ABSTRACT
The presence or absence of cytogenetic mutations is proposed to be responsible for the pathogenesis of acute myeloid leukaemia (AML). However, the current classification system is inadequate to elucidate the molecular heterogeneity of the disease, and therapy failures frequently occur. Leukaemia stem cells (LSCs) initiate and maintain the clonal hierarchy of AML and exhibit properties of self-renewal remaining recalcitrant to conventional chemotherapy. In this study, we identified a novel long non-coding RNA (lncRNA) MAGI2 antisense RNA 3 (MAGI2-AS3) in AML and investigated its functional role in regulating LSCs self-renewal. LSCs were identified by immunoprofiling of CD34+ CD123+ in AML patients’ marrow. MAGI2-AS3 exhibited a poor expression level in LSCs than the normal human haematopoietic stem cells. Lentivirus-mediated upregulation of MAGI2-AS3 or leucine-rich repeats and Ig-like domains 1 (LRIG1) impaired LSCs self-renewal. MAGI2-AS3-overexpressed LSCs acquired the ability of self-renewal following lentivirus-mediated knockdown of LRIG1. Methylation-dependent inhibition of LRIG1 was evident in LSCs. MAGI2-AS3 was found to induce occupancy of TET2 at the LRIG1 promoter. Lentivirus-mediated downregulation of TET2 could impair MAGI2-AS3-mediated elevation of LRIG1 and neutralize the inhibitory effect of MAGI2-AS3 on LSCs self-renewal. In vivo analysis indicated an elevated overall survival of NOD/SCID mice injected with LSCs in the presence of MAGI2-AS3. Altogether, the key findings support the potential of lncRNA MAGI2-AS3 to serve as a novel candidate for the improvement of AML treatment.
Introduction
Acute myeloid leukaemia (AML) is a heterogeneous malignancy with great heterogeneity in biology, phenotype and prognostic behaviour with persistent disease relapse [Citation1]. The treatment protocols and outcome for patients with AML are primarily age-dependent due to their tolerance of effective therapy [Citation2]. Although allogeneic stem cell transplantation has substantially reduced morbidity and mortality, AML still accounts for mortality in more than half of young adult patients and about 90% of older patients [Citation3]. Interestingly, AML originates from a hierarchy of leukaemic stem cells (LSCs) classes that illustrate different self-renewal capacities [Citation4], and the gene expression signatures in LSCs are associated with therapy failure [Citation5].
Recently, research has flagged the association of long noncoding RNA (lncRNA) expressions with recurrent mutations in AML and thus predictive of treatment response and survival [Citation6,Citation7]. For instance, overexpression of CASC15 contributed to a myeloid bias in progression and decreased colony formation [Citation8]. Moreover, lncRNA H22954 was downregulated in AML patients and inhibited cancer growth in a Bcl-2-dependent mechanism [Citation9]. MAGI2 antisense RNA 3 (MAGI2-AS3) is a newly identified lncRNA, and its functional role by regulating the Fas/FasL signalling pathway was studied in breast cancer [Citation10]. In addition, an analysis of the GSE17054 dataset profiled 3,005 differentially expressed genes between normal human haematopoietic stem cells and LSCs originating from patients with AML, and identified lncRNA MAGI2-AS3 as one of the down-regulated genes. In addition to the lncRNA, reports have demonstrated the vitality of epigenetic and genetic heterogeneity, such as DNA methylation signatures, to underlie various biological subtypes in AML [Citation11,Citation12]. For instance, Ten-Eleven Translocation-2 (TET2) demonstrates functionality as a DNA demethylase that can convert 5-methyl-cytosine to 5-hydroxymethyl-cytosine. An existing study documented the presence of TET2 missense or nonsense mutations in 53% of patients with chronic myelomonocytic leukaemia, and essentially, TET2 mutations increased global methylation in chronic myelomonocytic leukaemia [Citation13]. Similar to TET2, leucine-rich repeats and immunoglobulin-like domains-1 (LRIG1) also play a role in haematopoiesis and leukaemogenesis. LRIG1 was found to be significantly downregulated in chronic lymphocytic leukaemia [Citation14]. Moreover, LRIG1, essentially reduced in AML, has been shown to sustain stem cell quiescence [Citation15]. However, whether LRIG1 downregulation leads to human tumorigenesis and MAGI2-AS3 and whether DNA demethylase TET2 regulate the LRIG1 expression remain elusive.
In the present study, we observed MAGI2-AS3 and LRIG1 to be expressed at low levels in LSCs. Lentivirus-mediated overexpression of MAGI2-AS3 or LRIG1 inhibited LSCs self-renewal, while LRIG1 knockdown resulted in the enhancement of the self-renewal ability in MAGI2-AS3-overexpressing LSCs. Interestingly, methylation-dependent repression of LRIG1 was demonstrated in LSCs. Mechanistically, MAGI2-AS3 was found to facilitate the occupancy of TET2 in LRIG1 promoter. Besides, TET2 knockdown could impair MAGI2-AS3-mediated enhancement of LRIG1 and reduce the inhibitory effect of MAGI2-AS3 on LSCs self-renewal. The findings provided an insight into the novel regulatory network of MAGI2-AS3/TET2/LRIG1 in LSCs in AML.
Results
Downregulation of lncRNA MAGI2-AS3 in LSCs
We downloaded the gene expression profile GSE17054, which consisted of 4 normal samples and 9 AML samples with normal samples as control [Citation16] from the Gene Expression Omnibus database (http://www.ncbi.nlm.nih.gov/geo). Analysis of the differentially expressed lncRNAs was conducted using the ‘limma’ package of R language [Citation17] with the screening criteria set as |log FoldChange| >1 and a p value <0.05. Our analysis of the dataset revealed the presence of a total of 3005 differentially expressed lncRNAs in LSCs of AML, among which lncRNA MAGI2-AS3 was significantly down-regulated (). To elucidate MAGI2-AS3 function in LSCs, we first tested its expression by isolating CD34+ CD123+ LSCs (), followed by RT-qPCR. In accordance with the analysis results of the GSE17054 database, RT-PCR result revealed that expression of MAGI2-AS3 in LSCs was significantly lower in CD34+ CD123+ LSCs compared to the normal human haematopoietic stem cells (), suggesting that lncRNA MAGI2-AS3 is down-regulated in LSCs.
