ABSTRACT
Bacterial 6S RNA regulates transcription via binding to the active site of RNA polymerase holoenzymes. 6S RNA has been identified in the majority of bacteria, in most cases encoded by a single gene. Firmicutes including Bacillus subtilis encode two 6S RNA paralogs, 6S-1 and 6S-2 RNA. Hypothesizing that the regulatory role of 6S RNAs may be particularly important under natural, constantly changing environmental conditions, we constructed 6S RNA deletion mutants of the undomesticated B. subtilis wild-type strain NCIB 3610. We observed a strong phenotype for the ∆6S-2 RNA strain that showed increased biofilm formation on solid media and the ability to form surface-attached biofilms in liquid culture. This phenotype remained undetected in derived laboratory strains (168, PY79) that are defective in biofilm formation. Quantitative RT-PCR data revealed transcriptional upregulation of biofilm marker genes such as tasA, epsA and bslA in the ∆6S-2 RNA strain, particularly during transition from exponential to stationary growth phase. Salt stress, which blocks sporulation at a very early stage, was found to override the derepressed biofilm phenotype of the ∆6S-2 RNA strain. Furthermore, the ∆6S-2 RNA strain showed retarded swarming activity and earlier spore formation. Finally, the ∆6S-1&2 RNA double deletion strain showed a prolonged lag phase of growth under oxidative, high salt and alkaline stress conditions, suggesting that the interplay of both 6S RNAs in B. subtilis optimizes and fine-tunes transcriptomic adaptations, thereby contributing to the fitness of B. subtilis under the unsteady and temporarily harsh conditions encountered in natural habitats.
Introduction
Bacterial 6S RNAs are non-coding RNAs, 200 ± 50 nucleotides (nt) in length [Citation1]. They compete with DNA promoters for binding to RNA polymerase (RNAP) holoenzymes, thereby regulating transcription at a global level. 6S RNA adopts a rod-shaped structure with a largely single-stranded internal region (termed “central bulge“) flanked by imperfectly helical arms on both sides that partly adopt B-DNA-like conformations. This way they mimic an open DNA promoter structure and compete with DNA promoters for binding to the active site of RNAP [Citation2,Citation3]. Although 6S RNA globally interferes with transcription, 6S RNA deletions in several bacteria caused mostly minor phenotypes (see below). This has been surprising taking the RNA’s remarkable abundance, conservation, and widespread occurrence among bacteria into account [Citation1]. As an example, even the hyperthermophilic bacterium Aquifex aeolicus expresses a 6S RNA [Citation4,Citation5], although the bacterium has an extremely condensed genome of 1.6 Mbp and lost another ubiquitous non-coding RNA, the catalytic RNA subunit of RNase P, owing to replacement of RNA-based RNase P with a protein-only enzyme [Citation6]. E. coli cells lacking 6S RNA were reported to have reduced survival rates in stationary phase but to better survive at high pH [Citation7,Citation8]. Moreover, the 6S RNA knockout led to modestly increased cellular ppGpp levels during early stationary phase owing to an upregulation of the ppGpp synthase RelA [Citation9]. A major function of ppGpp is to dampen rRNA transcription and to induce the expression of genes involved in amino acid biosynthesis during early stationary growth. In later stationary phase, 6S RNA was inferred to continuously downregulate transcription, particularly at a subset of the amino acid biosynthetic gene promoters, but here in a ppGpp-independent manner [Citation9]. Overall, the findings indicate a role of E. coli 6S RNA in downregulating transcription upon nutrient deprivation and possibly during adaptation to other stresses. For stationary growth, this is mechanistically achieved by repression of a subset of σ70-dependent promoters and activation of σS-dependent transcription, including the control of transcription of several global regulators such as ppGpp, reviewed in [Citation10,Citation11].
In the soybean symbiont Bradyrhizobium japonicum (rhizobia, α-proteobacteria), 6S RNA levels were found to be elevated relative to free-living cells when B. japonicum formed bacteroids in root nodules of the host plant [Citation12]. In the photosynthetic α-proteobacterium Rhodobacter sphaeroides, a 6S RNA knockout resulted in a growth defect under high salt stress 0.25 M NaCl [Citation13]. In the phototrophic cyanobacterium Prochlorococcus MED4, upon cell cycle synchronization by light–dark cycles, 6S RNA levels decreased during night periods, suggesting a role for the RNA in regulating transcriptome adaptations in the course of light–dark cycles [Citation14]. In another cyanobacterium, Synechocystis sp. PCC 6803, 6S RNA was found to act as an integral part of the cellular response to changing nitrogen availability by accelerating the switch from group 2 sigma factor σB-, σC- and σE- to σA-dependent transcription [Citation15]. A study of the human pathogen Salmonella enterica serovar Typhimurium revealed a role for 6S RNA in survival under extremely acidic conditions (pH 3.0) and for invasion of epithelial cells during pathogenesis [Citation16]. In the γ-proteobacterium Legionella pneumophila, a human pathogen encoding two 6S RNAs with different expression profiles [Citation17,Citation18], a knockout of the initially identified 6S RNA reduced intracellular multiplication of the bacterium in protist and mammalian host cells; however, the knockout had no detectable effect on growth in rich media [Citation17]. This provides an example that biologically relevant 6S RNA knockout phenotypes may remain undetected under standard laboratory growth conditions. Interestingly, evidence was provided that the second 6S RNA of L. pneumophila is regulated by cis-encoded antisense transcripts [Citation18].
