ABSTRACT
Phytoene synthase (PSY) has been considered as an important regulatory enzyme in carotenoids biosynthesis pathway. Previous study finds that the yellow fruit in Solanum lycopersicum var. cerasiforme accession PI 114490 is caused by loss-of-function of SlPSY1 due to trans-splicing between SlPsy1 and an unknown gene transcribed from neighbour opposite strand DNA of SlPsy1. The genomic DNA sequences of SlPsy1 between red and yellow-fruited tomato lines have one single-nucleotide polymorphism (SNP) in the fourth intron and one SSR in the intergenic region. In the current study, the cause of trans-splicing event was further investigated. The data showed that the previously defined unknown gene was a putative long non-coding RNA ACoS-AS1 with three variants in many yellow-fruited tomato lines. The intronic SNP and intergenic SSR were tightly associated with trans-splicing event SlPsy1-ACoS-AS1. However, transgenic tomato lines carrying the genomic DNA of SlPsy1 from PI 114490 did not generate transcripts of ACoS-AS1and SlPsy1-ACoS-AS1 suggesting that only the intronic SNP could not cause the trans-splicing event. Over-expression of SlPsy1-ACoS-AS1 in red-fruited tomato line M82 did not have any phenotype change while over-expression of wild type SlPsy1 resulted in altered leaf colour. Sub-cellular localization analysis showed that SlPSY1-ACoS-AS1 could not enter plastids where SlPSY1 has its enzyme activity. Mutation of ACoS-AS1 in PI 114490 generated by CRISPR/Cas9 techniques resulted in red fruits implying that ACoS-AS1 was essential to trans-splicing event SlPsy1-ACoS-AS1. The results obtained here will extend knowledge to understand the mechanism of trans-splicing event SlPsy1-ACoS-AS1 and provide additional information for the regulation of carotenoids biosynthesis.
Introduction
Carotenoids are naturally occurring pigments in many organisms including plants, algae, and cyanobacteria. In plants, carotenoids are essential pigments responsible for colours in leaves, flowers and fruits that attract pollinators and seed dispersers, or act as photoprotectants of the photosynthetic apparatus against excess light [Citation1–Citation4]. Carotenoids also provide nutrient elements to human for health protection by serving as precursors to vitamin A and acting as antioxidants to reduce the risks of some chronic diseases [Citation5–Citation11]. Due to their vital roles in plants and benefits to human health, biosynthesis and manipulation of carotenoids in plants have been intensively investigated [Citation2–Citation4,Citation8].
Biosynthesis of carotenoids can be influenced by genes involved in the pathway or related to ripening process [Citation2]. Phytoene synthase (PSY) has been considered as the crucial enzyme for carotenoids biosynthesis because it catalyzes the first committed step in the pathway [Citation12]. In tomato (Solanum lycopersicum), three genes SlPsy1, SlPsy2, and SlPsy3 encoding phytoene synthase have been identified, but only SlPsy1 contributes to carotenoids accumulation in ripening fruits [Citation13–Citation16]. Suppression of SlPSY1 activity is sufficient to block the biosynthesis of carotenoids, which results in yellow fruits. While loss-of-function of CrtISO gene in the tangerine (t) mutant [Citation17], LCYE gene in the Delta (Del) mutant [Citation18], and LCYB gene in the Beta (B) mutant [Citation19] can alter components of carotenoids causing orange or yellow fruits in these mutants. In addition, ethylene receptor mutant Never ripe (Nr) [Citation20,Citation21], loss-of-function ethylene insensitive 2 (EIN2) mutation [Citation22], ripening defect mutants ripening inhibitor (rin) [Citation23,Citation24], Colourless non-ripening (Cnr) [Citation25], and non-ripening (nor) [Citation26] also play a central role in carotenoids accumulation and result in orange or yellow fruits.
Alternative splicing of genes involved in carotenoids biosynthesis pathway is another mechanism affecting the component and accumulation of caroteniods. Alternative splicing of lycopene-ε-cyclase pre-mRNA in Cara Cara navel orange (Citrus sinensis Osbeck) alters the carotenoids from α-carotene to lycopene and β-carotene [Citation27]. Four transcripts of Psy1 have been identified in wheat (Triticum aestivum L.), but only the normal transcript encodes a functional enzyme. The existence of other three alternative splice variants (ASV) reduces the mRNA abundance of the normal one, leading to a decrease of caroteniods biosynthesis [Citation28]. In Arabidopsis, two ASVs of Psy are generated by retaining different lengths of 5ʹ-UTR to compensate the only one Psy gene in the genome. The long ASV1 is involved in the development-dependent formation of carotenoids, while the short ASV2 is inducible when a sudden increase of carotenoids is needed [Citation29]. In tomato, ASVs of SlPsy1 have also been reported and their expressions vary significantly at different developmental stages in fruits [Citation30]. However, whether the ASVs of SlPsy1 can affect the biosynthesis or components of carotenoids remains unclear.
The formation of yellow flesh in tomato fruits is due to a great reduction of phytoene and coloured carotenoids [Citation31]. Mutation of SlPsy1 has been considered as the primary cause of yellow flesh in tomato. Loss-of-function of SlPSY1 due to insertion of a Rider in the genomic region of the SlPsy1 gene in the yellow flesh (r) mutant [Citation32,Citation33] or EMS-induced point mutation (knockout allele) in the SlPsy1 gene [Citation34] results in yellow fruits. However, other mutants such as ry with an aberrant transcript of SlPsy1 [Citation33] and r2997 with a low transcription of SlPsy1 in fruits [Citation16] are typical yellow flesh phenotype with normal genomic DNA sequence in the SlPsy1 gene region. Similarly, the expression of SlPsy1 is very low in a yellow-fruited S. lycopersicum var. cerasiforme accession PI 114490 but only one SNP exists in the fourth intron of the SlPsy1 gene between red and yellow-fruited lines [Citation35,Citation36]. Interestingly, another transcript SlPsy1/Unknown in PI 114490 caused by trans-splicing between SlPsy1 and an unknown gene transcribed from the nearby complementary DNA sequence shows a high level of transcription in fruits. Furthermore, the intronic SNP and a SSR between the SlPsy1 gene and acyl-CoA synthetase (ACoS) gene associate with yellow flesh phenotype [Citation35].
In this study, the cause of trans-splicing between SlPsy1 and ACoS-AS1 was investigated. The data showed that the gene transcribed from the neighbour complementary sequence was a long non-coding RNA ACoS-AS1 with three variants popularly existing in yellow-fruited tomato lines. The intronic SNP and intergenic SSR were tightly associated with trans-splicing between SlPsy1 and ACoS-AS1 but only the SNP could not cause trans-splicing event. Although the transcript SlPsy1-ACoS-AS1 could be translated into protein, it did not have a function as SlPSY1 in planta. Mutation of ACoS-AS1 generated by CRISPR/Cas9 techniques indicated that the transcription of ACoS-AS1 was essential to trans-splicing between SlPsy1 and ACoS-AS1. The results obtained here will extend knowledge to understand the mechanism of trans-splicing event SlPsy1-ACoS-AS1 and provide additional information for the regulation of caroteniods biosynthesis.
Results
ACoS-AS1 is a long non-coding RNA with three variants
Both Oligo (dT)18 and Oligo (dT)18-adaptor could perform reverse transcription of the SlPsy1/Unknown [35, this study]. Analysis of transcriptome data derived from PI 114490 (performed by Dr David Francis at The Ohio State University) also found a 195-bp fragment (solcap_tomato_PI114490_14479). These data suggested that mature RNA of the Unknown gene had a polyA tail.
