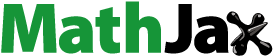
ABSTRACT
Retinoic acid-inducible gene I (RIG-I) is responsible for innate immunity via the recognition of short double-stranded RNAs in the cytosol. With the clue that G-U wobble base pairs in the influenza A virus’s RNA promoter region are responsible for RIG-I activation, we determined the complex structure of RIG-I ΔCARD and a short hairpin RNA with G-U wobble base pairs by X-ray crystallography. Interestingly, the overall helical backbone trace was not affected by the presence of the wobble base pairs; however, the base pair inclination and helical axis angle changed upon RIG-I binding. NMR spectroscopy revealed that RIG-I binding renders the flexible base pair of the influenza A virus’s RNA promoter region between the two G-U wobble base pairs even more flexible. Binding to RNA with wobble base pairs resulted in a more flexible RIG-I complex. This flexible complex formation correlates with the entropy-favoured binding of RIG-I and RNA, which results in tighter binding affinity and RIG-I activation. This study suggests that the structure and dynamics of RIG-I are tailored to the binding of specific RNA sequences with different flexibility.
Introduction
Retinoic acid-inducible gene I (RIG-I) is a cytosolic pathogen recognition receptor, which is known to be responsible for antiviral innate immunity. It is activated by short, double-stranded RNA (dsRNA) with blunt ends and a 5ʹ triphosphate moiety [Citation1,Citation2]. The 5ʹ triphosphate moiety is regarded as a critical structural feature of non-self, pathogen-associated molecular patterns, which are not common in the cytosol. RIG-I can detect several viral RNAs, such as influenza A and Sendai virus, that possess this structural feature [Citation3,Citation4].
Several studies have provided structural insights into the RNA recognition ability of RIG-I [Citation5–Citation7]. RIG-I has three domains: an N-terminal tandem caspase recruitment domain (CARD), an RNA helicase domain, and a C-terminal repressor domain (RD), also known as the RNA-binding domain (Fig. S1). In an inactivated state, the CARD is self-inhibited by interaction with the helicase domain, while the RD is structurally independent and flexible. The first step of RNA recognition by RIG-I is the complex formation between the RD of RIG-I and the blunt-ended dsRNA. In the presence of an RNA ligand, the helicase domain interacts with the helical backbone of the bound RNA, which leads to a structural change in the ATPase active site of the helicase domain so that RIG-I can hydrolyse ATP [Citation6,Citation8]. Upon ATP hydrolysis, the interaction between the CARD and the helicase domain ceases, and the CARD is freely released. Released CARDs experience K63-linked polyubiquitination, which causes CARD oligomerization [Citation9]. Oligomerized CARDs can interact with mitochondrial antiviral-signalling proteins, and further immunogenic signalling can be activated through IRF-3, NF-κB, and Type I interferon [Citation10].
Under these circumstances, the RNA of the influenza A virus is postulated to activate RIG-I [Citation3]. The influenza A virus has highly conserved non-coding regions in its RNA genome. In these regions, the 5ʹ- and 3ʹ- ends are nearly self-complementary (); therefore, they are able to form a blunt-ended duplex [Citation11]. This so-called promoter region binds to the viral RNA polymerase, and its non-canonical wobble base pairs are crucial for viral RNA replication [Citation12]. The promoter region of the viral RNA forms a hook-like structure in the presence of the viral polymerase [Citation13]; however, it forms the blunt-ended duplex in its absence [Citation14].
Figure 1. (A) Terminal region of influenza A virus RNA promoter sequence (left) and hp-IAV RNA sequence (right). Red box indicates sequences that mimic the influenza A RNA promoter sequence, which contains two G-U wobble base pairs. (B) Crystal structure of hp-IAV bound to RIG-I ΔCARD. (C) U11 of hp-IAV RNA is proposed to make polar contact with a loop (490–500) of RIG-I ΔCARD (2Fo-Fc map, contour 1.0σ). (D) View of U11 from the top. Atoms N and O in U11 base are located close to the backbone C = O of Q498 and the backbone N-H of Q500 (distance 2.6 and 3.0 Å, respectively).
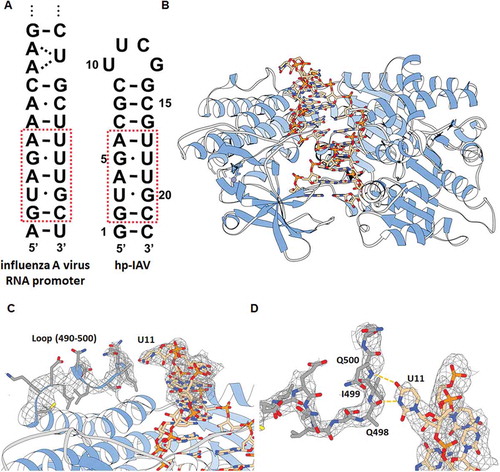
In a previous study, we showed that a short hairpin RNA that mimics the influenza A promoter region shows interferon induction via RIG-I activation, even in the absence of 5ʹ triphosphate, which might be due to the wobble base pairs in the promoter region [Citation15]. However, there is no understanding of the structural basis for this result. Several molecular structures of RIG-I complexed with dsRNA or hairpin RNA have been determined by NMR or X-ray crystallography [Citation5–Citation7]; however, these studies focused only on the 5ʹ end of the RNA and not its sequence. All the RNAs used in these structural studies were composed of Watson-Crick matched bases, even though wobble base pairs, which are commonly observed in biological systems, can affect the structure and dynamics of RNA. With this as background, we determined the molecular structure and biophysical characteristics of RIG-I when bound to wobble base pair-bearing RNA in order to deduce what differences the wobble base pair may cause.