B. subtilis, the model organism used in the presented study, also possesses two 6S RNA paralogs. Both have the ability to block the σA-RNAP housekeeping holoenzyme and to serve as templates for the synthesis of short de novo transcripts, called product RNAs (pRNAs). Such pRNAs, if long enough (≥14 nt) to remain stably bound to the 6S RNA template, induce refolding of 6S RNA and its dissociation from σA-RNAP [Citation19-23]. A notable difference between the two 6S RNA paralogs is their distinct expression profile: 6S-1 RNA levels peak at the transition to stationary phase, while 6S-2 RNA levels were observed to remain largely constant over the entire growth period [Citation24,Citation25], or to peak between early and mid-exponential phase and to decrease in stationary phase [Citation2,Citation19,Citation26]. This observed discrepancy might suggest strain-specific differences. There is also evidence that pRNA synthesis by σA-RNAP is less efficient and dynamic on 6S-2 relative to 6S-1 RNA [Citation22,Citation27]. This can be attributed to σA-RNAP being more efficient in initiating 6S-1 pRNA transcription with GTP than 6S-2 pRNA synthesis with ATP [Citation19,Citation27]. In the B. subtilis strain PY79, a derivative of the widely used 168 strain, simultaneous deletion of both 6S RNA genes (bsrA encoding 6S-1 RNA, bsrB 6S-2 RNA) caused an accelerated decrease of optical density towards extended stationary phase and a faster outgrowth from stationary phase under extremely alkaline conditions (pH 9.8). Several proteins deregulated in the single or double 6S RNA knockouts of B. subtilis PY79 were assigned to metabolic functions and stress responses [Citation28]. In B. subtilis strain 168, deletion of 6S-1 RNA, considered to be the functional homolog of E. coli 6S RNA based on its expression profile [Citation2,Citation19], caused a delay in outgrowth from stationary phase and resulted in an earlier onset of sporulation, which was attributed to faster exhaustion of nutrients [Citation24]. We were unable to observe this phenotype for the PY79 strain, which may be attributable to different strain backgrounds.
The function of 6S RNA as an inhibitor of transcription was identified ~20 years ago [Citation29], but our understanding of its complex regulatory effects is still fragmentary. This pertains even more to the intricate interplay of the two 6S RNAs in B. subtilis. In view of the remarkable abundance, conservation and dissemination of 6S RNA among bacteria [Citation1], it is surprising that the phenotypes of 6S-1 and/or 6S-2 gene knockouts in B. subtilis strains 168 and PY79 [Citation24,Citation28,Citation30] were quite modest. We hypothesized that 6S RNA might be more crucial in wild-type strains with their more comprehensive genetic repertoire that evolved to cope with the persistent exposure to rapidly changing growth conditions and environmental stresses.
The wild-type ancestor of the well-studied laboratory strains (B. subtilis 168 and PY79) is strain NCIB 3610 [Citation31], which has retained functions that are impaired or were lost in derived laboratory strains. This includes production of antibiotic compounds, biofilm/pellicle and fruiting body formation, motility, and sporulation [Citation32–36]. In some cases, the reason for the loss of a wild-type feature could be attributed to just a few point mutations in the laboratory strain. For example, a frame shift mutation in the sfp gene combined with a 1-bp insertion in the swrA gene leads to abrogation of surfactin production and consequently loss of swarming ability in strains PY79 and 168 [Citation34]. A point mutation in the epsC gene involved in exopolysaccharide capsule formation as well as loss of the plasmid-borne rapP gene contribute to defective biofilm formation in strain 168 [Citation35]. Another point mutation in the −10 region of the degQ promoter inhibits expression of the pleiotropic regulator DegQ [Citation35]. In addition, some genes relevant to amino acid metabolism, gudB and trpC, differ between B. subtilis wild-type and laboratory strains. The gudB gene is cryptic in the laboratory strain 168 owing to an 18 bp-long repeat making GudB unstable and inactive [Citation37]. Strain 168 possesses a 3-bp deletion at the trpC locus (trpC2) causing tryptophan auxotrophy [Citation38]. Furthermore, some other studies addressed genomic differences between several B. subtilis strains and could identify even more differences that potentially impair protein function and hide natural features in laboratory strains [Citation31,Citation39]. Thus, we chose the NCIB 3610 strain to construct 6S-1/2 RNA single and double knockouts towards understanding the physiological role of both 6S RNAs in B. subtilis.
Results
Expression profiles of 6S-1 and 6S-2 RNA in B. subtilis strain NCIB 3610
As a first step, we analysed the expression profiles of 6S-1 and 6S-2 RNA in B. subtilis strain NCIB 3610. For this purpose, total RNA was extracted along the growth curve in rich LB medium () and subjected to Northern blot analysis using probes specific for 6S-1 RNA () or 6S-2 RNA (). The levels of 6S-1 RNA, appearing as a 201-nt precursor form and a 190-nt mature RNA, peak in late exponential to stationary phase (), whereas 6S-2 RNA levels are highest during exponential phase and level off towards stationary phase (). These findings are in line with 6S RNA expression profiles observed for the derived 168 laboratory strain [Citation19].
Figure 1. Expression of the two 6S RNAs in the undomesticated B. subtilis strain NCIB 3610. (A) Representative growth curve in LB medium at 37°C. Filled dots represent growth points at which samples were withdrawn for total RNA extraction (a-h). (B, C) Expression of 6S-1 RNA (B) and 6S-2 RNA (C) analysed by Northern hybridization using 5S rRNA as loading control. For each analysed time point of the growth curve (a to h, panel A), the OD600 value at cell withdrawal is also given above each lane. RNAs were separated by 12% denaturing PAGE. See Materials and Methods for more experimental details
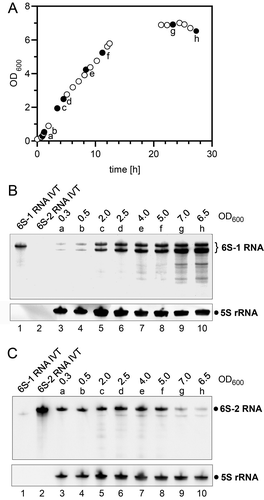
Construction of NCIB 3610 6S RNA deletion and respective complementation strains
Taking into account that the undomesticated B. subtilis strain NCIB 3610 is poorly susceptible to transformation, we used the thermosensitive integrative vector pMAD for allelic replacement/deletion to construct single and double 6S RNA gene knockouts [Citation40]. This strategy is laborious but obviates the need for a selection marker. Successful gene deletion was identified by size analysis of PCR fragments covering the 6S RNA loci and verified by DNA sequencing of the respective PCR fragments of candidate clones. Northern blot analyses were performed to visualize the absence of 6S RNAs in the deletion mutants (). In addition, qRT-PCR experiments confirmed the loss of 6S RNA transcripts in the corresponding 6S RNA single and double knockout strains (Fig. S1). In the single knockout strains, levels of the remaining 6S RNA did not change substantially ( and S1). Although the 6S-2 RNA-specific blot suggested a minor increase of 6S-2 RNA levels during exponential growth in the Δ6S-1 strain (, cf. lanes 1 and 2), this was not substantiated in the qRT-PCR quantification (Fig. S1, left panel).