Due to the lack of whole sequence information of the Unknown gene, a set of 15 pairs of primers (Fig. 1A, Table S1) was designed based on the genomic DNA sequence and used to amplify the transcripts of SlPsy1-/Unknown from cDNA of PI 114490 fruits. Seven primer pairs (PSY1F3/PSY1U-R1, PSY1F3/PSY1U-R2, PSY1F3/PSY1U-R3, PSY1F3/PSY1U-R4, PSY1F3/PSY1U-R8, PSY1F3/PSY1U-R9, and PSY1F3/PSY1U-R13) had PCR products. Sequence analysis of these PCR products revealed that there were three variants of the Unknown gene. Four pairs of primers (PSY1F3/PSY1U-R1, PSY1F3/PSY1U-R2, PSY1F3/PSY1U-R3, and PSY1F3/PSY1U-R4) amplified the same variant (designated as variant 1) with different lengths and the longest fragment of 1837 bp was produced by PSY1F3/PSY1U-R4. Two pairs of primers (PSY1F3/PSY1U-R8 and PSY1F3/PSY1U-R9) amplified the same variant (designated as variant 2) with different lengths and a longer fragment of 915 bp was obtained by PSY1F3/PSY1U-R9. The pair of primers PSY1F3/PSY1U-R13 amplified the third variant (designated as variant 3) of 475 bp. Excluding the sequence originating from SlPsy1, the lengths of three variants were 1588 bp, 666 bp, and 226 bp, respectively. Alignment of three variant sequences to the tomato genome of Heinz 1706 (SL3.0) (https://www.sgn.cornell.edu) found that all variants shared the first 147 bp but ended at different sites (Fig. 1B). The variant 3 was the most possible normal transcript of the Unknown gene with three exons and two introns predicted in the genomic DNA, while variants 1 and 2 were possibly alternative spliced transcripts by retaining part of the second intron (Fig. 1B). Three variants could be verified by amplifying the full length of SlPsy1/Unknown with another three pairs of primers PSY1F1/PSY1U-R4, PSY1F1/PSY1U-R9, and PSY1F1/PSY1U-R13 (Table S1) in PI 114490 (Fig. 1C). However, transcription of the Unknown gene could not be detected in red-fruited tomato lines Huangrong Red (HGR), TS-196, TS-223, Zhengyang (ZY) and Jinjue (JJ), and pink-fruited tomato line TS-35 (Fig. 1D).
Figure 1. Three transcripts of ACoS-AS1 in yellow-fruited tomato line PI 114490. A. Positions of 15 primers (Table S1) for detecting transcript variants on the opposite DNA sequence of SlPsy1 gene region. Red triangle indicates primers with the success of PCR amplification. Black box indicates exon of SlPsy1 and acyl-CoA synthase gene, while black line indicates intron and intergenic region. Primers are designed based on the reverse sequence. B. Diagram of three transcripts and their origin in the genomic sequence. Empty boxes represent potential exons, black line represents the first intron, and grey, blue, pink and green boxes represent the potential second intron. Variant 3 is the possible real transcript of ACoS-AS1, while variants 1 and 2 are alternative transcripts by retaining part of the second intron. C. Image of agarose gel for PCR products of three variants amplified from cDNA of PI 114490. Variants 1 (V1), 2 (V2) and 3 (V3) are amplified with primer pairs PSY1F1/PSY1U-R4, PSY1F1/PSY1U-R9, and PSY1F1/PSY1U-R13, respectively. D. RT-PCR detection of ACoS-AS1 in cDNA isolated from PI 114490 (PI), six red-fruited tomato lines Huangrong Red (HGR), TS-196, TS-223, Zhengyang (ZY), Jinjue (JJ), and OH 88119), and one pink-fruited tomato line TS-35.
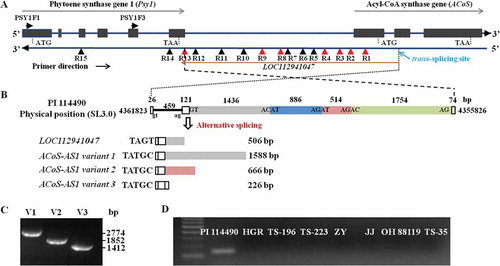
The Unknown gene started from the sequence complementary to the second exon of a gene encoding Acyl-CoA synthase (ACoS) and ended at the sequence complementary to the last exon of Psy1 (). However, no valid open reading frame (ORF) could be predicted by ORFfinder (NCBI, https://www.ncbi.nlm.nih.gov/orffinder/). Searching the NCBI nucleotide database revealed that the sequence of the Unknown gene was almost identical to an uncharacterized long non-coding RNA (lncRNA) LOC112941047 predicted from tomato RNA-seq alignments (https://www.ncbi.nlm.nih.gov/gene/?term=LOC112941047). Actually, the sequence of LOC112941047 obtained from NCBI was part of variant 1 but the first four nucleotides (TAGT) were different from those (TATGC) in all variants (). Following the guide to long non-coding RNA gene nomenclature [Citation37], the Unknown gene was designated as ACoS-AS1 because its 5ʹ-end was complementary to the second exon of ACoS gene.
cDNA sequence variation of SlPsy1 and SlPsy1-ACoS-AS1 in yellow-fruited tomato lines
According to the above and previous data [Citation35], four pairs of primers PSY1F1/PSY1R3, PSY1F1/PSY1U-R4, PSY1F1/PSY1U-R9, and PSY1F1/PSY1U-R13 (Table S1) were used to amplify cDNA derived from fruits of 55 yellow-fruited tomato lines. Based on sizes of PCR products, 23 lines had three variants of SlPsy1-ACoS-AS1 and most of them also had the normal SlPsy1 amplification as in PI 114490, 22 lines had the normal SlPsy1, two lines had one transcript amplified by PSY1F1/PSY1R3 but the sizes were smaller than the normal SlPsy1, and eight lines did not yield any PCR product (Table S2).
Analysis of 55 tomato lines with six InDel markers for tangerine, Delta, and Beta revealed that six had InDels in the CrtISO gene, but none had InDels in LCYE and LCYB genes (Table S2). In addition, the six tomato lines with InDels in the CrtISO gene did not show sequence variation in SlPsy1 gene (Table S2). Combining the observation of fruit colour, they were probably the tangerine type mutants and thus excluded for the following analysis. The remaining 49 tomato lines with yellow fruits were temporally considered as a mutation of SlPsy1.
SlPsy1 showed more sequence variation than SlPsy1-ACoS-AS1 in yellow-fruited tomato lines. Of the 23 tomato lines with three SlPsy1-ACoS-AS1 variants and normal SlPsy1 transcript, only two had sequence variations in SlPsy1-ACoS-AS1 (Table S2). Yellow Bell had a 9-bp deletion in variant 3, while LA3092 had three SNPs in variant 1. Interestingly, there was no variation in all detectable SlPsy1 in these 23 lines. However, of 16 tomato lines with the normal size of SlPsy1 amplification, eight lines had premature termination codon mutation, and one (Yellow Che) had one SNP, two PI128216-EMS mutants had two SNPs, and five lines had normal sequence (Table S2).