Results
Crystal structure of RIG-I ΔCARD in complex with hairpin RNA containing G-U wobble base pairs (hp-IAV)
In order to investigate the effect of wobble base pairs on the helical structure of the influenza promoter region and its recognition by RIG-I, we designed a hairpin RNA of 22 nucleotides (hp-IAV) that mimics the influenza A virus RNA promoter region (), because RIG-I covers at least 8 base pairs of dsRNA [Citation7]. hp-IAV is a hairpin RNA with 9 base pairs connected by a UUCG tetraloop, where two G-U wobble base pairs are located at the third and fifth base pairs from the blunt end, as in the influenza A promoter. In order to observe how hp-IAV binds to RIG-I and whether its helical shape changes, we tried to determine the structure of RIG-I in complex with hp-IAV.
Knowing that the binding of dsRNA with RIG-I occurs via the RD and the helicase domain without the CARD [Citation5–Citation7], we crystallized the complex of hp-IAV and RIG-I ΔCARD (residues 240–925) to investigate the structural features of the RNA helix backbone upon RIG-I binding (). We determined the structure of RIG-I ΔCARD in complex with hp-IAV in the resolution of 3.0Å (Table S1). hp-IAV shows a hairpin structure with a UUCG tetraloop when bound to RIG-I, as expected. By comparing the previously determined structure of RIG-I ΔCARD with dsRNA (where all base pairs were matching, PDB ID: 5F9F) [Citation16], we see the backbone trace of the protein remains almost the same (RMSD = 0.468Å), which means that the RNA binding mode of RIG-I ΔCARD is almost the same (Fig. S2A). Interestingly, the first to eighth base pairs of the overall phosphate backbone are well conserved upon RIG-I ΔCARD binding, regardless of the RNA sequence (Fig. S2B). Accordingly, RIG-I ΔCARD and hp-IAV structure showed almost same binding surface with the RIG-I ΔCARD and A-U rich hairpin dsRNA structure previously reported (Fig. S3) [Citation16]. In addition, we observed additional electron density for the flexible loop of RIG-I (490–500), which is located in the vicinity of the protruding base U11 in the UUCG tetraloop (). Electron density of this flexible loop region cannot be observed in the structure used in molecular replacement (MR) probe (PDB ID: 5F9F), however, this region was suggested to be a binding surface to dsRNA [Citation5]. This flexible loop region, which includes Q498, I499, and Q500, might be an additional binding region that interacts with other hairpin RNAs with 9 base pairs and UUCG tetraloops ().
By inspecting the RNA base pairs in the crystal structure of the RIG-I ΔCARD bound hp-IAV, it can be observed that all G-U wobble base pairs were conserved as cis- base pairs with a typical Watson-Crick edge, and formed two hydrogen bonds between bases, as generally observed for G-U wobble base pairs. The C1ʹ-C1ʹ distances of the G-U wobble base pairs are about 10.6 Å, as with other G-U wobble base pairs, and the angles between the C1ʹ-C1ʹ vector and the glycosidic bond (λ) for G and U are close to the typical values (40° and 65° for G and U, respectively [Citation17]) ().
Table 1. Base pair parameters of C1ʹ-C1ʹ distance, angle between the glycosidic bond and C1ʹ-C1ʹ line (λ1, λ2). Helical twist angles (Ωh) with preceding base pairs are also presented. Free indicates the parameters calculated from the crystal structure of hp-IAV only. Bound indicates the parameters calculated from the crystal structure of hp-IAV/RIG-I ΔCARD. All parameters are calculated by w3DNA [Citation31].
Crystal structure of hp-IAV
We crystallized hp-IAV to investigate the structural features of RNA helix and base pairs without RIG-I. The UUCG tetraloop is known to form a highly stable loop in solution [Citation18]; however, under our crystallization conditions, the structure of hp-IAV was determined to be a dimer (). Similar behaviour of other oligonucleotides has been observed under a high concentration of precipitants [Citation19]. In the complex formation state prior to crystallization, wrapping the dsRNA helix with RIG-I might prevent this hairpin-to-duplex transition during crystallization.
Figure 2. (A) 2Fo-Fc electron density map of the crystal structure of hp-IAV RNA (contour 1.0σ). (B) Two different helical conformations of the terminal region of hp-IAV in a single asymmetric unit were aligned. RNAs show a typical A-form helix structure. (C) Hydrogen bonds in base pairs U3-G20, A4-U19, and G5-U18. (D) Helical representation of the first 9 base pairs in free hp-IAV (beige) and RIG-I bound hp-IAV (pink). (E) Base pair stacking and global helical axis in two different state of dsRNA : free hp-IAV (left), RIG-I bound hp-IAV (right).
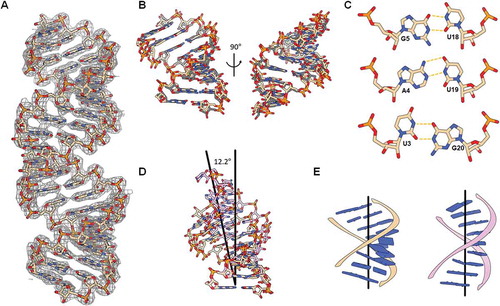
In the crystal state, two hp-IAV molecules formed one 22-base pair duplex, which is stacked to form a pseudo-infinite helix; there were two hp-IAV strands in a single asymmetric unit, of which symmetry mate formed two blunt-ended dsRNA. These two dsRNA duplexes showed a slight difference in their backbone structure and base pair stacking (). An overlay of the four terminal helix regions indicated that the region with G-U wobble base pairs forms an A-form helix.
The two wobble base pairs (U3-G20, G5-U18) in hp-IAV were made with two hydrogen bonds on the Watson-Crick side of the bases. The canonical A4-U19 pair between the two wobble base pairs remained a typical Watson-Crick base pair, without significant disturbance of its base pair structure (). The λ angles of U3-G20 (71.2° and 42.8°, respectively) deviated from those of typical U-G wobble base pairs (65° and 40°, respectively) () [Citation17]. Also, U3-G20 and G5-U18 base pairs showed 43.8° and 23.4° of helical twist angle with preceding base pairs respectively, which agrees with the typical values observed in U-G and G-U wobble base pairs [Citation20]. The presence of these two G-U wobble base pairs caused the phosphate backbone to deviate slightly from a normal A-form helix, causing the local helical axis to bend ~7° over 9 base pairs.