Figure 2. Northern blot analysis of NCIB 3610 6S RNA knockout and complementation strains. Total bacterial RNA from 6S RNA knockout strains and 6S RNA complementation strains was isolated from cells in (A) stationary and (B) exponential growth phase. (A) The Northern blot analysis verifies deletion of the 6S-1 RNA gene in the Δ6S-1 amyE::cat strain and successful reintegration of the 6S-1 RNA gene in the corresponding complementation strain (Δ6S-1 amyE::6S-1:cat). The 6S-1 RNA-specific double band represents the 5ʹ-precursor (201 nt) and mature 6S-1 RNA (190 nt), respectively. 5S rRNA served as a loading control. (B) Corresponding Northern blot analysis for the Δ6S-2 strain. RNAs were separated by 10% denaturing PAGE. For more details, see Materials and Methods. Δ6S-1&2, double knockout of both 6S RNA genes; IVT, in vitro T7 transcripts of 6S-1 or 6S-2 RNA. The shown blots represent one of two independent experiments that gave very similar results
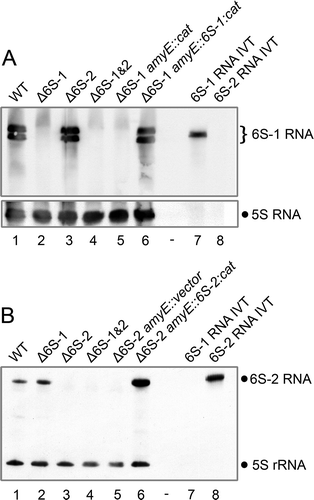
To be able to assign potential phenotypes of Δ6S RNA strains to the absence of the respective 6S RNA, that is, to exclude indirect effects caused by the chromosomal alteration, we constructed complementation strains in which the deleted 6S RNA gene was reintroduced at the amyE locus. Either the 6S-1 or 6S-2 RNA gene was reintroduced into the 6S RNA double knockout strain (). Complementation strains were constructed in two steps using a different and less cumbersome strategy involving chloramphenicol acetyltransferase (CAT) as selection marker. In the first step, we integrated the 6S-1 or 6S-2 RNA gene together with the cat gene into the amyE locus of the competent NCIB 3610 comIQ12L strain DK1042 [Citation41]. In the second step, extracted chromosomal DNA from such comIQ12L clones was used to transfer the genotypes into the 6S RNA knockout strains (and the parental NCIB 3610 strain as control) under chloramphenicol (CM) selection pressure. Successful complementation at the amyE locus of CM-resistant transformants was verified by DNA sequencing of PCR fragments covering the amyE locus as well as by Northern blotting (). For unknown reasons, increased 6S-2 RNA levels were observed in the corresponding Δ6S-2 amyE::6S-2:cat complementation strain relative to the NCIB 3610 wild-type strain (, cf. lanes 1 and 6).
Table 1. Strains and plasmids used in this study
Deletion of the 6S-1 RNA gene causes growth deficiency during extended stationary phase
In LB medium at 28°C, the NCIB 3610 Δ6S-1 strain reproducibly grew to lower optical density during extended stationary phase (measured in plate reader experiments, ). Upon reintegration of the 6S-1 RNA gene at the amyE locus, the growth behaviour was reverted to that of the parental strain, indicating that the absence of 6S-1 RNA is responsible for the observed phenotype during extended stationary phase.
Figure 3. Deletion of the 6S-1 RNA gene leads to accelerated decrease of cell density in late stationary phase. Bacterial cultures of the NCIB 3610 wild-type-like strain (WT amyE::cat), the corresponding 6S-1 RNA deletion strain (Δ6S-1 amyE::cat) and the complementation strain (Δ6S-1 amyE::6S-1:cat) were grown in LB medium at 28°C and OD600 was monitored using a plate reader. Standard deviations are based on two independent biological replicates with three technical replicates each
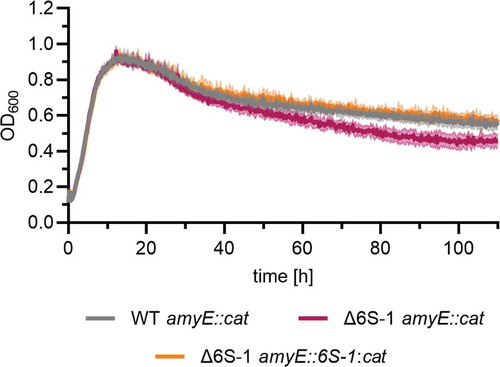
Deletion of the 6S-2 RNA gene results in enhanced biofilm formation
We previously deleted the 6S-1 and/or 6S-2 RNA gene in the B. subtilis laboratory strain PY79 [Citation28]. Growth measurements of the PY79 strain and its three 6S RNA deletion derivatives in the plate reader at 28°C in LB medium revealed little differences between these strains up to early stationary growth (). However, in the NCIB 3610 background, we observed an aberrant growth behaviour of the Δ6S-2 strain (), characterized by a wide scattering of optical density values (high standard deviations). Normal growth behaviour could be restored by ectopic reintegration of the 6S-2 RNA gene into the amyE locus (), verifying that the phenotype was due to the absence of 6S-2 RNA. Inspection of plate reader wells identified surface-attached biofilm formation at the bottom of the wells () as the reason for abnormal growth behavior of the NCIB 3610 Δ6S-2 strain. Biofilm formation of the parental NCIB 3610 strain and its 6S RNA knockout mutants was then tested on solid MSgg agar plates [Citation44]. Characteristics of biofilms are central ring zones and highly wrinkled ridges [Citation45]. Differences in colony morphology were reproducibly observed to be most pronounced for the NCIB 3610 Δ6S-2 mutant ( and S2), in line with the phenotype in the plate reader setup. Particularly the ridges in the centre of biofilms were fortified and the radii of colonies of the Δ6S-2 RNA strain tended to be larger than those of the parental wild-type strain. Also, Congo Red staining emphasized the overall increase of biofilm matrix formation in the absence of 6S-2 RNA (, column d). The three-dimensional colony phenotype was reverted in the 6S-2 RNA complementation strain, which allowed us to assign the biofilm enhancement phenotype to the absence of 6S-2 RNA (). As expected, the B. subtilis laboratory strain PY79 was unable to form biofilms (Fig. S2).