The intronic SNP and the intergenic SSR are associated with but only the SNP cannot cause trans-splicing between SlPsy1 and ACoS-AS1
Among 294 re-sequenced tomato lines [Citation38], 207 (70.4%) including 173 red-fruited lines, 23 pink-fruited lines, 9 yellow-fruited lines, and 2 green-fruited lines had no sequence variation in 5ʹ-UTR, CDS, and 3ʹ-UTR of the SlPsy1 gene region (Table S3). A total of 57 SNPs were found in the remaining 87 tomato lines. All SNPs presented in 70 red-fruited tomato lines and 14 SNPs were in 12 yellow-fruited tomato lines (). There were 29 SNPs unique to red-fruited lines but none was unique to yellow, pink or green fruit lines. The previously reported SNP (A/G) in the fourth intron of SlPsy1 gene between PI 114490 (G) and OH 88119 (A) [Citation36] was also presented. The 12 yellow-fruited lines with SNPs had the nucleotide G, while the remaining nine had nucleotide A. Surprisingly, three red-fruited lines TS-196, TS-280, TS-20, and one pink-fruited line TS-35 also had the nucleotide G. Sequence analysis of PCR products amplified from these four lines confirmed the G in three lines but the G in line TS-196 (Alisa Craig) should be an A. Therefore, 57.1% yellow-fruited lines had G and only 1.1% red-, pink-, and green-fruited lines had G, suggesting that the fourth intronic SNP was probably associated with yellow fruit.
Figure 2. Venn diagram shows the number of single nucleotide polymorphisms (SNPs) in red-, pink-, yellow-, and green-fruited tomato lines. The numbers in parentheses are a number of lines have SNPs/total lines used for comparison.
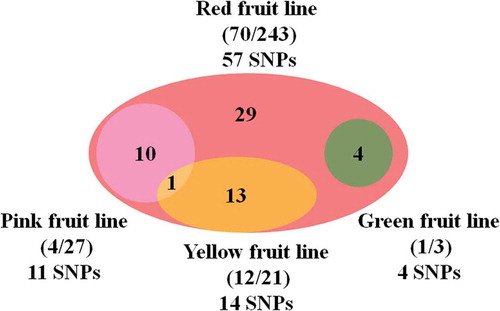
The intronic SNP was also analysed by sequencing PCR products amplified from genomic DNA of yellow-fruited lines in Table S2. Interestingly, of the 20 lines with three SlPsy1-ACoS-AS1 variants checked for the SNP, 18 had G and two F1s were heterozygotes of A/G. On the contrary, only one line in the tangerine mutant group had G but without SlPsy1-ACoS-AS1 transcript (Table S2). Genotypes of the intergenic SSR in 46 yellow-fruited tomato lines were determined by PCR analysis [Citation35]. The genotypes of SSR were completely consistent with the SNP (G) allele in all analysed tomato lines (Table S2). These data suggested that the intronic SNP and intergenic SSR were potentially associated with the existence of trans-splicing between SlPsy1 and ACoS-AS1.
To further determine the relationship between the intronic SNP and trans-splicing event of SlPsy1-ACoS-AS1, the genomic DNA (3302 bp from start codon ATG to spot codon TAA) of SlPsy1 gene from PI 114490 was introduced into a red-fruited tomato line OH 88119 without the SlPsy1-ACoS-AS1 transcript through genetic transformation. Although the expression of SlPsy1 gene in the transgenic lines was significantly increased in fruits, the fruit colour was red and no transcription of SlPsy1-ACoS-AS1 and ACoS-AS1 was detected (). These data indicated that only the intronic SNP could not cause trans-splicing between SlPsy1 and ACoS-AS1.
Figure 3. Only intronic SNP does not cause trans-splicing between SlPsy1 and ACoS-AS1. A. Fruit colour in transgenic lines with the gPIPsy1-OE construct containing the genomic DNA of SlPsy1 from PI 114490. B. Expression analysis of SlPsy1 (primer pair PSY1F3/R3), SlPsy1-ACoS-AS1, and ACoS-AS1 in transgenic lines, OH 88119 and PI 114490 revealed by RT-PCR. C. Relative expression of SlPsy1 in mature green fruits of OH 88119 and transgenic lines revealed by quantitative real-time PCR analysis. Gene expression (means ± SD, n = 3) is determined relative to transcriptional levels of EF1α in the same sample.
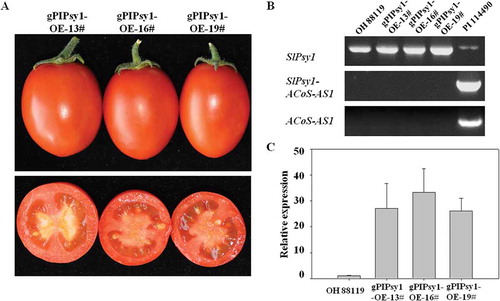
SlPSY1-ACoS-AS1 does not have a function as SlPSY1 in planta
Transformation of SlPsy1-ACoS-AS1 amplified from cDNA of PI 114490 into a red-fruited tomato line M82 was performed to investigate whether SlPSY1-ACoS-AS1 can function as SlPSY1 in planta. At least five independent transgenic lines were obtained for each construct of cPsy1-OE1 (SlPsy1-ACoS-AS1 CDS), cPsy1-OE2 (SlPsy1-ACoS-AS1 CDS-3ʹUTR) and cPsy1-OE3 (wild type SlPsy1). Ripe fruits of all transgenic plants were red (). However, the colour of young leaves in transgenic plants of cPsy1-OE3 showed altered colour similar to previous discovery [Citation39], while transgenic lines from cPsy1-OE1 and cPsy1-OE2 did not show this phenotype () and no transcription of ACoS-AS1 was detected in the transgenic lines (). These data suggested that SlPSY1-ACoS-AS1 might not have enzyme activity as SlPSY1 in planta.
Figure 4. Over-expression of SlPsy1-ACoS-AS1 in M82 does not change fruit colour. A. Representative fruit images of wild type M82, and transgenic lines expressing SlPsy1-ACoS-AS1 (cPsy1-OE1-1201# and cPsy1-OE2-1231#) and SlPsy1 (cPsy1-OE3-1218#). B. Representative leave images of wild type M82 and transgenic lines expressing SlPsy1-ACoS-AS1 (cPsy1-OE1-1201# and cPsy1-OE2-1231#) and SlPsy1 (cPsy1-OE3-1218#). C. Expression of ACoS-AS1 and SlPsy1 (primer pair qPsy1F1/R1) in PI 114490, M82, and transgenic lines expressing SlPsy1-ACoS-AS1 (cPsy1-OE1 and cPsy1-OE2) and SlPsy1 (cPsy1-OE3) revealed by RT-PCR.
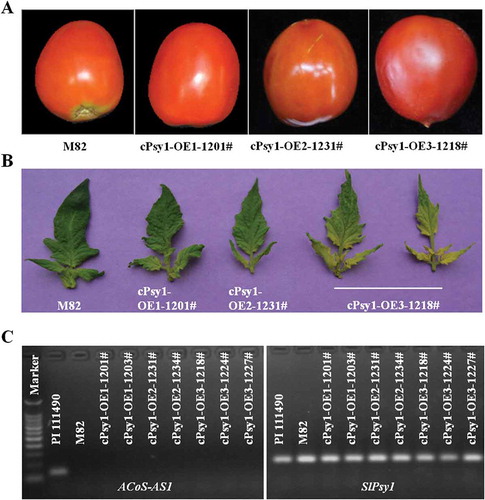
Then, subcellular localizations of SlPSY1 and SlPSY1-ACoS-AS1 were performed by Agrobacterium-mediated transient expression in leaf and protoplast of N. benthamiana. As expected, SlPSY1-ACoS-AS1-GFP protein was larger than wild type SlPSY1-GFP protein (Fig. S1) because the deduced protein sequence of SlPSY1-ACoS-AS1 was 20 amino acid longer than that of SlPSY1 [Citation35]. Both proteins were in the cytoplasm (). Wild type SlPSY1-GFP overlapped with plastids (chloroplast), while SlPSY1-ACoS-AS1-GFP did not (, Fig. S2), suggesting that SlPSY1-ACoS-AS1 could not get into plastids to have its function.