Figure 1. LncRNA MAGI2-AS3 is down-regulated in LSCs. A: Expression of MAGI2-AS3 in the GSE17054 dataset, n = 13; B: Isolation of LSCs (CD34+ CD123+ cells); C: Expression of MAGI2-AS3 in LSCs derived from AML patients (AMLSC group) and normal human haematopoietic stem cells (normal group) determined by RT-qPCR (normal: n = 12; AMLSC: n = 41). Data were expressed as mean ± standard error and analysed by unpaired t-test; * p < 0.05 vs. normal human haematopoietic stem cells.
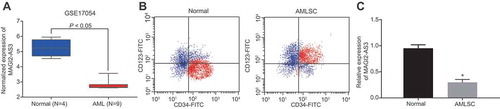
LncRNA MAGI2-AS3 inhibits the LSCs self-renewal
Given the downregulated level of MAGI2-AS3 in LSCs, we hypothesize a potential role of the lower level of MAGI2-AS3 in respect to LSCs self-renewal. To explore whether overexpression of lncRNA MAGI2-AS3 inhibits the cell properties of LSCs, we conducted sphere formation assay and colony formation assay with LSCs over-expressing MAGI2-AS3. The elevated level of MAGI2-AS3 was confirmed () by performing RT-qPCR. As depicted in and C, MAGI2-AS3 upregulation significantly reduced the sphere formation and colony formation abilities of LSCs. The results strongly support the conclusion that an increased level of lncRNA MAGI2-AS3 suppresses LSCs self-renewal.
Figure 2. LncRNA MAGI2-AS3 inhibits the LSCs self-renewal. LSCs were treated with either oe-MAGI2-AS3 or oe-NC. A: Expression of MAGI2-AS3 in LSCs determined by RT-qPCR; B: Sphere-forming ability of LSCs via sphere formation assay (× 200); C: Colony formation capability of LSCs via colony formation assay. Data were expressed as mean ± standard error and analysed by unpaired t-test. * p < 0.05 vs. the oe-NC group.
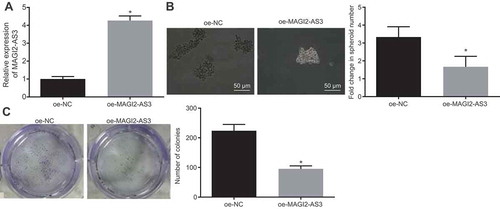
LncRNA MAGI2-AS3 inhibits the LSCs self-renewal by promoting LRIG1 expression
Our data clearly illustrated that lncRNA MAGI2-AS3 overexpression inhibited LSCs self-renewal; however, the mechanism underlying remained elusive. Interestingly, our analysis suggested a positive correlation between MAGI2-AS3 and LRIG1 (). Moreover, LRIG1 was reported to be down-regulated in AML [Citation14], similar to MAGI2-AS3. Therefore, we hypothesized that MAGI2-AS3 might inhibit LSCs self-renewal and AML progression by up-regulating LRIG1 expression. LRIG1 expression was tested by RT-qPCR and western blot analysis, and we found that LRIG1 was down-regulated in LSCs ( and ). In addition, a MAGI2-AS3 overexpression resulted in an increase in LRIG1 expression ( and ). Altogether, LRIG1 was down-regulated in LSCs, and MAGI2-AS3 overexpression promoted the LRIG1 expression.
Figure 3. LncRNA MAGI2-AS3 inhibits LSCs self-renewal by elevating LRIG1 expression. A: Analysis of the GSE17054 dataset revealed the positive correlation between MAGI2-AS3 and LRIG1; B: LRIG1 mRNA expression in LSCs and normal human haematopoietic stem cells determined by RT-qPCR; C: LRIG1 protein expression in LSCs and normal human haematopoietic stem cells determined by western blot analysis; D: LRIG1 mRNA expression in LSCs with MAGI2-AS3 over-expression revealed by RT-qPCR; E: LRIG1 protein expression in LSCs with MAGI2-AS3 over-expression shown by western blot analysis; F: LRIG1 protein expression in LSCs following LRIG1 over-expression shown by western blot analysis; G: Sphere-forming ability of LSCs examined by sphere formation assay (× 200); H: Colony formation ability of LSCs using colony formation assay; I: LRIG1 mRNA expression in cells with oe-MAGI2-AS3 and sh-LRIG1 determined by RT-qPCR; J: LRIG1 protein expression in cells with oe-MAGI2-AS3 and sh-LRIG1 detected by western blot analysis; K: Sphere-forming ability of LSCs using sphere formation assay (× 200); L: Colony formation ability of LSCs via colony formation assay; * p < 0.05 vs. normal human haematopoietic stem cells; # p < 0.05 vs. the oe-NC group; & p < 0.05 vs. the oe-MAGI2-AS3 + sh-NC group. Data were expressed as mean ± standard error and analysed using unpaired t-test.
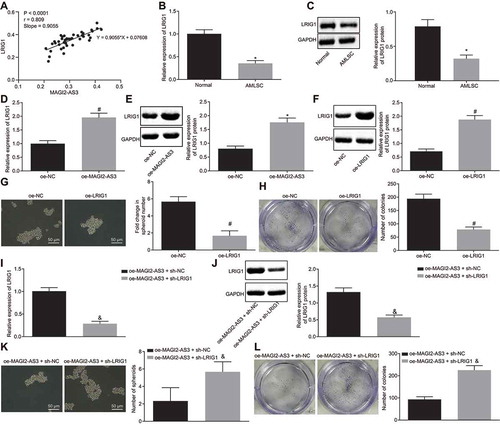
To further elucidate the influence of LRIG1 on LSCs self-renewal, LRIG1 was overexpressed (), followed by the sphere formation and colony formation assays, which illustrated significantly reduced ability to form sphere and colony ( and ). The results demonstrate that similar to MAGI2-AS3, overexpression of LRIG1 can also inhibit LSCs self-renewal.