Comparison of free hp-IAV with hp-IAV bound to RIG-I ΔCARD
In the RIG-I bound state, the two G-U wobble base pairs were different from the free hp-IAV state: especially the λ angles of the U3 and G20 bases in wobble base pairs were more typical as compared to the free form. Also, the A4-U19 pair, located between the two wobble pairs, showed the typical Watson-Crick base pair properties. In addition to the conformational changes that occurred in the G-U wobble base pairs when RIG-I ΔCARD binds, there was a significant change in the dsRNA helix shape. Aligning the first three base pairs of the structures of the free and RIG-I-bound forms of hp-IAV showed an approximately 12° difference between the orientation of the global helical axis (). The overall helix was observed to straighten upon RIG-I binding (). With respect to the global helical axis, all eight base pairs with backbones interacting with RIG-I ΔCARD showed small inclination angles (average 6°, (Fig. S4)), meaning that each base pair plane was nearly perpendicular to the global helical axis of the RNA duplex. This low base inclination angle indicates that all base pairs slightly deviated from the typical A-form RNA helix, somewhat similar to the base pair stacking in a B-form helix. In contrast, the base pair inclination angles of free hp-IAV showed a high inclination angle (average 14°), similar to a typical A-form helix, which has inclination angles between 10° and 20°.
Comparison of the different dsRNA helix structures in complex with RIG-I ΔCARD (Fig. S4) [Citation5,Citation7,Citation16] revealed that all dsRNAs have low inclination angles in the bound state, which deviates from the typical A-form helix, regardless of the sequences or 5ʹ-end modifications. Because the free state of hp-IAV has a typical A-form helix, it can be deduced that the dsRNA helix experiences structural changes from the proper helix form that can be observed in other RIG-I bound structures.
Base pair stability indicated by hydrogen exchange rates of imino protons
Keeping in mind that the conformational change in the backbone of hp-IAV occurs upon RIG-I binding, we attempted to figure out which step of RIG-I binding causes the conformational change. In the auto-inhibited form, dsRNA can bind to RIG-I RD first because the helicase domain interacts with the CARD [Citation6]. In order to understand the effect of the two G-U wobble base pairs on RIG-I RD binding, we quantified the base pair stability by measuring the hydrogen exchange rate of the imino protons of each base pair in the presence or absence of RIG-I RD using NMR spectroscopy.
We synthesized an RNA identical to hp-IAV, except that the G-U wobble base pairs were replaced by G-C Watson-Crick base pairs (hp-WC) for comparison (). The 1H NMR spectrum of hp-WC shows no imino protons of U3-G20 and G5-U18 belong to the non-canonical base pair region (9–12 ppm), and all the peaks of imino protons of guanine and uridine that form base pairs were identified ().
Figure 3. (A) RNAs used in NMR experiments with RIG-I RD. Asterisks indicate where hp-IAV has its G-U wobble base pairs changed to Watson-Crick G-C base pairs (hp-WC). 1D 1H NMR spectrum in the imino proton region (9–15 ppm) of hp-IAV (top) and hp-WC (bottom). (B) Imino proton exchange rates of imino protons in hp-IAV (left) and hp-WC (right). Exchange rates in the free state (white) and the RIG-I RD-bound state (black) were calculated.
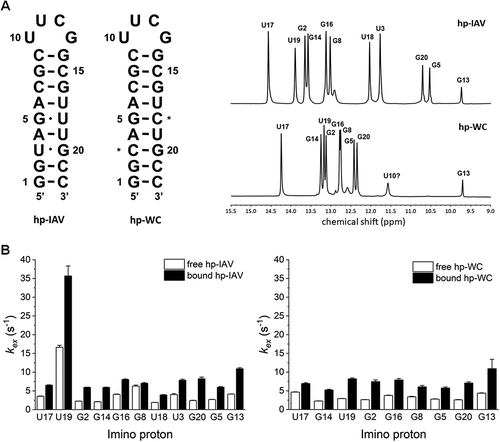
We then observed the perturbation of imino proton peaks of the dsRNA and backbone H-N peaks in the RIG-I RD during titration. The binding of both hp-IAV and hp-WC was saturated at 1:1 stoichiometry during RIG-I RD titration, and the 1H-15N heteronuclear single quantum coherence (HSQC) spectra showed different changes with hp-IAV than with hp-WC, indicating that the two dsRNAs bind to RIG-I RD in a different manner (Fig. S5).
Measurements of the imino proton exchange rate indicated that the U3-G20 and G5-U18 base pairs in hp-IAV are stable, similar to typical Watson-Crick base pairs (kex ~ 6 s−1), while the A4-U19 base pair in hp-IAV seems less stable than other Watson-Crick base pairs (kex ~ 16 s−1). In hp-WC, the A4-U19 pair seems to be stable, with a typical imino proton exchange rate (kex ~ 5 s−1) (Fig. S6).
In the RIG-I RD bound state, all imino protons in both hp-IAV and hp-WC showed a slight increase in exchange rate (). Interestingly, the unstable A4-U19 base pair of hp-IAV became much more exchange-prone than other base pairs upon binding to RIG-I RD. The imino proton exchange rate of U19 increased to 32 s−1 from 16 s−1, in contrast to the exchange rate of U19 in hp-WC, which did not change significantly. Also, the imino protons in the G-U wobble base pairs of hp-IAV showed no significant increase in exchange rate, which means these base pairs remain stable in the RIG-I RD bound state.
These findings suggest that some structural changes associated with the destabilization of the A4-U19 base pair occur when RIG-I RD binds to hp-IAV. This might be due to a steric clash between the RNA helix and RIG-I RD, which was shown when we aligned our RNA helix crystal structure of free hp-IAV upon the 5ʹ-OH dsRNA/RIG-I RD complex crystal structure of another group (PDB ID: 3OG8) (Fig. S7) [Citation21].