Figure 4. Loss of 6S-2 RNA (bsrB deletion) in the undomesticated B. subtilis strain NCIB 3610 enhances biofilm formation. (A, B) Growth curves of B. subtilis 6S RNA deletion strains, either in the (A) PY79 or (B) NCIB 3610 strain background. Optical density (OD600) was monitored in a plate reader in LB medium at 28°C. WT: parental PY79 or NCIB 3610 strain; ∆6S-1, ∆6S-2, ∆6S-1&2: strain-specific single or double knockout derivative strains. (C) Growth (as in panels A and B) of the NCIB 3610 6S-2 RNA complementation strain (Δ6S-2 amyE::6S-2:cat) and its reference strains WT amyE::cat and Δ6S-2 amyE::cat, demonstrating that loss of 6S-2 RNA is responsible for the biofilm formation phenotype of the deletion strain. Standard deviations in panels A to C are based on at least two biological replicates with three technical replicates each. (D) Close-up image of a 96-well plate after finishing a typical plate reader growth experiment. Shown are two wells each for the NCIB 3610 wild-type (WT) and the ∆6S-2 RNA derivative strain. In contrast to the WT, the 6S-2 RNA knockout strain formed surface-attached biofilms at the bottom of the wells upon incubation of the bacterial cultures in LB medium at 28°C. (E) Enhanced biofilm formation of the ∆6S-2 RNA strain on solid MSgg agar plates. The four strains (NCIB 3610 background) were spotted onto MSgg agar plates (containing Congo Red and Coomassie Brilliant Blue dyes) and incubated for several days at 30°C, revealing differences in their three-dimensional colony structures. The panel shows one representative experiment out of three independent ones that gave comparable results. Columns a and b are black-and-white photographs; columns b-d represent the same colony set, photographed in black and white (b) or in colour from the top (c) or as side view (d). (F) The same strains as analysed in panel C were spotted onto MSgg agar plates (containing Congo Red and Coomassie Brilliant Blue dyes) and grown at 30°C for 6 days. The panel shows the result of one representative out of three independent experiments. Columns a-c represent the same colony set, photographed in black and white (a) or in colour from the top (b) or as side view (c)
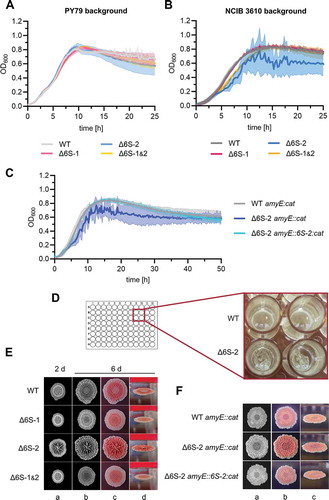
The biofilm phenotype of the Δ6S-2 RNA knockout analysed at different temperatures
We analysed growth of the four NCIB 3610 strains in a plate reader in LB medium at 37°C, 28°C, 22.5°C and 18°C. Surface-attached biofilm formation of the NCIB 3610 Δ6S-2 strain, as inferred from high standard deviations of optical density values (), appeared more pronounced at temperatures below 37°C. Likewise, the enhanced biofilm formation phenotype of the Δ6S-2 strain relative to the three other strains seemed more pronounced when comparing colony morphology on solid MSgg agar plates grown for 6 days at 20°C () versus 30°C (). This is also in line with the relative extent of Congo Red staining of the biofilm matrix polysaccharides [Citation46] at the two growth temperatures. While all four strains were substantially imbued at 30°C (), biofilm staining at 20°C was essentially confined to the Δ6S-2 strain ().