To explore whether LRIG1 was a key target during the inhibition of LSCs self-renewal by MAGI2-AS3, we down-regulated LRIG1 in LSCs overexpressing MAGI2-AS3 (oe-MAGI2-AS3 + sh-LRIG1) and compared its self-renewal ability with the control cells (oe-MAGI2-AS3 + sh-NC). The reduced level of LRIG1 was tested by RT-qPCR and western blot analysis ( and ). The following sphere formation and colony formation assays revealed that LSCs with oe-MAGI2-AS3 and sh-LRIG1 had promoted abilities of sphere and colony formation than cells treated with oe-MAGI2-AS3 and sh-NC ( and L). In summary, the results demonstrate that lncRNA MAGI2-AS3 could hinder the self-renewal of LSCs by promoting LRIG1 expression.
LRIG1 promoter region is methylated in AML
To further investigate how lncRNA MAGI2-AS3 regulated LRIG1 expression, we identified two CpG sites in the promoter region of LRIG1 using MethPrimer (http://www.urogene.org/cgi-bin/methprimer/methprimer.cgi) (). The status of methylation in the promoter of LRIG1 was analysed by MSP, which demonstrated that promoter methylation occurred in LSCs, but not in normal human haematopoietic stem cells (). We speculated that the promoter of LRIG1 was methylated and this methylation inhibited LRIG1 expression. Consistent with this hypothesis, the methylation level of LRIG1 promoter region was significantly reduced (), while the LRIG1 expression was notably enhanced ( and E) in LSCs treated with the methyltransferase inhibitor 5-Aza-dC. Together, the LRIG1 promoter region can be methylated and this methylation inhibits the LRIG1 expression.
Figure 4. LRIG1 promoter region was methylated and expressed at low levels in AML. A: CpG sites in the promoter region of LRIG1; B: Methylation level of LRIG1 promoter in LSCs and normal human haematopoietic stem cells determined by MS-PCR; C: Methylation level of LRIG1 promoter in LSCs treated with 5-Aza-dC determined by MS-PCR; D: LRIG1 mRNA expression in LSCs treated with 5-Aza-dC determined by RT-qPCR; E: The LRIG1 protein expression in LSCs treated with 5-Aza-dC detected by western blot analysis; *, p < 0.05 vs. the DMSO group; data were expressed as mean ± standard error and analysed using unpaired t-test. The experiment was repeated three times independently.
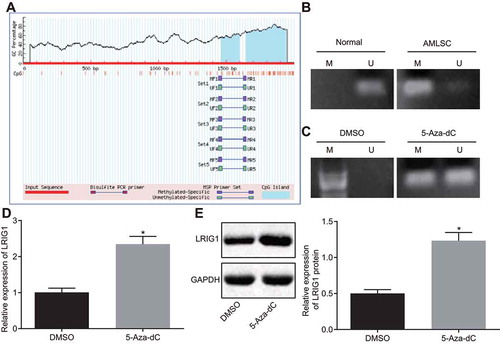
LncRNA MAGI2-AS3 recruits TET2 to the LRIG1 promoter region and causes DNA demethylation of LRIG1
To further understand the underlying mechanism on how lncRNA MAGI2-AS3 might regulate the methylation level of LRIG1 promoter, we characterized MAGI2-AS3 localization in cells. We found MAGI2-AS3 to be predominantly localized in the nucleus through lncATLAS analysis (http://lncatlas.crg.eu/) (), which was further verified by FISH (). Fractionation of the nuclear and cytoplasmic RNA displayed that the localization of MAGI2-AS3 in the nucleus strongly inferred to its ability to potentially regulate LRIG1 expression at the transcriptional level (). Interestingly, DNA demethylase TET2 was observed as a binding partner of MAGI2-AS3 in the RPIseq databank (http://pridb.gdcb.iastate.edu/RPISeq/) (). In addition, an analysis of the LongTarget website (http://lncrna.smu.edu.cn/show/DNATriplex) also revealed that MAGI2-AS3 might combine with LRIG1 promoter in the form of a RNA-DNA hybrid (). With the aforementioned evidence, we speculated that MAGI2-AS3 might activate LRIG1 expression by recruiting TET2 and then consequently demethylating LRIG1 DNA. To ascertain this speculation, we conducted RNA pull-down and RIP assays to detect the degree of TET2 recruitment by MAGI2-AS3 using LSCs with MAGI2-AS3 overexpression. The ability of MAGI2-AS3 to pull-down TET2 was corroborated by RNA pull-down assay (), and this binding was significantly enhanced in RIP assay, suggesting that MAGI2-AS3 can recruit TET2 and therefore more TET2 is recruited in MAGI2-AS3 overexpressed LSCs ().
Figure 5. LncRNA MAGI2-AS3 promotes DNA demethylation of LRIG1 by recruiting TET2. A: lncATLAS identifies the cellular localization of MAGI2-AS3 in nucleus; B: The cellular localization of MAGI2-AS3 is verified using FISH; C: The cellular localization of MAGI2-AS3 in nucleus is confirmed using fractionation of nuclear and cytoplasmic RNA; D: RPIseq databank predicts DNA demethylase TET2 are relevant to MAGI2-AS3 using RF classifier and SVM classifier (RF > 0.5 & SVM > 0.5); E: potential binding sites for MAGI2-AS3 within LRIG1 promoter region predicted by LongTarget; F: RNA pull-down shows TET2 binds to MAGI2-AS3 using western blot analysis; G: binding of MAGI2-AS3 to TET2 in LSCs with MAGI2-AS3 overexpression in RIP assay followed by RT-qPCR; H: enrichment of TET2 in LRIG1 promoter region revealed by ChIP-qPCR; * p < 0.05 vs. the oe-NC group; data were expressed as mean ± standard error and analysed using unpaired t-test. The experiment was repeated three times independently.