Figure 5. Transient expression of SlPSY1-GFP and SlPSY1-ACoS-AS1-GFP fusion proteins in leaves of Nicotiana benthamiana plants. The expression cassettes driven by 35S promoter were cloned into pCAMBIA1300 vector. Cell nuclei were stained with DAPI (4ʹ,6-diamidino-2-phenylindole), while chloroplasts were stained with CHL (chlorophyll autofluorescence). (Scale bars, 10 μm.).
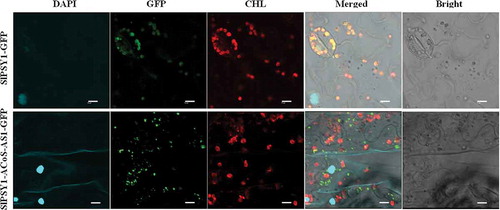
Transcription of ACoS-AS1 can be interrupted by sequence editing
The formation of SlPsy1-ACoS-AS1 needs two steps, transcription of ACoS-AS1 and trans-splicing between SlPsy1 and ACoS-AS1. The above data indicated that there was no transcription of ACoS-AS1 in red-fruited tomato lines (). Thus, it seemed that the transcription of ACoS-AS1 was essential to trans-splicing between SlPsy1 and ACoS-AS1. To test this hypothesis, the CRISPR/Cas9 editing technique was adopted to knockout ACoS-AS1 in PI114490 with two target sites T1 and T2 (). Four independent mutants with different sizes of fragmental deletions were obtained. However, only two lines borne fruits with seeds. Mutant TA-12 had 5-bp deletion at the position of target site T1 () and its fruits were red at ripe stage (), but fruits of mutant TA-2 with 1-bp deletion at the same site as TA-12 kept yellow (,). The content of total carotenoids dramatically increased in TA-12 mutant comparing to PI 114490, while it in TA-2 was low ().
Figure 6. Editing ACoS-AS1 using CRISPR/Cas9 technique changes the fruit colour. A. Schematic illustration of two target sites in the genome sequence. B. Sequence of target sites in two mutants TA-2 and TA-12 generated by CRISPR/Cas9 editing. The target sites are underlined, dash (-) represents deleted nucleotide, and red letters represented the protospacer adjacent motif. C. Fruit images of PI 114490, TA-2, TA-12, and OH 88119. D. Total carotenoids (means ± SD, n = 3) in fruits of PI114490, TA-2, TA-12 and OH 88119.
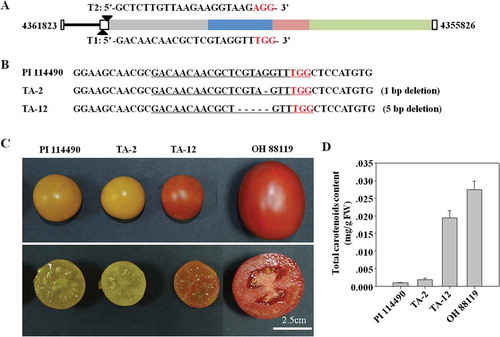
RT-PCR analysis revealed that SlPsy1 was expressed in PI 114490, TA-2, TA-12, and OH 88119, while ACoS-AS1 and SlPsy1-ACoS-AS1 were only expressed in PI 114490 (). Western blot analysis showed that SlPSY1 protein was detected in read-fruited lines OH 88119 and TA-12, but its level inTA-12 was lower than in OH 88119 (). These results suggested that transcription of ACoS-AS1 was essential to trans-splicing between SlPsy1 and ACoS-AS1.
Figure 7. Expression of SlPsy1, ACoS-AS1, and SlPsy1-ACoS-AS1 in fruits of two mutants TA-2 and TA-12 generated by CRISPR/Cas9 editing as well two wild types PI 114490 and OH 88119. A. Expression of SlPsy1, ACoS-AS1, and SlPsy1-ACoS-AS1 at RNA level detected by RT-PCR. B. Expression of SlPSY1 detected by western blot.
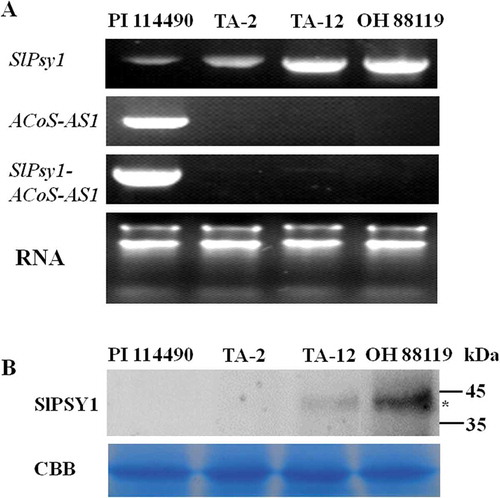
Discussion
The record of yellow-fruited tomatoes can be dated back to 1623 [Citation40]. Due to spontaneous mutation and selection in a long history, the colours of yellow fruits vary from almost creamy yellow to bright or orange with different nutritional profiles depending on varieties/lines. Various mutants including yellow flesh (r), tangerine (t), Delta (Del), Beta (B), Never ripe (Nr), loss-of-function ethylene insensitive 2 (EIN2), ripening inhibitor (rin), Colourless non-ripening (Cnr), non-ripening (nor) with yellow or orange fruit colour related to carotenoids biosynthesis have been reported [Citation17–Citation26,Citation33]. Although most mutants have their unique components of carotenoids, it is not easy to visually distinguish them because some mutants have similar fruit colour. In the current study, a collection of 56 yellow-fruited tomato lines including PI 114490 showed a wide range of fruit colour (Fig. S3). The data showed that the colour was not associated with the gene responsible for it. For example, the colour of Jinmiyingtao and Jinman was golden-yellow but their genotypes were different. Jinmiyingtao had a premature termination codon mutation in the SlPsy1 gene, while Jinman had normal SlPsy1 transcript (Table S2). With the help of molecular markers, six lines could be determined as tangerine type mutant. However, the cause of yellow colour in eight lines could not be determined yet (Table S2). Of the remaining 42 yellow-fruited lines examined in this study, approximately 57.0% were caused by trans-splicing event SlPsy1-ACoS-AS1, 19.0% were probably r mutant, and 24.0% had ORF shift due to premature termination codon mutation or deletion (Table S2). These results suggested that trans-splicing between SlPsy1 and ACoS-AS1 was probably a major type of SlPsy1 mutation in nature.
Low-level expression of SlPsy1 in fruits has been reported in r2997 mutant but there is no sequence variation unique to the mutant [Citation16]. In this study, three types of SlPsy1 mutation could be positively determined. Both r mutant and ORF shift due to premature termination codon mutation or deletion caused low-level expression or loss-of-function of SlPSY1 protein. The trans-splicing event significantly reduced the level of SlPsy1 expression [Citation35]. Although no expression of ACoS-AS1 and SlPsy1-ACoS-AS1 were detected in the TA-2 mutant generated by CRISPR/Cas9 editing, the expression of wild type SlPsy1 was also low and there was no measurable protein (B). Therefore, the fruit colour remained yellow. These data indicated that a low level of SlPSY1 protein resulting in low accumulation of phytoene and coloured carotenoids was one of the main causes of yellow fruits in tomato.
