared with cells from ovaries cultured at 37°C, mean ssc-ca-1 expression was significantly higher in GCs and cumulus cells (). Although Hsp70 transcript levels were significantly higher in heat-treated GCs than in control GCs (), we did not observe any differences in Hsp70 protein levels between the two groups (). Similar to what we observed in heat-treated GCs cultured in vitro ( and ), expression of JNK-induced BimEL phosphorylation was upregulated in GCs from ovarian tissues subjected to heat stress (), which indicated that heat stress damages growing follicles.
Higher ssc-ca-1 levels in granulosa cells of growing follicles in vivo during the summer
The molecular effect of temperature on GCs of growing follicles in vivo was examined by collecting pig ovaries in the summer (average temperature in Beijing, China in July and August 2014: 27.1°C) and in the autumn (average temperature in Beijing in September and October 2014: 17.6°C). Quantitative (real-time) PCR analysis revealed a significantly higher mean expression of Hsp70 mRNA and ssc-ca-1 in GCs of growing follicles isolated from ovaries collected in the summer than in those from ovaries collected in the autumn (). However, immunoblot analysis confirmed the in vitro results that Hsp70 protein expression was not induced by heat stress ( and ). Sows had a lower conception rate during the summer, but this returned to normal levels once the environmental temperature declined in the autumn (Figure S9). This suggested that despite an increase in Hsp70 mRNA in GCs in the summer, ssc-ca-1 upregulation inhibits Hsp70 protein expression in the summer. As expected, heat stress in the summer promoted BimEL phosphorylation through the JNK pathway () and decreased protein expression of aromatase, a key enzyme in the conversion of androgens to estrogens ( and ). Together, these findings indicated that summer heat stress leads to ssc-ca-1–mediated inhibition of Hsp70 mRNA translation, thereby impairing the function of GCs and the development of growing follicles, which results in reproductive loss in female mammals that live in the summer.
Figure 5. In vivo effect of seasonal temperature changes on ssc-ca-1 and HSP70 expression in granulosa cells of growing follicles
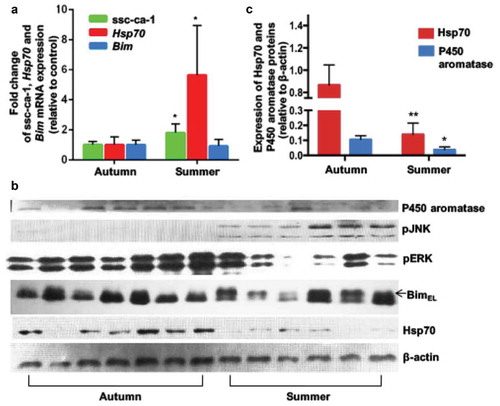
Spermatogonial stem cells are more heat stress tolerant than sertoli cells
For normal spermatogenesis, the porcine testicular temperature is maintained at 2–4°C lower than core body temperature [Citation22], thus control temperature of testes culture was selected at 35°C, and heating temperature was at 39°C. To investigate the effect of higher temperatures on testicular cells and function, we incubated piglet testes at 35°C (control) or 39°C (heat stress) for 2 h. Although the mean ssc-ca-1 level was significantly higher in SCs from heat-treated testes than in those from control testes (), we did not observe any differences in Hsp70 protein levels between the two groups (). This suggested ssc-ca-1 inhibits the translation of Hsp70 mRNA in SCs after heat stress in vitro. Interestingly, the mean fold change of upregulated Hsp70 protein level after in vivo heat exposure (45°C for 2 h/day, for 4 days) was significantly higher in SSCs () than in SCs ( and ). At the same time, mean ssc-ca-1 expression was increased in the SCs from testes subjected to both treatments, but not in the SSCs (). Based on these findings, we concluded that ssc-ca-1 is also present in testicular cells and has the same function as in ovarian cells. It can inhibit the expression of Hsp70 and mediate the different Hsp70 protein translation between SCs and SSCs during in vivo heat stress.
Figure 6. Ssc-ca-1 mediates difference in Hsp70 protein expression between Sertoli cells and spermatogonial stem cells after heat stress in vivo.