The large exchange rate of A4-U19 in hp-IAV might be related to the flexibility of the helix, which can be observed in the crystal structure of free hp-IAV. It can be deduced that the U3-G20 and G5-U18 base pairs in hp-IAV lead A4-U19 to be unstable, whereas the two G-U wobble base pairs themselves have an unexpectedly stable exchange rate. In the crystal structure of the hp-IAV/RIG-I ΔCARD complex, the A4-U19 base pair appears more like a canonical base pair than in its free state. The λ angles of the A4-U19 base pair of hp-IAV bound to RIG-I ΔCARD are 55° and 58.1°, which are closer to the values of canonical base pairs (Table 1).
hp-IAV forms more unstable complex with RIG-I than Watson-Crick paired RNA
To further characterize the changes in RIG-I induced by the binding of each type of RNA, we measured the melting temperature of several RIG-I constructs via differential scanning fluorimetry (DSF). We also designed RNAs whose whole phosphate backbones are substituted to phosphorothioate bonds (hp-IAV-PS and hp-WC-PS) in order to check whether the effect of G-U wobble base pairs in RNA sequence can also affect the binding pattern of RNA with the different backbone in the RIG-I/RNA complex formation. We measured the melting temperature for three different constructs – RIG-I RD (800–925), RIG-I ΔCARD (240–925), and full length RIG-I (1–925) – representing each stage of recognition of the dsRNA.
RIG-I RD showed a monophasic melting curve, with a melting temperature (Tm) of 46.6 ℃ (). For all 4 different RNAs, the melting curve of RIG-I RD shifted to the right, indicating an increase in stability. For hp-IAV binding to RIG-I RD, the Tm shifted to 53.9 ℃, which was lower than the Tm of the RIG-I RD/hp-WC complex (55.6 ℃). Interestingly, this feature was also observed for the complexes of the backbone modified RNAs, which had Tm values of 56.8 ℃ (hp-IAV-PS) and 58.8 ℃ (hp-WC-PS). This tendency is regardless of backbone modification, which means the sequence dependency, especially G-U wobble base pairs, affects the stability of the protein/RNA complex. From these measurements, we conclude that RNAs with G-U wobble base pairs form complexes with more thermally unstable than RNAs with Watson-Crick base pairs.
Figure 4. Melting curves for three different constructs of RIG-I RD (A), RIG-I ΔCARD (B), and full length RIG-I (C). Each construct was subjected to DSF alone and in complex with 4 different RNAs (hp-IAV, hp-WC, hp-IAV-PS, and hp-WC-PS) at 1.2 times the amount of protein.
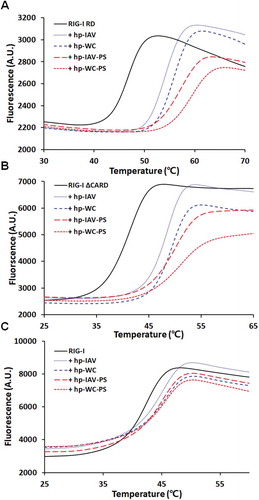
We also measured the Tm for the RIG-I ΔCARD construct in complex with the 4 different RNAs used above (). For RIG-I ΔCARD itself, the melting curve was monophasic, with a Tm of 40.6 ℃. Like the RIG-I RD, RIG-I ΔCARD showed Tm shift to higher values upon binding to the RNAs: 47.8 ℃ for hp-IAV and 48.8 ℃ for hp-WC. Phosphorothioate backbone modifications in the RNAs also showed the same trends as with RIG-I RD (Tm increased to 49.6 ℃ with hp-IAV-PS and to 50.8 ℃ with hp-WC-PS). Thus, for RIG-I ΔCARD, the presence of the G-U wobble base pairs led to slightly lower Tm values, regardless of its backbone modification, which means that G-U wobble base pairs itself forms a slightly less stable complex with RIG-I ΔCARD.
For full length RIG-I, the Tm of the protein itself was 41.8 ℃, and although the Tm shifted to higher values upon RNA binding, the shift was of smaller magnitude (). The same trends in Tm values were also observed: RNAs with wobble base pairs formed complexes with lower Tm values for phosphate backbone RNA. The Tm of RIG-I in complex with hp-IAV was 43.8 ℃ and that of hp-WC was 44.3 ℃. For the phosphorothioate-modified RNAs, the Tm values were the same: 47.2 ℃ for the complexes of RIG-I with either hp-IAV-PS or hp-WC-PS.
In each case, the upward shift in Tm values indicates an increase in stability upon binding to the RNAs. The smaller magnitude of the Tm shifts for the full length protein suggests that the complex between the complete RIG-I sequence and dsRNA of wobble base pairs is less stable than the complexes formed by the smaller constructs. Another common feature of the melting curves of the three different RIG-I constructs is that backbone-modified RNAs made the Tm shift higher than RNAs with a regular phosphate backbone. This could be because the backbone modification additionally affected the RIG-I binding affinity or the rigidity of the RNA itself.
Entropy-favoured binding and RIG-I dependent IFN-β induction
According to the results above, RIG-I RD binding weakens the A-U base pair sandwiched between the G-U wobble base pairs, and the overall helix experiences changes in base pair stacking and axis angle. DSF analysis showed that RIG-I forms less stable complexes with RNAs with G-U wobble base pairs than with RNAs composed solely of Watson-Crick base pairs. In order to determine how the RNA sequence affected the binding affinity and thermodynamic properties of the complex, we used isothermal titration calorimetry (ITC).