Abnormal transcription of SlPsy1 in ry mutant has been discovered more than 25 years [Citation33]. However, the origin of the fragment at the 3ʹ-end of the abnormal transcript was not determined before the availability of tomato genome sequence data. Alignment of the abnormal transcript sequence to the genomic DNA sequence revealed that the fragment was transcribed from the opposite DNA sequence nearby SlPsy1 [Citation35, ]. Current work on searching the sequence in NCBI database found a predicted lncRNA LOC112941047 (posted on 8 August 2018) with 4–5 nucleotides difference at the beginning of the sequence (). Further investigation suggested that transcription of ACoS-AS1 was crucial to trans-splicing between SlPsy1amd ACoS-AS1 to form the SlPsy1-ACoS-AS1 transcript. It has been shown that trans-splicing events JAZF1-JJAZ1 and SLC45A3-ELK4 in human can be translated into proteins associated with antiapoptotic effects and prostate cancer, respectively [Citation41,Citation42]. Both previous finding of the partial function of SlPSY1-ACoS-AS1 in E. coli [Citation35] and sub-cellular localization analysis in this study indicated that SlPsy1-ACoS-AS1 could also be translated into protein. However, the substitution at 3ʹ-end of SlPsy1 prevented the protein entering the plastids, where wild type SlPSY1 had its proper function [Citation12,Citation43]. Over-expression of SlPsy1 resulted in altered leaf colour, while the stable transformation of SlPsy1-ACoS-AS1 did not cause this phenomenon, indicating that the protein did not have enzyme activity as SlPSY1in planta.
Alternative splice variants of Psy1 due to cis-splicing (splicing within a molecule) have been frequently discovered in several plants including tomato [Citation27–Citation30]. However, trans-splicing (splicing between different molecules) between Psy1 and other genes is uniquely reported in yellow-fruited tomato lines [Citation35]. Indeed, the trans-splicing process in higher eukaryotes remains unclear though events have been described for various species [Citation44–Citation46]. Unlike other events in higher eukaryotes that trans-splicing occurs between two coding genes such as mod(mdg4) in Drosophila [Citation47], bursicon gene in Anopheles gambiae [Citation48], eri-6/7 mRNA in Caenorhabditis elegans [Citation49], and JAZF1-JJAZ1 and SLC45A3-ELK4 in human [Citation41,Citation42], the trans-splicing event SlPsy1-ACoS-AS1 involved the lncRNA ACoS-AS1 that was uniquely detected in certain type of yellow-fruited tomato lines. This makes the process of trans-splicing more complicated. Although SlPsy1 and ACoS-AS1 were coded by opposite DNA strand as mod(mdg4) in Drosophila [Citation47], there were a SNP tightly linked to the trans-splicing event as lola in Drosophila [Citation50] and an intergenic SSR associated with trans-splicing event as eri-6/7 mRNA in C. elegans [Citation49], none of these previously proposed models could be used to interpret the SlPsy1-ACoS-AS1 event [Citation35]. Over-expression of the genomic DNA containing the SNP in red-fruited tomato line OH 88119 did not yield transcripts of ACoS-AS1 and SlPsy1-ACoS-AS1 (), indicating that only the SNP could not cause trans-splicing event SlPsy1-ACoS-AS1 though the SNP was tightly associated with the event. Mutation of sequence for ACoS-AS1 by CRISPR/Cas9 editing blocked the transcription of ACoS-AS1 and the formation of SlPsy1-ACoS-AS1 resulting in red fruit in mutated lines (). These results indicated that target 1 region () was probably important for transcription of ACoS-AS1 that was a prerequisite of trans-splicing event SlPsy1-ACoS-AS1. The remaining question was why the SNP and SSR co-appeared with trans-splicing event SlPsy1-ACoS-AS1. One possible interpretation could be that existences of SNP and SSR as well as other sites like target 1 might be necessary for the DNA fragment to be recognized by certain transcription initiation factors in this type of yellow-fruited tomato lines. Once the transcription occurs, the resulting lncRNA ACoS-AS1 can fulfill trans-splicing with SlPsy1 either by itself or under the help of other factors.
In conclusion, the trans-splicing event SlPsy1-ACoS-AS1 is one of the major natural causes of yellow fruit in tomato. SlPsy1-ACoS-AS1 can be translated into protein but the protein cannot enter plastids to have its function in planta. Co-existence of intronic SNP, intergenic SSR and other regions might be required for transcription of ACoS-AS1 and trans-splicing event SlPsy1-ACoS-AS1. The data obtained here provide additional information for understanding the mechanism of trans-splicing event SlPsy1-ACoS-AS1 and the regulation of carotenoids biosynthesis.
Experimental procedures
Plant materials
Solanum lycopersicum var. cerasiforme accession PI 114490 with yellow fruit caused by trans-splicing between SlPsy1 gene and an unknown gene [Citation35] was used for initial investigation of transcript variants in the SlPsy1 gene region. Two red-fruited tomato lines OH 88119 and M82 were used for genetic transformation. An additional set of 55 yellow-fruited tomato lines (Table S2) including r and ry mutant [Citation33] collected from various sources was subjected to colour observation and analysis of transcript variation in the SlPsy1 gene region. Three mutants, tangerine (LA3183), Delta (LA2996A), and Beta (LA0348) were also included as controls for marker analysis to detect lines potentially carrying these mutations.
All seeds were germinated in 128 Square Plug Trays in a mixture of peat and vermiculite. Seedlings were transplanted to a greenhouse or field 45 days after sowing. Plants in the same experiment were grown simultaneously under the same conditions with water and fertilizers supplied.
RNA isolation and cDNA synthesis
Due to expressions of both SlPsy1 and SlPsy1-ACoS-AS1 (previous SlPsy1/Unknown) in tomato fruits reach a peak at ripe stage [Citation35], total RNA was isolated from tomato fruits at this stage using the Quick RNA Isolation Kit (Huayueyang Biotechnology (Beijing) Co., Beijing, China) following the manufacturer’s instruction. The RNA integrity was monitored by 1.2% (w/v) agarose gel electrophoresis and the concentration was determined using a Nanodrop 2000 Spectrophotometer (Thermo Fisher Scientific, DE, USA). Single-stranded cDNA was synthesized using either SuperScriptTM III Reverse Transcriptase Kit (InvitrogenTM, Life Technologies, Carlsbad, California, USA) or PrimeScript™RT reagent Kit with gDNA Eraser (TaKaRa Bio Inc., Dalian, Liaoning, China) following the manufacturer’s instruction. Both the 6 mers random primer provided in the Kit and oligo(dT)18 with adaptor (Table S1) were used for reverse transcription.
Development of transgenic lines
Previous study shows that SlPSY1-ACoS-AS1 protein had a partial function in Escherichia coli and the SNP (A/G) in the fourth intron of SlPsy1 gene in PI 114490 was tightly associated with the trans-splicing event SlPsy1-ACoS-AS1 [Citation35]. To check whether SlPSY1- ACoS-AS1 has a function as SlPSY1 in planta, three constructs were created for genetic transformation. The 1299-bp open reading frame (ORF) of the SlPsy1-ACoS-AS1 and a fragment of 1590-bp including the ORF and partial 3ʹUTR of the SlPsy1-ACoS-AS1 [Citation35] amplified from cDNA of PI 114490 fruits were separately inserted into the vector of pCAMBIA1304 resulting in two constructs cPsy1-OE1 (pCaMV35S::Psy1-ACoS-AS1 CDS, Fig. S4A) and cPsy1-OE2 (pCaMV35S::Psy1-ACoS-AS1 CDS-3ʹUTR, Fig. S4B). The ORF fragment (1239 bp) of SlPsy1 amplified from cDNA of red-fruited tomato line OH 88119 was inserted into the vector of pCAMBIA1304 resulting in the third construct cPsy1-OE3 (pCaMV35S::Psy1CDS, Fig. S4C), which was used as a control of over-expression of wild type SlPsy1. All primer pairs (Table S1) used for gene-specific amplification contained restriction sites of BamH I and Sac I, respectively.