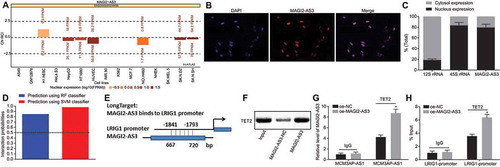
Next, we adopted ChIP to detect the enrichment of TET2 in the LRIG1 promoter, and this enrichment further increased with MAGI2-AS3 overexpression (). More importantly, the demethylation level of LRIG1 DNA increased after the recruitment of TET2.
LncRNA MAGI2-AS3 inhibits the LSCs self-renewal by promoting TET2-dependent DNA demethylation of the LRIG1 promoter
To examine whether TET2 recruitment is critical for the inhibition of MAGI2-AS3 on LSCs self-renewal by upregulation of LRIG1, we overexpressed MAGI2-AS3 and knocked down TET2 in LSCs. The western blot analysis showed that the TET2 protein level was diminished in cells treated with oe-MAGI2-AS3 and sh-TET2 (). In addition, the LRIG1 expression level reduced significantly ( and ) in cells treated with oe-MAGI2-AS3 and sh-TET2. These results suggest that MAGI2-AS3 mediates LRIG1 demethylation and promotes LRIG1 expression via recruiting TET2.
Figure 6. MAGI2-AS3 inhibits the LSCs self-renewal by promoting LRIG1 DNA demethylation via TET2 recruitment. A: protein expression of TET2 is determined by western blot analysis; B: mRNA expression of LRIG1 is tested by RT-qPCR; C: protein expression LRIG1 is determined by western blot analysis D: LSCs sphere formation using sphere formation assay (× 200); E: LSCs colony formation using colony formation assay; * p < 0.05 vs. the oe-MAGI2-AS3 + sh-NC group; data were expressed as mean ± standard error and analysed using unpaired t-test. The experiment was repeated three times independently.
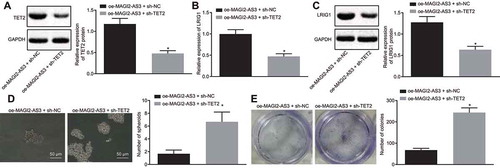
Furthermore, the self-renewal of LSCs was tested by adopting the sphere formation and colony formation assays. Results revealed that the TET2 knockdown in MAGI2-AS3 overexpressing cells led to enhanced sphere and colony formation ( and ). These results illustrate that TET2 recruitment in MAGI2-AS3-overexpressing cells is critical for LSCs self-renewal.
MAGI2-AS3 inhibits the LSCs self-renewal and increases survival rate of NOD/SCID mice with AML in vivo
The AML mouse model was established in NOD/SCID mice by an intravenous injection with LSCs into the caudal vein to explore how lncRNA MAGI2-AS3 influences AML in vivo. We conducted side population analysis to detect the LSCs content in peripheral blood and found that LSCs content reduced progressively following MAGI2-AS3 overexpression (). Survival rates of NOD/SCID mice with AML increased remarkably following MAGI2-AS3 overexpression (). In summary, MAGI2-AS3 could potentially inhibit the self-renewal of LSCs and increase the survival rate of AML mouse models.
Figure 7. MAGI2-AS3 inhibits self-renewal of LSCs and increases the survival rate of NOD/SCID mice with AML. A: side population analysis of LSCs content in peripheral blood using flow cytometry; B: quantitative analysis for the survival rates of NOD/SCID mice with AML; data were expressed as mean ± standard error and analysed using ANOVA of repeated measurements to examine the statistics from different groups at different time points, followed by Bonferroni post hoc test. n = 25, *, p < 0.05 vs. the oe-NC group.
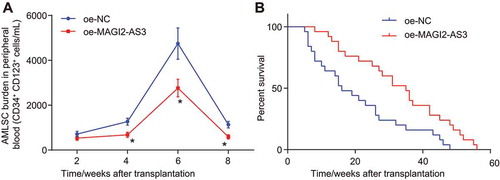
Discussion
LSCs have exhibited extensive self-renewing potentials and are vital for the progression of AML [Citation18,Citation19]. The potential of therapeutic modalities to eliminate the subgroup of LSCs in AML patients is clear and definite. Moreover, inactivation of the Wnt/β-catenin pathway has been suggested to impair LSCs development in AML [Citation20,Citation21]. However, the specific mechanism underlying LSCs maintenance and self-renewal remains elusive.
Accumulating evidence has proposed the modulatory roles of lncRNAs on cancer stem cell self-renewal and tumour propagation [Citation22,Citation23]. The study by Trimarchi et al. has examined the role of lncRNAs in T cell leukaemia and identified the potential of lncRNAs to function as biomarkers and therapeutic targets in AML as well as key regulators of cellular homoeostasis and transformation intrinsically contributing to tumorigenesis [Citation24]. A recent study acknowledged the functionality of MAGI2-AS3 as a suppressor of breast cancer [Citation10]. Another study has illustrated the capability of lncRNA MAGI2-AS3 to inhibit cell proliferation in hepatocellular carcinoma [Citation25]. With the aforementioned evidence, we hypothesized that lncRNA MAGI2-AS3 might be involved in LSCs self-renewal. In this study, the results of a series of experiments revealed that lncRNA MAGI2-AS3 overexpression can suppress LSCs self-renewal through in vitro and in vivo experimentation.