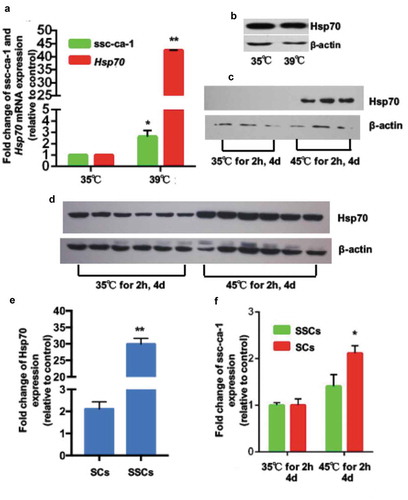
Discussion
In this study, we demonstrated a difference in the posttranscriptional regulation of Hsp70 by microRNAs in primordial follicles and growing follicles in response to heat stress. We identified two new microRNAs that interact with the 5′- or 3′-UTR of HspA1A mRNA (ssc-ca-1 and ssc-ca-3), to decrease its translation in GCs, but not in serum-starved GCs. These data suggested that microRNAs play a crucial role in growing follicles being more susceptible to heat stress–induced apoptosis than primordial follicles. Meanwhile, ssc-ca-1 modulated the different Hsp70 expression between SCs and SSCs after scrotal heat stress. Moreover, our findings support the idea there is one biological mechanism present in female and male mammals by which fertility is lower during hot seasons, but returns to normal levels once the environmental temperature declines in cooler seasons [Citation3,Citation4].
Many studies have shown that heat stress induces apoptosis in vitro. We found that exposure of cultured porcine GCs to 47°C for 2 h induced apoptotic cell death. This lethal heat stress condition led to phosphorylation of proapoptotic BimEL and an increase in caspase-3 activity, as well as a decrease in antiapoptotic Bcl-2 expression. Changes in the expression of apoptosis-related proteins did not occur at a sublethal temperature (42°C). Proteins of the BCL-2 family have either a pro- or antiapoptotic activity and regulate the mitochondrial pathway of apoptosis by controlling the permeabilization of the outer mitochondrial membrane. Unlike Bax and Bak, which are proapoptotic proteins that mediate mitochondrial damage, Bim regulates the ‘Bax/Bcl-2 balance’ to initiate apoptosis at an apical step, by activating Bax [Citation23] and inhibiting Bcl-2 [Citation24]. In a previous study, we showed BimEL plays an important role in follicular atresia and promotes apoptosis of porcine GCs in vitro [Citation25]. This suggests that the intrinsic mitochondrial apoptotic pathway was activated in GCs during recovery at 37°C after lethal heating.
HSP70s are intracellular stress proteins that provide cytoprotection against a variety of cellular stressors [Citation26]. In our current study, Hsp70 protein levels were significantly increased in GCs after heat stress, with the highest levels observed after exposure to sublethal heat stress. Furthermore, Hsp70 overexpression led to decreased caspase-3 activity after exposure to lethal heat stress, essentially blocking the apoptotic response. These findings are consistent with previous reports that concluded Hsp70 acts as a strong suppressor of apoptosis upstream of caspase-3 activation in U937 cells [Citation27] and in human acute lymphoblastic T cells [Citation28].
Several microRNAs posttranscriptionally regulate the HspA1A or HspA1B gene to decrease stress-induced Hsp70 protein expression [Citation15]. However, it was not clear whether microRNAs negatively regulate Hsp70 expression during heat stress. Dicer is pivotal for the last step in microRNA processing, which generates a mature microRNA duplex from a precursor stem-loop form [Citation13]. While heat stress treatment of GCs induced Dicer expression, DICER knockdown led to an increase in heat stress–induced Hsp70 expression. In serum-starved GCs and primordial follicles, Dicer expression was diminished. Taken together, Dicer decreases Hsp70 levels in response to heat stress through microRNAs, which contributes to cell apoptosis.