To determine whether the SNP (A/G) in the fourth intron is the cause of trans-splicing between SlPsy1 and ACoS-AS1, a construct gPIPsy1-OE (pCaMV35S::PIPsy1gDNA, Fig. S4D) was generated by inserting the genomic DNA of SlPsy1 into the vector of pH7WG2D.1 [Citation51]. The genomic DNA fragment (3302 bp from ATG to TAA) was amplified from PI 114490 using primers (Table S1) with recombination exchange sites.
All destination constructs were confirmed by sequencing, separately transformed into Agrobacterium tumefaciens strain C58 or GV3021 using electroporation, and then transformed into the tomato lines OH 88119 or M82 using the methods previously described [Citation52] with slight modifications. The transgenic tomato lines were verified by PCR using primers (Table S1) specific to each construct.
Marker development and analysis for detecting tangerine, Delta and Beta mutants
Various mutants have the similar phenotype of yellow fruit but caused by mutation of different genes. At least four types of mutants including r (SlPsy1 mutation), t (tangerine, CRTISO mutation), Delta (LCYE mutation), and Beta (LCYB mutation) have been characterized to date [Citation17–Citation19,Citation33]. Several molecular markers were developed here for discriminating the yellow fruits of tomato lines due to mutation of SlPsy1 from mutants of tangerine, Delta and Beta. The primers used for detecting tangerine mutant were designed based on deletion in tangerine3183 mutant and deletion in tangerinemic mutant of the CRTISO gene [Citation17], while primers for detecting Beta and Delta mutants were designed by comparing the sequences of genes encoding lycopene beta-cyclase (AF254793) and lycopene epsilon-cyclase (Y14387) to the genome sequence of LA716 (https://www.sgn.cornell.edu). Two pairs of primers for detecting potential InDels were designed for each gene (Table S1).
Genomic DNA was isolated from fresh-collected young leaves using a modified CTAB method. PCR was conducted in a 20-μl reaction volume consisting of 1 μl DNA template, 1 μl each of forward and reverse primers, 10 μl 2x Master Mix (Beijing ComWin Biotech Company, Beijing, China) and 8 μl ddH2O. PCR was performed and PCR products were visualized using the methods described in Yang et al. [Citation53].
Amplification of lncRNA ACoS-AS1 from PI 114490
Potential variants of SlPsy1-ACoS-AS1 transcript were detected during the initial amplification of the transcript from the cDNA of PI 114490 fruits. To verify this observation, a total of 15 reverse primers (PSY1U-R1 to PSY1U-R15, Table S1, ) combining with the forward primer PSY1F3 (Table S1) were used to amplify the cDNA derived from PI 114490 fruits. Due to a lack of available cDNA sequence data in this region, the 15 primers were designed based on the genomic DNA sequence of Heinz 1706 (https://www.sgn.cornell.edu). PCR was performed in 50-μl reaction volume containing 0.5 μl (5 U/μl) TaKaRa LA Taq DNA polymerase (Takara, Japan), 5 μl 10 x LA Taq Buffer II (Mg2+ Plus), 8 μl dNTP Mixture (2.5 mM each), 1 μl (10 mM/μl) of each forward and reverse primer, 5 μl of diluted cDNA template, and 29.5 μl ddH2O. PCR reaction was conducted using a program consisting of 95°C for 5 min, 38 cycles of 30 s at 95°C, 30 s at 53°C, and 3 min at 72°C, followed by 72°C for 5 min. The PCR products were visualized on 1% agarose gel, purified from the gel using TIANgel Midi Purification Kit (TIANGEN Biotech, Beijing, China), ligated into pGEM-T Easy Vector (Promega (Beijing) Biotech Co., Beijing, China), and sequenced using a ABI 3730 (Applied Biosystems, Foster City, California, USA). To verify the PCR products obtained above, three pairs of primers PSY1F1/PSY1U-R4, PSY1F1/PSY1U-R9, and PSY1F1/PSY1U-R13 (Table S1) were used to amplify the full length of SlPsy1-ACoS-AS1 variants from cDNA of PI 114490 fruits.
Amplification and sequencing of SlPsy1 and SlPsy1-ACoS-AS1 from yellow-fruited tomato lines
Based on the success of PCR amplification and sequence variation detected in PI 114490, four pairs of primers PSY1F1/PSY1R3, PSY1F1/PSY1U-R4, PSY1F1/PSY1U-R9, and PSY1F1/PSY1U-R13 (Table S1) were applied to amplification of cDNA isolated from all 56 yellow-fruited tomato lines including PI 114490 as a control. PSY1F1/PSY1R3 was used to amplify the wild type SlPsy1 and the other three were used to amplify SlPsy1-ACoS-AS1 variants. The PCR was performed and PCR products were sequenced using the method described above.
All variants were verified using another set of four pairs of primers PSY1F3/PSY1R3, PSY1F3/PSY1U-R4, PSY1F3/PSY1U-R9, and PSY1F3/PSY1U-R13 (Table S1). The PCR was conducted in a 20-μl reaction volume containing 2 μl diluted cDNA template, 1 μl (10 mM/μl) of each forward and reverse primer, 8 μl Taq10 2× Master Mix (Ausable Biotechnology, Beijing, China), and 8 μl ddH2O. The reaction was performed by initial denaturing at 95°C for 5 min followed by 32 cycles of 95°C for 30 s, 53°C for 30 s, and 72°C for 45 s. The final reaction was extended at 72°C for 5 min. PCR products were visualized on 1% agarose gel and photographed using Gel Documentation and Image Analysis System (Beijing Sage Creation Science Co., Beijing, China).
SNP(A/G) detection and SSR analysis in yellow-fruited tomato lines
The SNP (A/G) in the fourth intron of SlPsy1 gene was genotyped by direct sequencing PCR products amplified from genomic DNA using a pair of primers LEGTOM5cF1/R1 [Citation36]. The intergenic SSR discovered previously was genotyped using the method described in Kang et al. [Citation35].
RT-PCR and qRT-PCR analysis
Expressions of SlPsy1, ACoS-AS1, and SlPsy1-ACoS-AS1 were analysed using either RT-PCR or real-time quantitative reverse transcription (qRT-PCR) depending on experiments. RT-PCR was conducted using the methods described in Kang et al. [Citation35]. For qRT-PCR analysis, 1 μg RNA was reversely transcribed into cDNA using a PrimeScript™ RT Master Mix (Takara). qRT-PCR was carried out using TB Green™ Premix Ex Taq™ (Tli RNaseH Plus) (Takara) in 384-well blocks with the QuantStudioTM 6 Flex System (ThermoFisher Scientific, USA) following the manufacturer’s protocols. The expression of the EF1α gene was used as an internal control. All primers used for RT-PCR and qRT-PCR analysis were listed in Table S1.