All 4 RNAs showed 1:1 binding stoichiometry when binding to RIG-I RD, with affinities in the nanomolar range (). As reported previously, the majority of RIG-I RD’s interactions with RNA are mediated by the RNA’s phosphate backbone. Thus, the phosphorothioate-modified RNAs (hp-IAV-PS, hp-WC-PS) showed slightly tighter binding affinity (hp-IAV-PS KD 31.6 nM, hp-WC-PS KD 97.1 nM) than the corresponding RNAs with a normal phosphate backbone RNAs (hp-IAV KD 46.7 nM, hp-WC KD 114 nM). This result agrees with the DSF results, where the Tm of phosphorothioate-modified RNAs was higher than that of RNAs with a phosphate backbone.
Figure 5. Binding of RIG-I RD to short hairpin RNAs. ITC results of RIG-I RD titration with hp-IAV (A), hp-WC (B), hp-IAV-PS (C), hp-WC-PS (D). Representative thermodynamic parameters (N, KD, ΔH, ΔS) are indicated as mean ± standard deviation.
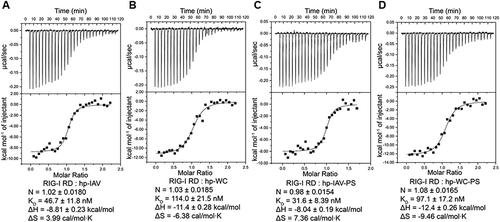
Interestingly, the RNAs with wobble base pairs showed tighter binding, by 2- to 3-fold, to RIG-I RD regardless of the backbone modification, suggesting a larger release of Gibbs free energy upon the binding. Accordingly, complex formation with wobble base paired RNAs resulted in a positive entropy change (ΔS values of 3.99 cal/mol∙K for hp-IAV, 7.36 cal/mol∙K hp-IAV-PS) (, ). In contrast, ΔS values were negative for complex formation with hp-WC (−6.38 cal/mol∙K) and hp-WC-PS (−9.46 cal/mol∙K) (, ). With the observation that the enthalpy changes for RNAs with G-U wobble base pairs were smaller than those for Watson-Crick base paired RNAs, it can be deduced that the change in entropy drove the Gibbs free energy change for the binding reaction, leading to tighter binding affinity. Thus, regardless of backbone modification, the RNA sequence drives the entropy-favoured binding to RIG-I RD.
With these observations together, G-U wobble base pairs lead entropy-favoured binding to RIG-I RD and formation of a thermally unstable complex with RIG-I constructs. These trends can also be observed for the phosphorothioate backbone modified RNA, except for the Tm shift of full length RIG-I: Tm shift was same between hp-IAV-PS and hp-WC-PS. We checked whether the G-U wobble base pair introduction into phosphorothioate backbone RNA also leads RIG-I activation. By comparison to RIG-I knockout (KO) HEK293T cell, hp-IAV-PS induced interferon β (IFN-β) in RIG-I dependent manner while there is no significant difference in IFN-β induction level for hp-WC-PS ().
Figure 6. Relative IFN-β promoter activity in HEK293T cells (black) and RIG-I KO HEK293T cells (grey). Error bars indicate the standard deviation. FLuc expression from the IFN-β promoter was normalized to RLuc expression from the CMV promoter. Experiments were performed in triplicate, and p-values were determined by Student’s t-test. n.s., p > 0.05; **, p ≤ 0.01.
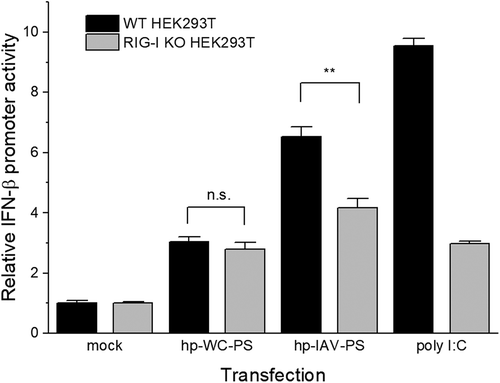
Discussion
In this study, we observed that RIG-I RD binding makes the A4-U19 base pair in hp-IAV more flexible with higher binding affinity, whereas the helicase domain binding sequentially leads the A4-U19 base pair to be stable and the G-U base pairs to deviate from their typical arrangement in the crystal structure [Citation17]. This can be explained by the structural transition of the overall helix shape to the proper shape, with a low base pair inclination angle. With two G-U wobble base pairs, hp-IAV has flexibility in its base pair and duplex structure to ease the structural transformation to the proper helix shape upon RIG-I binding ().
The crystal structure of free hp-IAV shows that the presence of two G-U wobble base pairs does not affect the overall A-form helix of dsRNA significantly; however, it can lead to a steric clash when it binds to RIG-I RD (Fig. S7). Moreover, the unstable A4-U19 base pair was observed by measuring the imino proton exchange rate. In the complex formed with RIG-I ΔCARD, the hp-IAV helix was straightened by the interaction, and its base pair stacking became much more perpendicular to its helical axis (). Comparing this structure to the structures of Watson-Crick base paired RNAs, we deduced that RIG-I ΔCARD binding induces or conformationally selects the specific helical shape of its target dsRNA.
In hp-IAV bound to RIG-I RD, all observable imino proton peaks showed a slightly increased hydrogen exchange rate (). This implies that all imino protons are more exposed to the solvent upon binding to RIG-I RD. The hydrogen exchange rate of imino protons participating in RNA base pairs correlates with the base pair stability. The A-U base pair sandwiched between two G-U wobble base pairs showed intrinsically unstable base pairing, and this base pair became even more unstable when hp-IAV bound to RIG-I RD. We did not observe any significant change in the hydrogen exchange rate of the base pair in hp-WC which is located in the same position as in hp-IAV. This difference in kex suggests that the two G-U wobble base pairs are responsible for the stability of the A-U base pair between them, which dramatically decreases upon RIG-I RD binding. Along with the observation that the overall helix shape and the orientation of the helical axis changes upon RIG-I ΔCARD binding, the hydrogen exchange rate measurements suggest that the structural changes RNA undergoes occur when it first interacts with RIG-I RD. This structural change might alleviate the expected steric clash (Fig. S7).