Figure 5. Manifestation of the Δ6S-2 RNA biofilm phenotype at different growth temperatures. Growth of the NCIB 3610 strain and its 6S RNA deletion derivatives was measured in a plate reader in LB medium at the indicated temperatures (37°C/28°C/22.5°C/18°C). Precultures were cultivated at 37°C before inoculating the main cultures to avoid pre-adaptation to lower temperatures. Standard deviations are calculated from at least two biological replicates and three technical replicates each
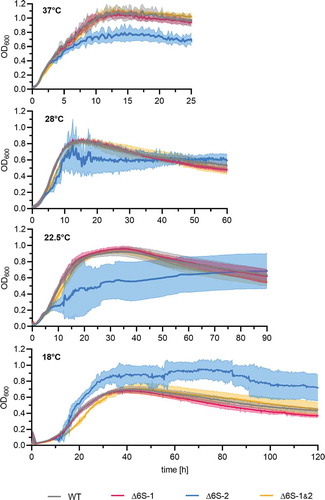
Figure 6. Incubation at 20°C influences biofilm manifestation on solid MSgg agar plates. Aliquots of cell suspensions (NCIB 3610 WT, 6S-1/6S-2 RNA knockout, double 6S RNA knockout) were spotted onto MSgg agar plates (containing Congo Red and Coomassie Brilliant Blue dyes) and incubated for up to 6 days at 20°C. The shown images represent one of three independent experiments yielding comparable results
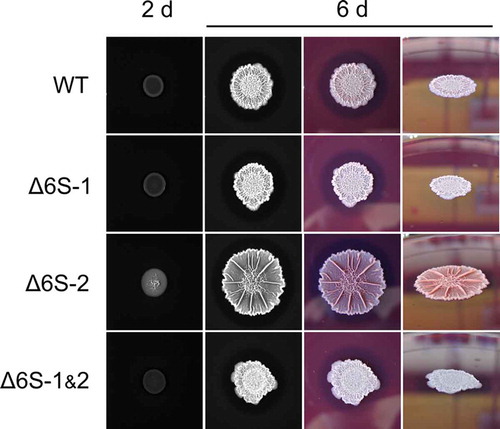
mRNA levels of biofilm-related genes are upregulated in the Δ6S-2 RNA knockout mutant
To address the molecular basis of upregulation of biofilm formation in the Δ6S-2 mutant, we quantified the mRNA levels of selected biofilm marker genes (tasA, epsA and bslA) via qRT-PCR [Citation47,Citation48] using total RNA of cells grown at 37°C or 20°C (). While mRNA levels of the biofilm-related genes tasA, epsA and bslA were moderately increased in exponential phase relative to NCIB 3610 wild-type cells, this expression difference increased during transition from exponential to stationary phase (). Expression changes were similar at 37°C and 20°C, although epsA showed a stronger expression difference at 20°C in exponential phase.
Figure 7. Increased biofilm formation ability of the 6S-2 RNA knockout mutant correlates with elevated mRNA levels of main biofilm matrix components. Strains NCIB 3610 and 3610_Δ6S-2 were grown in LB medium at (A) 37°C or (B) 20° and total RNA was isolated in exponential phase (OD600 ~ 0.3 − 0.5; left panel) or at the transition from exponential to stationary phase growth (OD600 ~ 3.5–4.0; right panel); mRNAs encoding central biofilm matrix proteins (tasA, epsA, bslA) were quantified by qRT-PCR using the ΔΔCT-value method and 5S rRNA for normalization; a 2−ΔΔCT value of 1 corresponds to equal mRNA levels in the Δ6S-2 and wild-type strain; 2−ΔΔCT values and standard errors of the mean (SEM) are based on four (panel A) or two (panel B) independent biological replicates with at least two technical replicates each
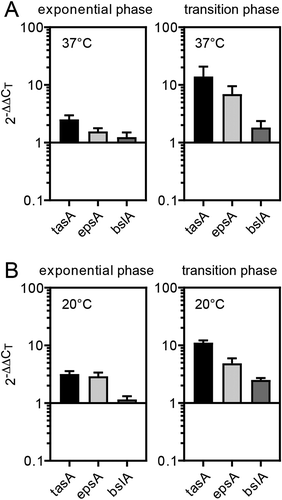
Interplay of both 6S RNAs is important to cope with environmental stresses
To explore if 6S RNAs play a role in adaption to environmental stresses in the B. subtilis NCIB 3610 wild-type strain, we tested bacterial growth under high osmolarity as well as oxidative and alkaline pH stress in the plate reader experimental setup. The 6S RNA double knockout mutant showed growth retardation under all three conditions, as inferred from a more extended lag phase (). The Δ6S-1 strain showed similar growth behaviour as the wild type under all three stress conditions. Remarkably, the Δ6S-2 biofilm phenotype was suppressed at 0.8 M NaCl.
Figure 8. The NCIB 3610_Δ6S-1&2 double knockout strain has impaired proficiency to adapt to stresses. Precultures of the NCIB 3610 strain and its three derivative strains (Δ6S-1, Δ6S-2 and Δ6S-1&2) were grown in LB medium at 37°C before inoculating the main cultures under the specified stress conditions. Oxidative stress: LB medium supplemented with 50 µM H2O2; high salt stress: MSgg medium containing 0.8 M NaCl; alkaline stress: LB medium adjusted to pH 9.5. The optical density at 600 nm (OD600) was measured in a plate reader. Standard deviations are based on two independent biological replicates with three technical replicates each
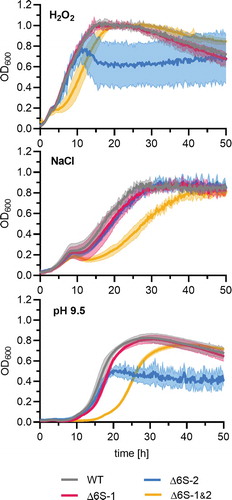
The Δ6S-2 RNA knockout strain has retarded swarming ability
As biofilm formation was found to be enhanced in the Δ6S-2 strain, we asked if swarming motility may also be affected in this mutant. For this purpose, liquid cultures of strains WT amyE::cat, Δ6S-2 amyE::cat and Δ6S-2 amyE::6S-2:cat were grown in LB medium with shaking at 220 rpm using baffled Erlenmeyer flasks. Under these conditions, biofilm formation of the Δ6S-2 strain was less evident (Fig. S3), likely due to vigorous aeration and associated mechanical forces. At a defined OD600 (2.0), 2 µl of liquid culture were spotted onto 0.7% TG swarm agar plates (for details, see Material and Methods), followed by incubation of the plates at 28°C. Every hour the distance from the colony centre to the swarm front (= radius) was measured (). The resulting graph (radius over time) revealed slower swarming kinetics of the Δ6S-2 strain and restoration of wild-type-like swarming kinetics for the 6S-2 RNA complementation strain (). Thus, we identified another physiologically relevant function affected by the 6S-2 RNA knockout in the NCIB 3610 background.