To identify potential microRNAs that suppress heat stress–induced Hsp70 expression in porcine GCs, we performed small RNA deep sequencing. A new microRNA molecule, ssc-ca-1, is encoded on the X chromosome. Overexpression of ssc-ca-1 inhibited Hsp70 protein expression, even after sublethal heat stress when Hsp70 mRNA levels were highly upregulated. Conversely, blocking this microRNA increased Hsp70 expression, exposure to lethal heat stress. We established that the inhibition by ssc-ca-1 occurs through binding to the UTRs of HspA1A. Importantly, the negative effect of microRNAs on Hsp70 expression after heat stress was not seen when cells were serum starved. Indeed, our findings in proliferating and serum-starved GCs were replicated in heat stress–treated growing and primordial follicles that had been isolated from whole ovaries.
Our study suggests that primordial follicles, which form the reservoir of female gametes during the reproductive lifespan of females, have unique mechanisms of less microRNAs to avoid damage from environmental challenges, while growing follicles lack this protection and are susceptible to heat stress. This is logical, as growing follicles do not have time to recover from significant damage and can, therefore, propagate adverse genetic information into the next generation. Primordial follicles are protected from stress-based damage, and can be recruited at later times when environmental circumstances are more favourable.
We also showed that ssc-ca-1 mediated the difference in Hsp70 protein expression between SCs and SSCs after male piglets were subject to heat stress in vivo. Upregulated ssc-ca-1 expression inhibited Hsp70 protein expression in SCs after heat stress. However, in SSCs, ssc-ca-1 expression was unaffected by the heat treatment, resulting in an increased Hsp70 expression.
In conclusion, we have elucidated the expression pattern and function of ssc-ca-1 in germ cells and their surrounding cells under heat stress, and have shown that microRNA-mediated regulation of Hsp70 in response to heat stress is different among quiescent and proliferative germ cells in pigs.
Author Contributions
Y. Wu, T.K. Woodruff and S. Zeng provided conceptualization and design; Y. Wu, Y. Wang, Q. Liu, H. Gao, M. Cui, J. Liu, L. Chen, J. Wang, W. Zeng performed the experiments; Y. Wu, L.J. Zhu, P. Zhao and J. Liu analysed the data; Y. Wu, T.K. Woodruff and S. Zeng wrote the manuscript; and all authors provided the final approval of the manuscript.
Correction Statement
This article has been republished with minor changes. These changes do not impact the academic content of the article.
Supplemental Material
Download Zip (571.9 KB)Acknowledgments
This research was in part supported by a grant from the National Key R&D Program of China [2017YFC1002003], China Postdoctoral Science Foundation (2013M530780), National Natural Science Foundation of China (31470077 and 31672419), and the National Institutes of Health (NIH) (P01 HD021921). Part of the work was conducted during a yearlong sabbatical (SZ) in the Woodruff Laboratory and supported by the Center for Reproductive Health After Disease (P50HD076188) from the NIH’s National Center for Translational Research in Reproduction and Infertility. Dr. Stacey Tobin provided editorial assistance (The Tobin Touch LLC).
Disclosure statement
No potential conflict of interest was reported by the authors.
Supplementary Materials
Supplemental Materials data for this article can be accessed here.
Additional information
Funding
References
- De Rensis F, Lopez-Gatius F, Garcia-Ispierto I, et al. Causes of declining fertility in dairy cows during the warm season. Theriogenology. 2017;91:145–153.
- Pena ST, Gummow B, Parker AJ, et al. Revisiting summer infertility in the pig: could heat stress-induced sperm DNA damage negatively affect early embryo development? Anim Prod Sci. 2017;57:1975–1983.
- Hansen PJ. Effects of heat stress on mammalian reproduction. Philos Trans R Soc London, Ser B. 2009;364:3341–3350.
- Roth Z, Arav A, Bor A, et al. Improvement of quality of oocytes collected in the autumn by enhanced removal of impaired follicles from previously heat-stressed cows. Reproduction. 2001;122:737–744.
- Ma W, An L, Wu Z, et al. Efficient and safe recipient preparation for transplantation of mouse spermatogonial stem cells: pretreating testes with heat shock. Biol Reprod. 2011;85:670–677.
- Tilly JL, Kowalski KI, Johnson AL, et al. Involvement of apoptosis in ovarian follicular atresia and postovulatory regression. Endocrinology. 1991;129:2799–2801.
- Shimizu T, Ohshima I, Ozawa M, et al. Heat stress diminishes gonadotropin receptor expression and enhances susceptibility to apoptosis of rat granulosa cells. Reproduction. 2005;129:463–472.