With the observation that RIG-I RD binding leads to base pair instability, it was also observed that RNA binding increased the melting temperature of RIG-I. Melting temperature generally increases when a ligand or other biomolecule binds because in the bound state the protein requires more energy to be unfolded. All constructs of RIG-I (RIG-I RD, RIG-I ΔCARD, full length RIG-I) showed Tm values shifting to higher temperature upon binding to all 4 sequences of RNA. Interestingly, RNAs with a modified backbone (phosphorothioate bond) induced a larger Tm shift than RNAs with a normal phosphate backbone. This result suggests that complexes with phosphorothioate-containing RNAs (hp-IAV-PS, hp-WC-PS) are more rigid. In addition, Tm increases induced by RNAs with G-U wobble base pairs were smaller than those induced by RNAs with Watson-Crick base pairs. This result agrees with the observation that RNA base pairs become unstable as RIG-I RD binds. Combining these observations, we conclude that hp-IAV or hp-IAV-PS binding to RIG-I leads to increased structural instability in protein structure overall, as hp-IAV undergoes structural destabilization of A-U base pair.
In the ITC experiments, RNAs with a phosphorothioate-modified backbone (hp-IAV-PS, hp-WC-PS) showed lower KD values with RIG-I RD than RNAs with a phosphate backbone (hp-IAV, hp-WC). This tendency agrees with the DSF finding that hp-IAV-PS and hp-WC-PS formed more rigid complexes with RIG-I. In contrast, RNAs with G-U wobble base pairs (hp-IAV, hp-IAV-PS) showed lower Tm shifts than Watson-Crick paired RNAs, even though their KD values indicated tighter binding to RIG-I RD. The low KD values might be responsible for the positive value of ΔS during binding, which is not usual for the binding of two molecules. This increase of the total entropy may due to solvent release during the complex formation, but it could also be due to the increase in the RNA’s conformational flexibility. This conclusion is supported by the imino proton exchange rates. Not only the structural complex formation, but RNA base pair destabilization during RIG-I binding might also affect the overall entropy change.
With the observation that RIG-I ΔCARD (with helicase domain) binding leads to a change in the helical shape, it can be deduced that the structural flexibility of G-U wobble base paired RNA leads entropy-favoured binding to RIG-I RD, which also means that the flexibility of the dsRNA structure might ease the binding to the helicase domain. Moreover, the flexibility affects the binding thermodynamics, and hp-IAV-PS can activate RIG-I with its strong binding affinity (, Fig. S8)
RIG-I’s helicase domain does not interact with the bases of the dsRNA of stem region, so the structural rigidity of the dsRNA helix might be a key to regulating the binding and RIG-I activation preferences. While there are several possible mechanisms for recognizing and binding dsRNA, it should be considered that dsRNA helix rigidity may affect RIG-I recognition. There are several papers showing that RIG-I activation exhibits preferences for certain sequences [Citation22,Citation23]. Our finding that hp-IAV-PS and hp-WC-PS induce different RIG-I signalling levels shows that RIG-I activation might be sequence dependent, which is actually structural stability dependence; that is, flexible RNA structure might enhance the activation of RIG-I.
In conclusion, we observed a change in the base pair stability and base pair inclination angle upon RIG-I recognition of RNA. These results shed light on the way RIG-I induces the proper helical angle. This approach can be applied to design and fine-tune RNA ligands with optimal helical shape and stability that can activate RIG-I in a more efficient manner.
Materials and methods
RNAs and proteins
All RNAs were chemically synthesized and purchased from IDT (Integrated DNA Technologies, Coralville, Iowa, United States). The RD of RIG-I (800–925) was cloned into a 2M-T vector, and the protein expression was performed in an E. coli BL21(DE3) strain. E. coli was grown at 37 ℃ to OD600 = 0.6. Protein overexpression was induced by 0.4mM IPTG (Isopropyl-β-D-thiogalactoside), and the cells were incubated at 17 ℃ overnight. Cells were harvested by centrifugation, and the pellet was resuspended in buffer A (20 mM Tris pH 7.9, 500 mM NaCl, 5 mM imidazole). Cells were lysed by sonication and purified with a Ni-NTA column. Proteins with a 6xHis-MBP fusion tag were eluted by buffer B (20 mM Tris pH 7.9, 500 mM NaCl, 250 mM imidazole). The 6xHis-MBP fusion tag was cleaved by TEV protease at 4 ℃ and removed by passage over a Ni-NTA column with buffers A and B. Protein was further purified by a heparin exchange column (HiTrap Heparin-HP column, GE Healthcare Life Sciences) and dialysed into a final buffer (10 mM potassium phosphate pH 6.87, 50 mM NaCl, 1 mM DTT). RIG-I ΔCARD (242–922) was cloned into a 2S-T vector, and the protein expression and purification were performed in a manner similar to RIG-I RD, with an additional gel filtration chromatography step (Superdex-200, GE Healthcare Life Sciences) by using final buffer (50 mM HEPES pH 7.3, 50 mM NaCl, 5mM MgCl2, 1mM DTT). Full length RIG-I was cloned into a 2M-T vector, and the protein expression was performed in an E. coli BL21-Gold(DE3) strain. RIG-I was purified in a manner similar to RIG-I ΔCARD.
Crystallization
For hp-IAV alone, a 100µ M RNA sample in buffer (50 mM HEPES pH 7.3, 50 mM NaCl, 5 mM MgCl2, 1 mM DTT) was annealed at 98 ℃ and snap-cooled in ice. RNA was concentrated to 2.5 mg/mL using an Amicon filter with centrifugation. The RNA sample was mixed with an equal volume of crystallization buffer (30% PEG 4000, 200 mM ammonium sulphate) using a sitting drop vapour diffusion method. Crystals appeared after 1 week of incubation at 20 ℃. Crystals were flash-frozen using liquid nitrogen directly after having been cryoprotected with ethylene glycol.