Figure 9. Bacteria lacking 6S-2 RNA show retarded swarming behaviour. Strain NCIB 3610 amyE::cat (WT amyE::cat), the derived 6S-2 RNA knockout mutant strain (Δ6S-2 amyE::cat) and the latter complemented with the 6S-2 RNA gene (Δ6S-2 amyE::6S-2:cat) were analysed for the time dependence of swarming. When liquid cell cultures grown at 37°C reached an OD600 of 2.0, 2 µl of culture were spotted in the centre of a swarm plate (0.7% TG agar) that was incubated at 28°C. (A) Example swarm plates after 10 h of incubation at 28°C. Dashed semi-circles mark the boundary of the swarm area; the distance/radius from the initial spot centre to the outer swarm front (double arrows) was measured at various time points, providing the basis for the graph in panel B. (B) Swarming kinetics of the three analysed strains. Values are based on three independent experiments with five technical replicates each. Error bars indicate standard errors of the mean (SEM). For more details, see Materials and Methods
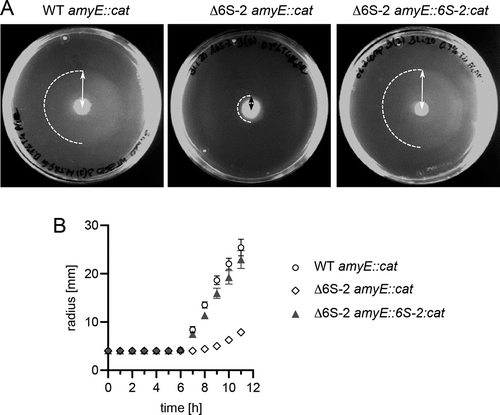
The Δ6S-2 RNA knockout strain shows accelerated spore formation
As biofilm formation and sporulation are interdigitated cellular processes [Citation45], it seemed possible that the Δ6S-2 strain also differs from the parental NCIB 3610 wild-type strain in some aspect of sporulation. We grew the four strains in sporulation medium and withdrew cell culture aliquots at different time points (6, 8, 10 and 24 h), which were split into two fractions. One fraction was plated directly in serial ten-fold dilutions, and the other fraction was heated to 80°C for 20 min before plating which kills vegetative cells but allows spores to survive. This then yields the ratio of colony numbers after heat treatment divided by those before heating. In these experiments, we noticed that the fraction of spores was negligible at 6 h and approached its maximum at 10 h for all strains (65% to 80% spores, not shown). However, after 8 h, we reproducibly observed a higher fraction of spores for the Δ6S-2 strain and a considerably reduced fraction for the double knockout strain ().
Figure 10. Fraction of spores after 8 h of growth in sporulation medium. The fraction of spores was determined by counting the number of colonies before and after heating cell culture dilutions to 80°C for 20 min and calculating the ratio of ‘colonies heated/colonies not heated’. At 10 h, the relative number of spores became more similar for all strains (65 to 80%, not shown). The substantially higher fraction of spores formed by the Δ6S-2 strain after 8 h suggests accelerated spore formation in this strain. For details, see Materials and Methods
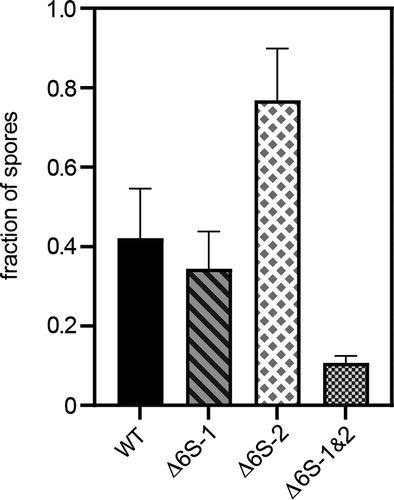
Discussion
The use of the undomesticated NCIB 3610 wild-type strain for studying the consequences of 6S RNA gene deletions revealed previously unrecognized phenotypes for the 6S-2 RNA single and the double knockout strains. No new phenotypic features were observed for the 3610_Δ6S-1 strain under the conditions tested in the present study. The latter mutant strain showed reduced optical density during extended stationary growth (), a feature shared with the laboratory derivative strain PY79 [Citation28]. One possible explanation is that cells lacking 6S-1 RNA consume nutrients more rapidly than the parental strain and initiate processes mediated by nutrient deprivation, such as cell lysis, cell size alterations and/or sporulation, more rapidly. Major support for this notion came from a study of a 6S-1 RNA knockout in the B. subtilis 168 strain background, showing that the absence of 6S-1 RNA leads to an earlier onset of sporulation which is attributable to faster consumption of nutrients [Citation24].
For the first time, we observed a strong phenotype of a Firmicute 6S-2 RNA knockout strain. This mutant showed an increased relative propensity to form biofilms, particularly evident at lower temperatures such as ~20°C. This was observed on solid biofilm-inducing MSgg agar plates and in plate reader liquid growth experiments () where surface-attached biofilms formed at the bottom of the wells (). Accordingly, genes encoding markers of biofilm formation (tasA, epsA and bslA) were found to be derepressed in the absence of 6S-2 RNA, particularly at the transition from exponential to stationary phase (). Yet, qRT-PCR quantification of tasA, epsA and bslA mRNAs did not reveal major differences in their extent of upregulation in Δ6S-2 cells grown at 37°C versus 20°C (), except for a larger upregulation of epsA mRNA in exponential phase at 20°C. The latter observation might be considered as correlating with the marked colony phenotype observed at 20°C (), but this is speculative at present.