For a better understanding of the underlying MAGI2-AS3 regulation of LSCs self-renewal, we initially analysed the publicly available GSE17054 dataset and further analysed the correlation between MAGI2-AS3 and LRIG1. LRIG1, a transmembrane protein, has shown an interaction with the receptor tyrosine kinases of the EGFR-family MET and RET. Expression of LRIG1 is suggested to be involved in epidermal homoeostasis and downregulation of LRIG1 in cultured human keratinocytes resulted in enhanced proliferation and stemness. In the context of malignancy, LRIG1 has not only demonstrated functionality as a tumour suppressor in the intestinal stem cells but also as an independent positive prognostic marker in oropharyngeal cancer [Citation26,Citation27]. The current study identified two CpG sites in the LRIG1 promoter region using MethPrimer and inferred the prospect of methylation of the LRIG1 promoter in LSCs. In accordance with our finding, an existing study has also demonstrated the involvement of LRIG1 in association with increased promoter region hyper-methylation and LRIG1 methylation in colorectal cancer cells [Citation28]. Our experiments further elucidated that an elevated expression of LRIG1 by MAGI2-AS3 overexpression impairs LSCs self-renewal.
We further identified the DNA demethylase TET2, which is enlisted by MAGI2-AS3 to the LRIG1 promoter and contributes to DNA demethylation. The TET family member TET2 dioxygenase is highly conserved, with critical functionality in the regulation of DNA-methylation status by oxidizing 5-methylcytosine (5mC) to generate 5-hydroxymethylcytosine (5hmC). Somatic mutations of TET2 developed in about 15% patients with various myeloid cancers, suggesting the role of TET2 as a modulator in the control of the balance between survival, growth, and differentiation in normal haematopoiesis [Citation29]. Meanwhile, studies have observed that DNA methylation at non-CpG islands regions was affected by leukaemia-associated TET2 mutations [Citation30]. Consistently, a recent research found that the TET2 mutations could essentially administer DNA methylation dynamics in AML patients [Citation31]. Additionally, Kenji Ichiyama and his group further demonstrated the functionality of TET2-regulated active DNA demethylation in helper T cells [Citation32]. In the present study, the RPIseq databank demonstrated MAGI2-AS3 to be significant to TET2, and our results showed that MAGI2-AS3 recruited TET2 due to its ability to co-precipitate with TET2. We also observed that MAGI2-AS3 could bind to the LRIG1 promoter. Collectively, we hypothesized that lncRNA MAGI2-AS3 might facilitate TET2-dependent DNA demethylation of the LRIG1 promoter. Indeed, MAGI2-AS3 overexpression can lead to enhanced enrichment of TET2 in LRIG1 promoter, and consequently triggers LRIG1 demethylation.
To conclude, the current study provides new insights into the mechanism behind the regulation network of lncRNA MAGI2-AS3 in the control of LSCs self-renewal (). Specifically, lncRNA MAGI2-AS3, which is downregulated in LSCs, suppressed the LSCs self-renewal via binding to LRIG1 and by recruiting DNA demethylase TET2 to the LRIG1 promoter. In addition, the TET2-dependent DNA demethylation of the LRIG1 promoter is essential for the inhibition of LSCs and can serve as a prospective therapeutic target for AML in the future. More importantly, our study paved the way for future studies to identify the detailed binding form mediating the interaction between lncRNA MAGI2-AS3 and LRIG1.
Materials and methods
Ethics statement
The study was conducted with approval of the Ethics Committee of Tongji Medical College, Huazhong University of Science and Technology (IORG No: IORG0003571) and followed the tenets of the Declaration of Helsinki. All participants provided written informed consent prior to enrolment. Animal experiments were conducted in strict accordance with the Institutional Guidelines under permission from the Animal Ethics Committee of Tongji Medical College, Huazhong University of Science and Technology and in compliance with the Guide for the Care and Use of Laboratory animals published by the US National Institutes of Health.
Samples
Bone marrow samples were collected from 12 healthy volunteers and 41 AML patients with the French-American-British (FAB) subtypes [Citation33] of M1 (3 cases), M2 (22 cases), M4 (10 cases), M5 (6 cases). The patients were clinically diagnosed and treated at the Institute of Haematology in Union Hospital, Tongji Medical College, Huazhong University of Science and Technology from June 2016 to December 2018, including 26 males and 15 females with a mean age of 42 years (ranging from 18 to 72 years).
Isolation and sorting of leukaemia stem cells (LSCs)
Bone marrow (4 mL) was extracted and then mixed with 4 mL Ficoll-Paque PLUS (17-1440-03, Shanghai Yes Service Biotech, Inc., Shanghai, China), followed by centrifugation for 10 min at 2000 × g. Peripheral blood mononuclear cells (PBMCs) were suspended at a concentration of 1 × 107 cells/mL. Antibodies against fluorescein isothiocyanate-conjugated CD34 (1:50, ab8536, Abcam, Cambridge, UK) and polyethyleneglycol-conjugated CD123− (1:500, ab21562, Abcam, Cambridge, UK) were added into the cell suspension to sort and isolate CD34+CD123+ LSCs cells that are defined as LSCs [Citation34]. Meanwhile, CD34+CD123− LSCs cells were also harvested as normal human haematopoietic stem cells.
Fluorescence in situ hybridization (FISH) assay
The subcellular localization of MAGI2-AS3 in LSCs was identified by FISH in strict accordance with the instructions of the RiboTM lncRNA FISH Probe Mix (Red) (RiboBio Co., Ltd., Guangzhou, Guangdong, China). Concisely, the cover glass was subjected in a six-well plate, and the LSCs were seeded for 1 d until cells were covered with 80% microscopic view. The cover glass was fixed using 1 mL 4% polyformaldehyde. After treatment with a combination of protease K (2 μg/mL), glycine, and acetylation reagents, the cells were pre-hybridized using 250 μL pre-hybridization solution at 42°C for 1 h and hybridized using 250 μL hybridization solution containing 300 ng/mL probe at 42°C overnight. Next, PBS/Tween-20 diluted 4ʹ,6-Diamidino-2-Phenylindole (1:800) was added to the cells for staining the nucleus for 5 min. Then, the cells were sealed using anti-fluorescence quencher. Subsequently, the cells were observed and photographed under a fluorescence microscope (Olympus, Tokyo, Japan) from five different fields.