For hp-IAV in complex with RIG-I ΔCARD, a 100µ M RNA sample in buffer (50 mM HEPES pH 7.3, 50 mM NaCl, 5 mM MgCl2, 1 mM DTT) was annealed at 98 ℃ and snap-cooled in ice. RNA was mixed with protein (RIG-I ΔCARD) in a ratio of 1.1:1. Gel filtration chromatography with Superdex-200 was performed to collect a 1:1 complex. The collected sample was concentrated to ~2 mg/mL and mixed with an equal volume of crystallization buffer (15% PEG 3350, 100 mM MES pH 6.5, 100 mM NaSCN, 20 mM sodium citrate tribasic) using a hanging drop vapour diffusion method. Crystals appeared 3 days after incubation at 20°C. Crystals were harvested after 1 week and were flash-frozen using liquid nitrogen directly after having been cryoprotected with glycerol.
Data collection and structure determination
All diffraction data were collected at the PAL (Pohang Accelerator Laboratory, Pohang, Republic of Korea) PLS-7A beamline with 0.9794 Å wavelength under cryogenic conditions. Data indexing and reduction was performed by XDS [Citation24] and HKL-2000 [Citation25]. All structures were determined using molecular replacement (MR) with Phenix [Citation26]. The RNA model for MR was modelled by Coot [Citation27]; the protein complex model for MR was a deposited structure (PDB ID: 5F9F) [Citation16]. Refinement after MR was performed in several rounds by Phenix Refinement, and model building was performed by Coot. The RNA model was built using RCrane [Citation28]. All figures with crystal structure were made in Chimera [Citation29].
NMR experiments
All NMR experiments were performed on a 700 MHz Bruker spectrometer with cryoprobe at KBSI (Korea Basic Science Institute, Ochang, Republic of Korea). All experiments were performed at 15 ℃. Protein-RNA titration experiments were performed with 1H-15N HSQC experiments. NOESY experiments with mixing times of 150 ms and 300 ms were performed for the assignment of 1D imino proton peaks. The exchange rate (kex) of each imino proton was calculated by a 1D water magnetization transfer experiment [Citation30]. T1 values of the imino protons in hp-IAV and hp-WC in the free state and the RIG-I RD-bound state were determined by inversion recovery, followed by fitting the data to an exponential function. With 20 different delay times (0 ~ 300 ms), the intensity of each imino proton was measured. The measured intensities were fitted to the equation below, where I0, I(t) are intensities at delay times of zero and t, respectively, and R1a and R1w are the longitudinal relaxation rates of each imino proton and water, respectively.
ITC
RIG-I RD was dialysed into buffer A (50 mM potassium phosphate pH 6.87, 50 mM NaCl, 5 mM β-mercaptoethanol). RNAs used in ITC experiments were annealed as mentioned above and dialysed into buffer A. A 50 µM sample of RIG-I RD was loaded into the syringe, and RNAs were loaded in the cell at 5 µM (total volume 1.408 mL). All samples were degassed before the experiment and incubated at 25 ℃. ITC experiments were performed on a VP-ITC microcalorimeter (MicroCal, Malvern Panalytical), with a total 30 injections of 10 µL each. Recorded data were analysed by Origin 7.0 (MicroCal).
DSF
RIG-I RD, ΔCARD, and full length were prepared as mentioned above. All proteins were purified by gel filtration chromatography and dialysed into buffer B (50 mM HEPES pH 7.5, 50 mM NaCl, 5 mM MgCl2, 5 mM DTT) before the experiment. All RNAs used in DSF experiments were annealed as mentioned above and dialysed into buffer B. In a 20 µL PCR tube, proteins and RNAs were mixed to a final concentration of 5 µM protein and 6 µM RNA. Mixtures were incubated at 4 ℃ for 20 min and then 5X SYPRO Orange dye (Sigma) was added. Fluorescence (excitation at 470 nm, emission at 605 nm) was measured by a CFX 96 Real-Time System (Bio-Rad) with a gradual increase of temperature from 18 ℃ to 80 ℃, with 0.5 ℃ increments, every 15 sec.
Cells
HEK293T cells used in this experiment were purchased from ATCC (American Type Culture Collection, Manassas, Virginia, United States). RIG-I knockout (KO) HEK293T cells were generated using a lentiCRISPR-V2 vector designed to target exon2 of human RIG-I (DDX58). The vector was transfected into HEK293T cells using the in vivo-jetPEI® (Polyplus-transfection, Illkirch, France) to harvest viral supernatant. HEK293T cells were transduced with viral supernatant, then selected with puromycin. To verify RIG-I KO, human RIG-I signal was analysed by western blotting using a RIG-I antibody (Cell Signalling). The sgRNA sequences were as follows: 5F, 5ʹ-CACCGGTTCCTGTTGGAGCTCCAGG-3ʹ; 3R, 3ʹ-CCAAGGACAACCTCGAGGTCCCAAA-5ʹ.
Dual luciferase reporter gene assay
HEK293T or RIG-I KO cells were seeded at a density of 5 × 105 cells/well on 12-well plates. The next day, synthetic RNA (100 pmol; hp-IAV-PS or hp-WC-PS) or polyinosine–polycytidylic acid [Poly (I:C)] (0.1 μg) was mixed with pGL3-IFN-β-FLuc (1 μg) and phRLuc-CMV (2 ng; Promega). The mixtures were transfected into the cells using lipofectamine 2000 (ThermoFisher Scientific). At 24 h after transfection, both firefly luciferase (FLuc) and Renilla luciferase (RLuc) activities were measured using a dual luciferase assay kit (Promega). Transfection efficiency was normalized by dividing FLuc activity by RLuc activity in each well. Independent experiments were performed in triplicate.
Supplemental Material
Download PDF (1.3 MB)Acknowledgments
This work was supported by the Korea Drug Development Fund under Grant KDDF-201809-04 to B.-S. C. Experiments at PLS-II were supported in part by MSICT (Ministry of Science and ICT) and POSTECH.