Biofilms designate communities of tightly associated bacteria encased in an extracellular matrix. Biofilms of B. subtilis start to form when motile cells in ageing cultures differentiate to non-motile, matrix-producing cells that form cell chains and three-dimensional aggregates encapsidated by a matrix consisting of exopolysaccharides (EPS) and proteins. In mature biofilms, which retain a small subpopulation of motile cells, matrix-producing cells start to sporulate. In aged biofilms, decomposition of biofilms leads to the release of motile cells and spores (reviewed in [Citation45]). Matrix formation and sporulation are processes regulated by several intertwined pathways, a major one being the Spo0A pathway. The transcription factor Spo0A controls the expression of more than 100 genes and its activity depends on phosphorylation, where intermediate levels of phosphorylated Spo0A (Spo0A-P) induce the expression of biofilm matrix genes, while higher Spo0A-P levels trigger sporulation. Spo0A-P levels are determined by the activity of several kinases (KinA-D) that phosphorylate Spo0A either directly or indirectly via a so-called phosphorelay [Citation45]. Spo0A-P triggers matrix gene expression in a subset of cells by inducing SinI expression; SinI then sequesters SinR, a DNA repressor of matrix gene expression. Biofilm formation is further fine-tuned and coordinated by Spo0A-P-mediated repression of a second matrix gene repressor, AbrB, and by interdigitation with at least three additional regulatory subnetworks (reviewed in [Citation45]).
At present, it remains speculative at which level the absence of 6S-2 RNA may induce derepression of matrix gene expression in the NCIB 3610_Δ6S-2 strain. As this phenotype is not observed in the Δ6S-1 and Δ6S-1&2 strains, the molecular basis for its specificity must go beyond a simple change of the equilibrium between transcription-competent and 6S RNA-inhibited σA-RNAP holoenzymes. The phenotype might reflect a transcriptional effect that occurs in early exponential phase when 6S-2 RNA levels peak and those of 6S-1 RNA are still very low. However, the impact on matrix gene expression may come into full effect only at a later stage, as we demonstrated by qRT-PCR that mRNA levels for the biofilm marker proteins tasA, epsA and bslA are particularly upregulated in the transition growth phase, where 6S-1 RNA levels are substantial and exceed those of 6S-2 RNA ([Citation28]; ).
Higher tasA, epsA and bslA expression in the transition relative to the exponential phase of growth observed for the Δ6S-2 RNA strain () may be explained by more efficient repression of matrix gene expression in exponential versus transition phase. Although matrix gene repression is relaxed in the Δ6S-2 strain, it may still be quite effective in exponential phase when biofilm formation is biologically inappropriate. However, in the transition phase, when repression is relieved to favour matrix gene expression in some cells of the population, the relaxation of repression in the Δ6S-2 strain may take full effect. Also, substantially less biofilm formation is observed for the three reference strains at lower temperatures (), suggesting that the Δ6S-2 strain may be less responsive to environmental signals that normally suppress the expression of biofilm matrix genes. Altogether, our findings suggest a constitutive derepression of biofilm gene expression in the NCIB 3610 Δ6S-2 strain. RNA-Seq analyses of the knockout strains may provide clues as to which of the subnetworks that regulate biofilm formation might be affected in the Δ6S-2 strain.
We also considered the possibility that the particularly pronounced biofilm phenotype of the Δ6S-2 strain at lower temperatures () may report a syntenic link to temperature adaptation in B. subtilis. The 6S-2 RNA gene (bsrB) is located close to the des operon (3.6 kb end-to-end distance in opposite orientation). The des operon is known to be involved in cold shock adaptation of B. subtilis by controlling membrane fluidity [Citation49]. A consequence of this synteny may be a local increase of the 6S-2 RNA concentration at the site of its transcription, which might directly dampen the expression of nearby genes including the des operon. Such a local effect on gene expression of genes in vicinity of the 6S RNA gene was considered to explain the phenotype of a 6S RNA gene knockout in Rhodobacter sphaeroides. In this α-proteobacterium, the salt stress-induced gene sspA is encoded close to the 6S RNA gene (ssrS) and an ssrS knockout resulted in a salt stress phenotype [Citation13]. To examine the possibility of such a local effect in B. subtilis, we compared biofilm formation for the 6S-2 RNA complementation strain Δ6S-2 amyE::6S-2:cat, the corresponding wild-type-like parental strain (WT amyE::cat) and the Δ6S-2 RNA strain (Δ6S-2 amyE::cat). Although expressed from another location on the chromosome, 6S-2 RNA complementation reversed the biofilm phenotype at 20°C (Fig. S4B), suggesting that such local effects of the 6S-2 RNA gene do not play a role here.
Another link might be to the alternative transcription factor sigma B (σB) that is activated in B. subtilis under several stress conditions [Citation50] including adaptation to lower temperatures [Citation51,Citation52]. The phenotype we observed for the 6S-2 RNA deletion strains is very similar to that of a B. subtilis SigB deletion strain, i.e. enhanced biofilm formation, increased epsA and bslA expression and reduced swarming ability [Citation53]. This suggests a functional link between the transcriptional regulator 6S-2 RNA and the general stress transcription factor SigB. It is also a possibility that 6S-2 RNA, e.g. due to potential changes in secondary structure at lower temperature, may support the replacement of σA by σB on RNAP, although this is speculative at present. Of note, expression of the sigB operon is controlled by a σB-dependent promoter and a CRE binding site for the carbon catabolite control protein A (CcpA), and evidence was provided that CcpA is required for σB-dependent stress responses [Citation54]. Interestingly, in our previous study on target proteins affected by 6S RNA deletions in the B. subtilis PY79 laboratory strain, six proteins belonging to the CcpA regulon and five putative interaction proteins of CcpA were identified as candidates of aberrant expression in the Δ6S-1 and/or Δ6S-2 RNA strain in a growth phase-dependent manner [Citation28].
6S-1 and 6S-2 RNA of B. subtilis were shown to be able to bind to the housekeeping σA-RNAP holoenzyme to inhibit transcription at DNA promoters [Citation21,Citation25]. So far, it is not clear whether one or both of the two 6S RNAs are able to bind to RNAP when complexed with alternative sigma factors such as σB. Irrespectively, it is a possibility that the absence of 6S-2 RNA may cause an imbalance in the pool of sigma factors and the distribution of the different RNAP holoenzymes, possibly perturbing downstream pathways such as the general stress response. Finally, we cannot exclude the possibility that the biofilm phenotype of the NCIB 3610 Δ6S-2 strain is due to a specialized moonlighting function of 6S-2 RNA, rather than resulting from inhibition/sequestration of RNAP holoenzymes.