Cell treatment
We developed MAGI2-AS3- or LRIG1-overexpressed LSCs using pCMV6-AC-GFP models and LRIG1- or TET2-depleted LSCs using pGPU6/Neo-LRIG1 shRNA-expressing pDNA (LRIG1 shRNA) or TET2 shRNA. The models of pCMV6-AC-GFP and pGPU6/Neo were purchased from Wuhan Miaoling Biotechnology (Wuhan, Hubei, China) and GenePharma Co., Ltd. (Shanghai, China) respectively. Transfection with Lipofectamine 2000 (11,668,019, Thermo Fisher Scientific Inc., San Jose, CA, USA) was performed in strict accordance with the provided protocol. Methyltransferase inhibitor 5-aza-2ʹ-deoxycytidine (5-aza-dC) was added to the LSCs for reducing the methylation.
RNA extraction and quantification
Total RNA was isolated using Trizol (Invitrogen, Carlsbad, CA, USA) in strict accordance with the provided instruction. All RNA samples were quantified using a nanodrop2000 ultraviolet spectrophotometer (1011U, NanoDrop Technologies, Wilmington, DE, USA). The cDNA was reversely transcribed with a PrimeScript RT reagent Kit (RR047A, Takara Bio, Shiga, Japan). Primers of lncRNA MAGI2-AS3 and LRIG1 were designed and synthesized by the Takara Bio Company (Shiga, Japan) (). Reverse-transcription quantitative polymerase chain reaction (RT-qPCR) was then conducted using the ABI7500 Real-Time PCR System (7500, Applied Biosystems, Inc., Foster City, CA, USA) with glyceraldehyde phosphate dehydrogenase (GAPDH) as its normalization. Analysis and fold change were determined based on the relative quantification (2−ΔΔCt method) [Citation35].
Table 1. Primer sequences for RT-qPCR.
Western blot analysis
The cells were lysed using radioimmunoprecipitation (RIPA) assay lysis buffer containing phenylmethyl sulfonylfluoride (R00013, Beyotime Biotechnology, Shanghai, China). The proteins were separated by conducting sodium dodecyl sulphate-polyacrylamide gel electrophoresis and then transferred onto polyvinylidene fluoride membranes. Membrane blockade was conducted using 5% skim milk for 1 h followed by incubation with the primary antibody, rabbit antibodies against CD34 (1:10,000, ab81289, Abcam, Cambridge, UK), CD123 (1:1500, ab200687, Abcam, Cambridge, UK), LRIG1 (1:1000, #12,752, Cell Signalling Technology, Beverly, MA, USA) and TET2 (1:2000, ab94580, Abcam, Cambridge, UK) with GAPDH (ab9485, 1:2500, Abcam, Cambridge, UK) as normalization. The membranes were then incubated with the goat anti-rabbit antibody IgG (ab97051, 1:2000, Abcam, Cambridge, UK) labelled using horseradish peroxidase for 1 h. Finally, the enhanced chemiluminescence reagent kit (BB-3501, Amersham Pharmacia Biotech, Little Chalfont, UK) was utilized to visualize the protein bands under a Bio-Rad image analysis system (Bio-Rad, Hercules, CA, USA). The relative protein expression was quantified as the ratio of the grey value of the corresponding protein band to that of the grey value of GAPDH.
Sphere formation and colony formation assay
Transfected LSCs were suspended at a density of 1 × 104 cells/mL in a 24-well ultra-low-attachment plate. The formed spheres were observed under an inverted optical microscope (CKX4l, Olympus, Tokyo, Japan). The number of newly formed suspended cell spheres per well was documented. LSCs were plated using 0.7% agar in DMEM as the bottom layer of agar. A mixture of 1 mL suspension and 1 mL 0.7% agarose was prepared to culture 1 × 104 LSCs in every 100 cm2. After solidification of the upper layer of agar, 2–3 mL culture medium was added gradually over the surface of the agar and then incubated at 37°C with 5% CO2. The culture solution was replaced every 3 days. A month later, the colonies with more than 50 cells were counted under a microscope (Olympus, Tokyo, Japan) [Citation36].
Fractionation of nuclear and cytoplasmic RNA
Nuclear and cytoplasmic RNA fractions were isolated in strict accordance with the PARISTM Kit Protein and the RNA Isolation System (Life Technologies, Carlsbad, CA, USA). Briefly, LSCs were resuspended in 500 μL cell fractionation buffer followed by trypsinization and centrifugation. Nuclear fraction and cytoplasmic fraction were separated by centrifugation at 500 × g for 5 min at 4°C. Cytoplasmic fraction was harvested and subjected to repeated centrifugation. While the nuclear fraction was combined and reacted successively with 500 μL cell fractionation buffer, 500 μL 2× lysis/binding solution, 500 μL cell disruption buffer, 500 μL absolute ethyl alcohol, 700 μL reaction solution, followed by centrifugation. Afterwards, the nuclear fraction was, respectively, centrifuged using a combination of 700 μL wash solution I and 500 μL wash solution 2/3 at 12,000 × g for 30 s. Both mixtures were transferred to a filter cartridge. Nuclear and cytoplasmic RNA was harvested after elution and then determined by RT-qPCR, with 45S rRNA serving as the internal control for the nuclear RNA expression and 12S rRNA for determination of the cytoplasmic RNA-expression ().
Table 2. RT-qPCR primer sequences for fractionation of nuclear and cytoplasmic RNA.
Methylation-specific PCR (MS-PCR)
Genomic RNA was extracted using a Genomic RNA extraction kit (Beijing TianGen Biochemical Company, Beijing, China) and treated with sodium sulphite using an EZ DNA Methylation Kit (Zymo Research®, Orange, CA, USA) in strict accordance with the provided instructions. Methylated- and unmethylated-specific primers () amplified the modified DNA, followed by repeated amplification using the same fragment within the promoter of LRIG1. MSP products were analysed using agarose gel with an imaging system as aforementioned [Citation37].