- Lue Y, Wang C, Liu YX, et al. Transient testicular warming enhances the suppressive effect of testosterone on spermatogenesis in adult cynomolgus monkeys (Macaca fascicularis). J Clin Endocrinol Metab. 2006;91:539–545.
- Radons J. The human HSP70 family of chaperones: where do we stand? Cell Stress Chaperones. 2016;21:379–404.
- Zhao M, Tang D, Lechpammer S, et al. Double-stranded RNA-dependent protein kinase (pkr) is essential for thermotolerance, accumulation of HSP70, and stabilization of ARE-containing HSP70 mRNA during stress. J Biol Chem. 2002;277:44539–44547.
- Wang T, Yu Q, Chen J, et al. PP2A mediated AMPK inhibition promotes HSP70 expression in heat shock response. PloS One. 2010;5:e13096.
- Yamashita M, Kamitani W, Yanai H, et al. Persistent borna disease virus infection confers instability of HSP70 mRNA in glial cells during heat stress. J Virol. 2005;79:2033–2041.
- Kim VN, Han J, Siomi MC. Biogenesis of small RNAs in animals. Nat Rev Mol Cell Biol. 2009;10:126–139.
- Tang Q, Yuan Q, Li H, et al. miR-223/Hsp70/JNK/JUN/miR-223 feedback loop modulates the chemoresistance of osteosarcoma to cisplatin. Biochem Biophys Res Commun. 2018;497:827–834.
- Tranter M, Helsley RN, Paulding WR, et al. Coordinated post-transcriptional regulation of Hsp70.3 gene expression by microRNA and alternative polyadenylation. J Biol Chem. 2011;286:29828–29837.
- Mendell JT, Olson EN. MicroRNAs in stress signaling and human disease. Cell. 2012;148:1172–1187.
- Asada S, Takahashi T, Isodono K, et al. Downregulation of Dicer expression by serum withdrawal sensitizes human endothelial cells to apoptosis. Am J Physiol Heart Circ Physiol. 2008;295:H2512–21.
- Hackenberg M, Rodriguez-Ezpeleta N, Aransay AM. miRanalyzer: an update on the detection and analysis of microRNAs in high-throughput sequencing experiments. Nucleic Acids Res. 2011;39:W132–8.
- Hunter RH, Bogh IB, Einer-Jensen N, et al. Pre-ovulatory graafian follicles are cooler than neighbouring stroma in pig ovaries. Hum Reprod. 2000;15:273–283.
- Lei L, Jin S, Gonzalez G, et al. The regulatory role of Dicer in folliculogenesis in mice. Mol Cell Endocrinol. 2010;315:63–73.
- Huynh TT, Aarnink AJ, Verstegen MW, et al. Effects of increasing temperatures on physiological changes in pigs at different relative humidities. J Anim Sci. 2005;83:1385–1396.
- Ivell R. Lifestyle impact and the biology of the human scrotum. Reprod Biol Endocrinol. 2007;5:15.
- Czabotar PE, Colman PM, Huang DC. Bax activation by Bim? Cell Death Differ. 2009;16:1187–1191.
- Zong WX. BH3-only proteins that bind pro-survival Bcl-2 family members fail to induce apoptosis in the absence of Bax and Bak. Genes Dev. 2001;15:1481–1486.
- Wang XL, Wu Y, Tan LB, et al. Follicle-stimulating hormone regulates pro-apoptotic protein Bcl-2-interacting mediator of cell death-extra long (BimEL)-induced porcine granulosa cell apoptosis. J Biol Chem. 2012;287:10166–10177.
- Lanneau D, Brunet M, Frisan E, et al. Heat shock proteins: essential proteins for apoptosis regulation. J Cell Mol Med. 2008;12:743–761.
- Li CY, Lee JS, Ko YG, et al. Heat shock protein 70 inhibits apoptosis downstream of cytochrome c release and upstream of caspase-3 activation. J Biol Chem. 2000;275:25665–25671.
- Stankiewicz AR, Lachapelle G, Foo CP, et al. Hsp70 inhibits heat-induced apoptosis upstream of mitochondria by preventing Bax translocation. J Biol Chem. 2005;280:38729–38739.