Sub-cellular localization
The ORF without stop codon of SlPsy1 in OH 88119 and SlPsy1-ACoS-AS1 in PI114490 were amplified and separately inserted into a modified pCAMBIA 1300 vector (pCAMBIA1300-ProSuper) [Citation54] to generate green fluorescent protein (GFP)-fusion constructs (ProSuper1300-SlPSY1-GFP and ProSuper1300-SlPsy1-ACoS-AS1-GFP). The resulting constructs were verified by sequencing and introduced into A. tumefaciens strain GV3101 through electroporation. The Agrobacterium containing each construct was separately infiltrated into leaves of six-week-old Nicotiana benthamiana plants. Subcellular localization in the epidermis was observed using Olympus BX 51 fluorescence microscope (Olympus Corporation, Tokyo, Japan) 24 h after infiltration. Protoplasts were isolated from the infiltration location [Citation55] and visualized using the same microscope (Olympus). Expression of SlPSY1-GFP and SlPsy1-ACoS-AS1-GFP was detected using anti-GFP-tag mouse monoclonal antibody (CoWin Biotech Co. Ltd., Jiangsu, China) by western blot described below.
Knock-out ACoS-AS1 through CRISPR/Cas-9 editing
A modified expression vector pCBC-DT1T2_tomatoU6 was used to generate mutations of ACoS-AS1 with CRISPR/Cas9 system [Citation56]. Guide RNA sites (Table S1, ) were determined following the recommendation from the website of http://crispr.hzau.edu.cn/CRISPR2/. Mutants generated by CRIPSR/Cas9 in T0 was detected by sequencing the PCR products of target region, and in T1 and T2 generations were verified using a PCR-based assay with primers specific to the target region (Table S1).
Total carotenoids measurement
Total carotenoids were extracted from freshly harvested tomato fruits at the red ripe stage with 95% ethyl alcohol. The content of total carotenoids was determined by spectrophotometer using a previously described method [Citation36].
Western blot analysis
Total protein was isolated from tomato fruits using the Tissue Protein Extraction Kit (Beijing ComWin Biotech Company, Beijing, China) following its protocol. The supernatant was used for western blot after centrifugation at 12,000 g for 20 min at 4°C. Proteins were separated using 10% sodium dodecyl sulphate (SDS)-PAGE gels, transferred to a polyvinylidene difluoride membrane, and incubated overnight at 4°C with skim milk powder [Citation57]. The monoclonal antibody against the SlPSY1 protein was prepared by screening four peptide antigens designed by hydrophobic and antigenicity analysis, homology comparison analysis, and the effective peptide antibody sequence was LVPPTKTASLQR (YouKe Biotech, Shanghai, China). The peptide antibody against the SlPSY1 protein was at a concentration of 1:5000. An enhanced Chemiluminescence Kit (Beijing ComWin Biotech Company, Beijing, China) was used for detection after incubation with the horseradish peroxidase-conjugated secondary antibody (YouKe Biotech).
Survey of genomic DNA sequence variation of SlPsy1 gene in tomato germplasm
Single nucleotide variation (SNV) in 5ʹ-UTR, coding, and 3ʹ-UTR regions of the SlPsy1 gene were analysed in 294 tomato lines [Citation38] including 243 red-fruited, 23 pink-fruited, 21 yellow-fruited and 3 green-fruited lines. The SNV data were obtained from SOL Genomics Network (https://www.sgn.cornell.edu). Fruit colour data were kindly provided by the tomato breeding group in the Institute of Vegetable and Flowers of Chinese Academy of Agricultural Sciences (Beijing, China).
Author contributions
YX, BK, HX, HS and WY designed the study; YX, BK, ML and LX performed the experiments; YX, BK, LX, HX, HS and WY analyzed the data. WY coordinated the study. YX, BK, HX, HS and WY contributed to the writing of the manuscript.
Disclosure of Potential Conflicts of Interest
No potential conflicts of interest were disclosed.
Supplemental Material
Download PDF (379.2 KB)Acknowledgments
The authors would like to thank all persons and institutes listed in Table S2 for providing tomato seeds, as well as Dr David M. Francis for his help in blasting the transcriptome of PI 114490. The work was partially supported by the National Key Research and Development Program of China (2018YFD1000800), and the Construction of Beijing Science and Technology Innovation and Service Capacity in Top Subjects (CEFF-PXM2019_014207_000032) and 111 projects (B17043).
Supplementary material
Supplemental data for this article can be accessed here.
Additional information
Funding
References
- Cao HB, Luo HM, Yuan H, et al. A neighboring aromatic-aromatic amino acid combination governs activity divergence between tomato phytoene synthases. Plant Physiol. 2019;180:1988–2003.
- Liu LH, Shao ZY, Zhang M, et al. Regulation of carotenoid metabolism in tomato. Mol Plant. 2015;8:28–39.
- Nisar N, Li L, Lu S, et al. Carotenoid metabolism in plants. Mol Plant. 2015;8:68–82.
- Wurtzel ET. Changing form and function through carotenoids and synthetic biology. Plant Physiol. 2019;179:830–843.
- Elvira-Torales LI, Garcia-Alonso J, Periago-Caston MJ. Nutritional importance of carotenoids and their effect on liver health: a review. Antioxidants. 2019;8:229.
- Fraser PD, Bramley PM. The biosynthesis and nutritional uses of carotenoids. Prog Lipid Res. 2004;43:228–265.
- Landrum JT, Bone RA. Dietary lutein and zeaxanthin: reducing the risk for macular degeneration. Agro Food Ind Hi Tech. 2004;15:22–25.
- Rodriguez-Concepcion M, Avalos J, Bonet ML, et al. A global perspective on carotenoids: metabolism, biotechnology, and benefits for nutrition and health. Prog Lipid Res. 2018;70:62–93.
- Salehi B, Sharifi-Rad R, Sharopov F, et al. Beneficial effects and potential risks of tomato consumption for human health: an overview. Nutrition. 2019;62:201–208.
- Soares NDP, Elias MD, Machado CL, et al. Comparative analysis of lycopene content from different tomato-based food products on the cellular activity of prostate cancer cell lines. Foods. 2019;8:201.
- Stahl W, Sies H. Antioxidant activity of carotenoids. Mol Aspects Med. 2003;24:345–351.
- Cunningham FX Jr., Gantt E. Genes and enzymes of carotenoid biosynthesis in plants. Ann Rev Plant Physiol Plant Mol Biol. 1998;49:557–583.
- Fraser PD, Kiano JW, Truesdale MR, et al. Phytoene synthase-2 enzyme activity in tomato does not contribute to carotenoid synthesis in ripening fruit. Plant Mol Biol. 1999;40:687–698.
- Fraser PD, Truesdale MR, Bird CR, et al. Carotenoid biosynthesis during tomato fruit development (evidence for tissue specific gene expression). Plant Physiol. 1994;105:405–413.
- Giorio G, Stigliani AL, D’Ambrosio C. Phytoene synthase genes in tomato (Solanum lycopersicum L.): new data on the structures, the deduced amino acid sequences and the expression patterns. Febs J. 2008;275:527–535.
- Kachanovsky DE, Filler S, Isaacson T, et al. Epistasis in tomato color mutations involves regulation of phytoene synthase 1 expression by cis-carotenoids. Proc Natl Acad Sci USA. 2012;109:19021–19026.
- Isaacson T, Ronen G, Zamir D, et al. Cloning of tangerine from tomato reveals a carotenoid isomerase essential for the production of beta-carotene and xanthophylls in plants. Plant Cell. 2002;14:333–342.
- Ronen G, Cohen M, Zamir D, et al. Regulation of carotenoid biosynthesis during tomato fruit development: expression of the gene for lycopene epsilon-cyclase is down-regulated during ripening and is elevated in the mutant Delta. Plant J. 1999;17:341–351.
- Ronen G, Carmel-Goren L, Zamir D, et al. An alternative pathway to β-carotene formation in plant chromoplasts discovered by map-based cloning of Beta and old-gold color mutations in tomato. Proc Natl Acad Sci USA. 2000;97:11102–11107.