Table 3. Primer sequences for methylation-specific PCR.
RNA pull-down
LncRNA MAGI2-AS3 was transcribed using the T7 RNA polymerase (Ambion, Austin, TX, USA), treated using the RNeasy Plus Mini kit (Qiagen, Hilden, Germany) and DNase I (Qiagen, Hilden, Germany), purified using a RNeasy Mini Kit (Qiagen, Hilden, Germany) and finally labelled with the Biotin RNA Labelling mix (Ambion, Austin, TX, USA). The biotinylated RNA (1 μg) was pretreated with the RNA structure buffer to facilitate the formation of a suitable secondary structure. LSCs were resuspended using the lysis buffer. The supernatant was collected and transferred to the RNase-free centrifuge tube. The biotinylated RNA (400 ng) was added to 500 μL RNA immunoprecipitation (RIP) buffer and mixed at room temperature with the cell lysate, followed by the addition of streptavidin beads. The beads were rinsed 5 times in 5 × RIP buffer. Western blot analysis was conducted to detect the concentration of eluted TET2 protein. In this experiment, IgG (ab172730, 1:100, Abcam, Cambridge, UK) was used as a negative control (NC).
RIP assay
RIP was performed using the RIP RNA-Binding Protein Immunoprecipitation Kit (Millipore Corp, Billerica, MA, USA) based on the provided instructions. This protocol was originally published in a study [Citation38]. RIPA lysis buffer (P0013B, Beyotime Biotechnology, Shanghai, China) was added to lyse the cells. Magnetic beads (50 μL) were extracted and resuspended using 100 μL RIP Wash Buffer. Magnetic beads-antibody complex was resuspended using 900 μL RIP Wash Buffer. The rabbit antibody against TET2 (1:100, ab94580, Abcam, Cambridge, UK) was added for RIP with IgG (1:100, ab172730, Abcam, Cambridge, UK) as NC. RT-qPCR was conducted to detect the co-precipitated RNA.
Chromatin immunoprecipitation (ChiP) assay
Chromatin immunoprecipitation was performed as aforementioned [Citation39]. After cross-linking of LSCs with the protein, the chromatin fragmentation was performed using sonication. Supernatants were collected after centrifugation at 12,000 × g at 4°C and then separated into two tubes, after which the rabbit antibodies against TET2 (1:100, ab230358, Abcam, Cambridge, UK) or IgG (1:100, ab172730, Abcam, Cambridge, UK) were added. Protein Agarose/Sepharose was added to precipitate the endogenous DNA-protein complex. The crosslinking was reversed at 65°C overnight. The DNA fragments were then collected, and the detection of LRIG1 was performed by qPCR with the LRIG1 gene promoter-specific primers [Citation40].
Animal experiment in vivo
NOD/SCID mice aged between 6–8 weeks (weighting 18–22 g) were purchased from Beijing Vital River Laboratory Animal Technology Co., Ltd. (Beijing, China). Mice were divided randomly between two groups (25 mice for each group) after which they underwent treatment with whole-body X-ray irradiation (1.8 Gy). Mice were injected intravenously with 5 × 106 LSCs in the logarithmic growth phase transfected with MAGI2-AS3 or oe-NC. Caudal vain blood was extracted from 4 mice to detect LSCs for its survival rates every 2 weeks.
Statistical analysis
All data were processed and analysed using the SPSS 21.0 statistical software (IBM Corp., Armonk, New York, USA). Data were expressed as mean ± standard deviation. Unpaired t test was adopted to analyse the differences between normally distributed values of two experimental groups. Two-way analysis of variance (ANOVA) was performed to examine the statistics from different mice at different time points, followed by Kaplan-Meier analysis to evaluate the survival rate of mice. A value of p < 0.05 was considered to be statistically significant.
Disclosure statement
No potential conflict of interest was reported by the authors.
Additional information
Funding
References
- Patel JP , Gonen M , Figueroa ME , et al. Prognostic relevance of integrated genetic profiling in acute myeloid leukemia. N Engl J Med. 2012;366:1079–1089.
- Burnett A , Wetzler M , Lowenberg B. Therapeutic advances in acute myeloid leukemia. J Clin Oncol. 2011;29:487–494.
- Ferrara F , Schiffer CA . Acute myeloid leukaemia in adults. Lancet. 2013;381:484–495.
- Hope KJ , Jin L , Dick JE . Acute myeloid leukemia originates from a hierarchy of leukemic stem cell classes that differ in self-renewal capacity. Nat Immunol. 2004;5:738–743.
- Shlush LI , Mitchell A , Heisler L , et al. Tracing the origins of relapse in acute myeloid leukaemia to stem cells. Nature. 2017;547:104–108.
- Garzon R , Volinia S , Papaioannou D , et al. Expression and prognostic impact of lncRNAs in acute myeloid leukemia. Proc Natl Acad Sci U S A. 2014;111:18679–18684.
- Cao L , Xiao PF , Tao YF , et al. Microarray profiling of bone marrow long non-coding RNA expression in Chinese pediatric acute myeloid leukemia patients. Oncol Rep. 2016;35:757–770.
- Fernando TR , Contreras JR , Zampini M , et al. The lncRNA CASC15 regulates SOX4 expression in RUNX1-rearranged acute leukemia. Mol Cancer. 2017;16:126.
- Qi X , Jiao Y , Cheng C , et al. H22954, a novel long non-coding RNA down-regulated in AML, inhibits cancer growth in a BCL-2-dependent mechanism. Cancer Lett. 2019;454:26–36.
- Yang Y , Yang H , Xu M , et al. Long non-coding RNA (lncRNA) MAGI2-AS3 inhibits breast cancer cell growth by targeting the Fas/FasL signalling pathway. Hum Cell. 2018;31:232–241.
- Li S , Garrett-Bakelman FE , Chung SS , et al. Distinct evolution and dynamics of epigenetic and genetic heterogeneity in acute myeloid leukemia. Nat Med. 2016;22:792–799.