Disclosure statement
No potential conflict of interest was reported by the authors.
Supplementary material
Supplemental data for this article can be accessed here.
Additional information
Funding
References
- Hornung V, Ellegast J, Kim S, et al. 5ʹ-Triphosphate RNA is the ligand for RIG-I. Science. 2006;314:994–997.
- Pichlmair A, Schulz O, Tan CP, et al. RIG-I-mediated antiviral responses to single-stranded RNA bearing 5ʹ-phosphates. Science. 2006;314:997–1001.
- Kato H, Takeuchi O, Sato S, et al. Differential roles of MDA5 and RIG-I helicases in the recognition of RNA viruses. Nature. 2006;441:101–105.
- Yoneyama M, Kikuchi M, Natsukawa T, et al. The RNA helicase RIG-I has an essential function in double-stranded RNA-induced innate antiviral responses. Nat Immunol. 2004;5:730–737.
- Luo D, Ding SC, Vela A, et al. Structural insights into RNA recognition by RIG-I. Cell. 2011;147:409–422.
- Kowalinski E, Lunardi T, McCarthy AA, et al. Structural basis for the activation of innate immune pattern-recognition receptor RIG-I by viral RNA. Cell. 2011;147:423–435.
- Jiang F, Ramanathan A, Miller MT, et al. Structural basis of RNA recognition and activation by innate immune receptor RIG-I. Nature. 2011;479:423–427.
- Kohlway A, Luo D, Rawling DC, et al. Defining the functional determinants for RNA surveillance by RIG-I. EMBO Rep. 2013;14:772–779.
- Gack MU, Shin YC, Joo CH, et al. TRIM25 RING-finger E3 ubiquitin ligase is essential for RIG-I-mediated antiviral activity. Nature. 2007;446:916–920.
- Jiang X, Kinch LN, Brautigam CA, et al. Ubiquitin-induced oligomerization of the RNA sensors RIG-I and MDA5 activates antiviral innate immune response. Immunity. 2012;36:959–973.
- Desselberger U, Racaniello VR, Zazra JJ, et al. The 3ʹ and 5ʹ-terminal sequences of influenza A, B and C virus RNA segments are highly conserved and show partial inverted complementarity. Gene. 1980;8:315–328.
- Fodor E, Pritlove DC, Brownlee GG. The influenza virus panhandle is involved in the initiation of transcription. J Virol. 1994;68:4092–4096.
- Pflug A, Guilligay D, Reich S, et al. Structure of influenza A polymerase bound to the viral RNA promoter. Nature. 2014;516:355–360.
- Bae SH, Cheong HK, Lee JH, et al. Structural features of an influenza virus promoter and their implications for viral RNA synthesis. Proc Natl Acad Sci U S A. 2001;98:10602–10607.
- Lee MK, Kim HE, Park EB, et al. Structural features of influenza A virus panhandle RNA enabling the activation of RIG-I independently of 5ʹ-triphosphate. Nucleic Acids Res. 2016;44:8407–8416.
- Devarkar SC, Wang C, Miller MT, et al. Structural basis for m7G recognition and 2ʹ-O-methyl discrimination in capped RNAs by the innate immune receptor RIG-I. Proc Natl Acad Sci U S A. 2016;113:596–601.
- Varani G, McClain WH. The G x U wobble base pair. A fundamental building block of RNA structure crucial to RNA function in diverse biological systems. EMBO Rep. 2000;1:18–23.
- Tuerk C, Gauss P, Thermes C, et al. CUUCGG hairpins: extraordinarily stable RNA secondary structures associated with various biochemical processes. Proc Natl Acad Sci U S A. 1988;85:1364–1368.
- Nakano S, Hirayama H, Miyoshi D, et al. Dimerization of nucleic acid hairpins in the conditions caused by neutral cosolutes. J Phys Chem B. 2012;116:7406–7415.
- Masquida B, Westhof E. On the wobble GoU and related pairs. RNA. 2000;6:9–15.
- Lu C, Ranjith-Kumar CT, Hao L, et al. Crystal structure of RIG-I C-terminal domain bound to blunt-ended double-strand RNA without 5ʹ triphosphate. Nucleic Acids Res. 2011;39:1565–1575.
- Hwang SY, Sun HY, Lee KH, et al. 5ʹ-Triphosphate-RNA-independent activation of RIG-I via RNA aptamer with enhanced antiviral activity. Nucleic Acids Res. 2012;40:2724–2733.
- Saito T, Owen DM, Jiang F, et al. Innate immunity induced by composition-dependent RIG-I recognition of hepatitis C virus RNA. Nature. 2008;454:523–527.
- Kabsch W. Xds. Acta Crystallogr D Biol Crystallogr. 2010;66:125–132.
- Otwinowski Z, Minor W. Processing of X-ray diffraction data collected in oscillation mode. Methods Enzymol. 1997;276:307–326.
- Adams PD, Afonine PV, Bunkoczi G, et al. PHENIX: a comprehensive Python-based system for macromolecular structure solution. Acta Crystallogr D Biol Crystallogr. 2010;66:213–221.
- Emsley P, Lohkamp B, Scott WG, et al. Features and development of Coot. Acta Crystallogr D Biol Crystallogr. 2010;66:486–501.
- Keating KS, Pyle AM. RCrane: semi-automated RNA model building. Acta Crystallogr D Biol Crystallogr. 2012;68:985–995.
- Pettersen EF, Goddard TD, Huang CC, et al. UCSF Chimera–a visualization system for exploratory research and analysis. J Comput Chem. 2004;25:1605–1612.
- Lee JH, Pardi A. Thermodynamics and kinetics for base-pair opening in the P1 duplex of the Tetrahymena group I ribozyme. Nucleic Acids Res. 2007;35:2965–2974.
- Li S, Olson WK, Lu XJ. Web 3DNA 2.0 for the analysis, visualization, and modeling of 3D nucleic acid structures. Nucleic Acids Res. 2019;47:W26–W34.