- Liu L, Wei J, Zhang M, et al. Ethylene independent induction of lycopene biosynthesis in tomato fruits by jasmonates. J Exp Bot. 2012;63:5751–6571.
- Yen HC, Lee S, Tanksley SD, et al. The tomato Never-ripe locus regulates ethylene-inducible gene expression and is linked to a homolog of the Arabidopsis ETR1 gene. Plant Physiol. 1995;107:1343–1353.
- Gao L, Zhao WH, Qu HO, et al. The yellow-fruited tomato 1 (yft1) mutant has altered fruit carotenoid accumulation and reduced ethylene production as a result of a genetic lesion in ETHYLENE INSENSITIVE2. Theor Appl Genet. 2016;129:717–728.
- Ito Y, Nishizawa-Yokoi A, Endo M, et al. Re-evaluation of the rin mutation and the role of RIN in the induction of tomato ripening. Nat Plants. 2017;3:866–874.
- Vrebalov J, Ruezinsky D, Padmanabhan V, et al. A MADS-Box gene necessary for fruit ripening at the tomato ripening-inhibitor (rin) locus. Science. 2002;296:343–346.
- Manning K, Tör M, Poole M, et al. A naturally occurring epigenetic mutation in a gene encoding an SBP-box transcription factor inhibits tomato fruit ripening. Nat Genet. 2006;38:948–952.
- Zhu M, Chen G, Zhou S, et al. A new tomato NAC (NAM/ATAF1/2/CUC2) transcription factor, SlNAC4, functions as a positive regulator of fruit ripening and carotenoid accumulation. Plant Cell Physiol. 2014;55:119–135.
- Tao NG, Xu J, Cheng YJ, et al. Lycopene-ε-cyclase pre-mRNA is alternatively spliced in Cara Cara navel orange (Citrus sinensis Osbeck). Biotechnol Lett. 2005;27:779–782.
- Howitt CA, Cavanagh CR, Bowerman AF, et al. Alternative splicing, activation of cryptic exons and amino acid substitutions in carotenoid biosynthetic genes are associated with lutein accumulation in wheat endosperm. Funct Integr Genomics. 2009;9:363–376.
- Álvarez D, Voß B, Maass D, et al. Carotenogenesis is regulated by 5ʹUTR-mediated translation of phytoene synthase splice variants. Plant Physiol. 2016;172:2314–2326.
- Sun Y, Xiao H. Identification of alternative splicing events by RNA sequencing in early growth tomato fruits. BMC Genomics. 2015;16:948.
- Lerosen AL, Went FW, Zechmeister L. Relation between genes and carotenoids of the tomato. Proc Natl Acad Sci USA. 1941;27:236–242.
- Cheng X, Zhang D, Cheng Z, et al. A new family of Ty1-copia-like retrotransposons originated in the tomato genome by a recent horizontal transfer event. Genetics. 2009;181:1183–1193.
- Fray RG, Grierson D. Identification and genetic analysis of normal and mutant phytoene synthase genes of tomato by sequencing, complementation and co-suppression. Plant Mol Biol. 1993;22:589–602.
- Gady ALF, Vriezen WH, Van de Wal MHBJ, et al. Induced point mutations in the phytoene synthase 1 gene cause differences in carotenoid content during tomato fruit ripening. Mol Breed. 2012;29:801–812.
- Kang B, Gu Q, Tian P, et al. A chimeric transcript containing Psy1 and a potential mRNA is associated with yellow flesh color in tomato accession PI 114490. Planta. 2014;240:1011–1021.
- Yuan DJ, Chen J, Shen HL, et al. Genetics of flesh color and nucleotide sequence analysis of phytoene synthase gene 1 in a yellow-fruited tomato accession PI114490. Sci Hortic. 2008;118:20–24.
- Wright MW. A short guide to long non-coding RNA gene nomenclature. Hum Genomics. 2014;8:7.
- Lin T, Zhu GT, Zhang JH, et al. Genomic analyses provide insights into the history of tomato breeding. Nat Genet. 2014;46:1220–1226.
- Fray RG, Wallace A, Fraser PD, et al. Constitutive expression of a fruit phytoene synthase gene in transgenic tomatoes causes dwarfism by redirecting metabolites from the gibberellin pathway. Plant J. 1995;8:693–701.
- Fleming HK, Myers CE. Tomato inheritance, with special reference to skin and flesh colour in the orange variety. Proc Am Soc Hortic Sci. 1937;35:609–624.
- Kumar-Sinha C, Kalyana-Sundaram S, Chinnaiyan AM. SLC45A3-ELK4 chimera in prostate cancer: spotlight on cis-splicing. Cancer Discov. 2012;2:582–585.
- Li H, Wang J, Mor G, et al. A neoplastic gene fusion mimics trans-splicing of RNAs in normal human cells. Science. 2008;321:1357–1361.
- Shumskaya M, Bradbury LMT, Monaco RR, et al. Plastid localization of the key carotenoid enzyme phytoene synthase is altered by isozyme, allelic variation, and activity. Plant Cell. 2012;24:3725–3741.
- Gingeras TR. Implications of chimaeric non-co-linear transcripts. Nature. 2009;461:206–211.
- Nelson C, Ambros V. Trans-splicing of the C. elegans let-7 primary transcript developmentally regulates let-7 microRNA biogenesis and let-7 family microRNA activity. Development. 2019;146:dev172031.
- Yu CY, Kuo HC. The trans-spliced long noncoding RNA tsRMST impedes human embryonic stem cell differentiation through WNT5A-mediated inhibition of the epithelial-to-mesenchymal transition. Stem Cells. 2016;34:2052–2062.
- Labrador M, Mongelard F, Plata-Rengifo P, et al. Molecular biology-protein encoding by both DNA strands. Nature. 2001;409:1000.
- Robertson HM, Navik JA, Walden KKO, et al. The bursicon gene in mosquitoes: an unusual example of mRNA trans-splicing. Genetics. 2007;176:1351–1353.
- Fischer SEJ, Butler MD, Pan Q, et al. Trans-splicing in C. elegans generates the negative RNAi regulator ERI-6/7. Nature. 2008;455:491–496.
- Horiuchi T, Giniger E, Aigaki T. Alternative trans-splicing of constant and variable exons of a Drosophila axon guidance gene, lola. Genes Dev. 2003;17:2496–2501.
- Karimi M, Depicker A, Hilson P. Recombinational cloning with plant gateway vectors. Plant Physiol. 2007;145:1144–1154.
- Fillatti JJ, Kiser J, Rose R, et al. Efficient transfer of a glyphosate tolerance gene into tomato using a binary Agrobacterium tumefaciens vector. Bio/Technology. 1987;5:726–730.
- Yang JJ, Wang YY, Shen HL, et al. In silico identification and experimental validation of insertion-deletion polymorphisms in tomato genome. DNA Res. 2014;21:429–438.
- Su T, Xu Q, Zhang FC, et al. WRKY42 modulates phosphate homeostasis through regulating phosphate translocation and acquisition in Arabidopsis. Plant Physiol. 2015;167:1579–1591.
- Yoo SD, Cho YH, Sheen J. Arabidopsis mesophyll protoplasts: a versatile cell system for transient gene expression analysis. Nat Protoc. 2007;2:1565–1572.
- Xing HL, Dong L, Wang ZP, et al. A CRISPR/Cas9 toolkit for multiplex genome editing in plants. BMC Plant Biol. 2014;14:327.
- Zhang Z, Shrestha J, Tateda C, et al. Salicylic acid signaling controls the maturation and localization of the Arabidopsis defense protein ACCELERATED CELL DEATH6. Mol Plant. 2014;7:1365–1383.