- Figueroa ME , Lugthart S , Li Y , et al. DNA methylation signatures identify biologically distinct subtypes in acute myeloid leukemia. Cancer Cell. 2010;17:13–27.
- Yamazaki J , Taby R , Vasanthakumar A , et al. Effects of TET2 mutations on DNA methylation in chronic myelomonocytic leukemia. Epigenetics. 2012;7:201–207.
- Hanlon K , Rudin CE , Harries LW . Investigating the targets of MIR-15a and MIR-16-1 in patients with chronic lymphocytic leukemia (CLL). PLoS One. 2009;4:e7169.
- Jensen KB , Collins CA , Nascimento E , et al. Lrig1 expression defines a distinct multipotent stem cell population in mammalian epidermis. Cell Stem Cell. 2009;4:427–439.
- Majeti R , Becker MW , Tian Q , et al. Dysregulated gene expression networks in human acute myelogenous leukemia stem cells. Proc Natl Acad Sci U S A. 2009;106:3396–3401.
- Ritchie ME , Phipson B , Wu D , et al. limma powers differential expression analyses for RNA-sequencing and microarray studies. Nucleic Acids Res. 2015;43:e47.
- Dohner H , Weisdorf DJ , Bloomfield CD . Acute Myeloid Leukemia. N Engl J Med. 2015;373:1136–1152.
- Kreso A , Dick JE . Evolution of the cancer stem cell model. Cell Stem Cell. 2014;14:275–291.
- Shlush LI , Zandi S , Mitchell A , et al. Identification of pre-leukaemic haematopoietic stem cells in acute leukaemia. Nature. 2014;506:328–333.
- Wang Y , Krivtsov AV , Sinha AU , et al. The Wnt/beta-catenin pathway is required for the development of leukemia stem cells in AML. Science. 2010;327:1650–1653.
- Wang Y , He L , Du Y , et al. The long noncoding RNA lncTCF7 promotes self-renewal of human liver cancer stem cells through activation of Wnt signaling. Cell Stem Cell. 2015;16:413–425.
- Wang X , Sun W , Shen W , et al. Long non-coding RNA DILC regulates liver cancer stem cells via IL-6/STAT3 axis. J Hepatol. 2016;64:1283–1294.
- Trimarchi T , Bilal E , Ntziachristos P , et al. Genome-wide mapping and characterization of Notch-regulated long noncoding RNAs in acute leukemia. Cell. 2014;158:593–606.
- Jiang L , Lv L , Liu X , et al. MiR-223 promotes oral squamous cell carcinoma proliferation and migration by regulating FBXW7. Cancer Biomark. 2019;24:325–334.
- Powell AE , Wang Y , Li Y , et al. The pan-ErbB negative regulator Lrig1 is an intestinal stem cell marker that functions as a tumor suppressor. Cell. 2012;149:146–158.
- Lindquist D , Nasman A , Tarjan M , et al. Expression of LRIG1 is associated with good prognosis and human papillomavirus status in oropharyngeal cancer. Br J Cancer. 2014;110:1793–1800.
- Kou C , Zhou T , Han X , et al. LRIG1, a 3p tumor suppressor, represses EGFR signaling and is a novel epigenetic silenced gene in colorectal cancer. Biochem Biophys Res Commun. 2015;464:519–525.
- Delhommeau F , Dupont S , Della Valle V , et al. Mutation in TET2 in myeloid cancers. N Engl J Med. 2009;360:2289–2301.
- Yamazaki J , Jelinek J , Lu Y , et al. TET2 mutations affect Non-CpG Island DNA methylation at enhancers and transcription factor-binding sites in chronic myelomonocytic leukemia. cancer res. 2015;75:2833–2843.
- Ponciano-Gomez A , Martinez-Tovar A , Vela-Ojeda J , et al. Mutations in TET2 and DNMT3A genes are associated with changes in global and gene-specific methylation in acute myeloid leukemia. Tumour Biol. 2017;39:1010428317732181.
- Ichiyama K , Chen T , Wang X , et al. The methylcytosine dioxygenase Tet2 promotes DNA demethylation and activation of cytokine gene expression in T cells. Immunity. 2015;42:613–626.
- Bennett JM , Catovsky D , Daniel MT , et al. Proposals for the classification of the acute leukaemias. French-American-British (FAB) co-operative group. Br J Haematol. 1976;33:451–458.
- Mani R , Goswami S , Gopalakrishnan B , et al. The interleukin-3 receptor CD123 targeted SL-401 mediates potent cytotoxic activity against CD34(+)CD123(+) cells from acute myeloid leukemia/myelodysplastic syndrome patients and healthy donors. Haematologica. 2018;103:1288–1297.
- Ayuk SM , Abrahamse H , Houreld NN . The role of photobiomodulation on gene expression of cell adhesion molecules in diabetic wounded fibroblasts in vitro. J Photochem Photobiol B. 2016;161:368–374.
- Shields LE , Kiem HP , Andrews RG . Highly efficient gene transfer into preterm CD34 hematopoietic progenitor cells. Am J Obstet Gynecol. 2000;183:732–737.
- Ozdemir F , Altinisik J , Karateke A , et al. Methylation of tumor suppressor genes in ovarian cancer. Exp Ther Med. 2012;4:1092–1096.
- Alspach E , Stewart SA . RNA-binding protein immunoprecipitation (RIP) to examine AUF1 binding to Senescence-Associated Secretory Phenotype (SASP) factor mRNA. Biol Protoc. 2015;5:e1481.
- Hanaoka M , Tanaka K . Dynamics of RpaB-promoter interaction during high light stress, revealed by chromatin immunoprecipitation (ChIP) analysis in Synechococcus elongatus PCC 7942. Plant J. 2008;56:327–335.
- Nelson JD , Denisenko O , Sova P , et al. Fast chromatin immunoprecipitation assay. Nucleic Acids Res. 2006;34:e2.