Remarkably, the biofilm phenotype of the 6S-2 RNA knockout mutant disappeared when cells were grown under high salt stress (0.8 M NaCl; ). In high-salinity environments B. subtilis blocks entry into sporulation to prevent commitment to a developmental programme that cannot be completed under high salt conditions [Citation55]. This block is achieved by downregulating transcription of genes under control of the sporulation master regulator Spo0A and the sporulation-specific sigma factor σH; additionally, association of the sporulation-specific sigma factor σH with core RNAP is reduced under these conditions [Citation55]. As Spo0A also controls biofilm matrix gene expression see above [Citation45,Citation56,Citation57], it is not too surprising that Spo0A downregulation during high osmolarity adaptation overrides the derepression of biofilm genes in the Δ6S-2 strain.
Interestingly, the 6S RNA double knockout strain (Δ6S-1&2) showed phenotypes that differed from those of the 6S RNA single knockout mutants. In liquid cultures (plate reader experiments), we observed a slight growth retardation from mid-exponential to stationary phase in the Δ6S-1&2 strain particularly at 28°C, 22.5°C and 18°C ( and S3). This kind of growth retardation exacerbated under oxidative, high salt and alkaline stress conditions (). We conclude that the interplay of both 6S RNAs is important for the adaption to changing environments. Evidently, the two 6S RNAs of B. subtilis constitute a well-balanced, interdigitated regulatory system that contributes to the adaptation and fine-tuning of gene expression.
In the PY79 Δ6S-1&2 strain, we observed two growth phenotypes that were more pronounced for the double knockout than for any of the corresponding single knockouts: (i) accelerated decrease of optical density towards extended stationary phase and (ii) faster outgrowth from stationary phase under alkaline stress conditions (pH 9.8) [Citation28]. These phenotypes of the PY79 Δ6S-1&2 strain were not evident here in the analysis of the NCIB 3610 Δ6S-1&2 strain, illustrating the importance of strain background.
Our study further revealed retarded swarming behaviour of the 6S-2 RNA knockout mutant (). Biofilms and bacterial swarms feature multicellular communities, where division of labour drives the specialization of phenotypically different subpopulations to increase fitness of the entire bacterial population [Citation58,Citation59]. For instance, motile cells, surfactin producers, competent cells, matrix producers, spores and cannibal-like cells are able to coexist within a multicellular biofilm colony [Citation60]. With respect to our NCIB 3610 Δ6S-2 strain, we cannot decide at present whether the strain’s phenotype of enhanced biofilm formation is caused by an increase in the number of matrix-producing cells or whether this subpopulation remains constant but individual cells of this subpopulation strongly overexpress the matrix genes. In the former case, 6S-2 RNA would be involved in the regulation of B. subtilis cell differentiation. Finally, relative to the wild type, we observed earlier spore formation for the NCIB 3610 Δ6S-2 strain, retarded spore formation of the double knockout strain and little change in the case of the Δ6S-1 strain (). The finding for the Δ6S-2 strain illustrates the tight interdigitation of biofilm formation and sporulation. Previously, a 6S-1 RNA knockout and 6S-1&2 double knockouts (but not a 6S-2 knockout) in the B. subtilis 168 laboratory strain background were reported to result in earlier sporulation [Citation24]. For the B. subtilis 168 Δ6S-1 strain, this could be ascribed to an earlier onset of sporulation without affecting the time elapsing between initiation and completion of spore formation [Citation24]. We do not know yet if earlier spore formation observed for our NCIB 3610 Δ6S-2 strain is due to an earlier onset of sporulation or faster completion of spore formation. The seemingly contradictive sporulation phenotypes of 6S RNA deletion strains in the B. subtilis 168 versus NCIB 3610 background are not understood at present, but again emphasize the remarkable differences between B. subtilis laboratory and wild type-like strains.
Material and methods
Bacterial strains, plasmids, media and growth conditions
General methods
All bacterial strains and plasmids used in this study are listed in . DNA cloning and transformation of E. coli DH5α cells were carried out according to standard techniques [Citation61]. E. coli cultures were grown in LB medium, supplemented with 100 μg/ml ampicillin for plasmid maintenance. DNA oligonucleotides for plasmid construction, sequencing and qRT-PCR are summarized in . All plasmid constructs were verified by DNA sequencing. B. subtilis cells were streaked from frozen glycerol stocks onto LB agar plates (supplemented with antibiotics if necessary) that were incubated overnight at 37°C. A single colony was used for inoculation of a liquid culture that was grown overnight at 37°C and under shaking. On the next day, fresh prewarmed LB medium (antibiotics added as indicated) was inoculated with 1/1000 volume of overnight culture; this preculture was grown to early exponential phase (0.3 to 0.5 OD600) and used to inoculate the main culture to a starting OD600 of 0.05. Depending on the strain, antibiotics were added to final concentrations of 10 μg/ml in the case of chloramphenicol and kanamycin, and 100 μg/ml in the case of spectinomycin.
Table 2. Oligonucleotides used in this study
B. subtilis growth measurements
B. subtilis precultures (prepared as described above) grown to an OD600 of 0.3 to 0.5 were diluted with fresh prewarmed LB medium (20 ml in 100 ml Erlenmeyer flasks, without antibiotics) to a starting OD600 of 0.05. Growth was monitored in a TECAN Saphire 2 Platereader in 96-well microtiter plates (Greiner Bioscience, flat bottom, transparent, with plastic cover) at high orbital shaking and at the specified temperature. For the testing of different stress conditions, the preculture was diluted with fresh prewarmed LB medium to an OD600 of 0.05, and 1-ml aliquots thereof were centrifuged at low speed, the supernatant was discarded and the cell pellet was resuspended in either 1 ml prewarmed LB supplemented with 50 mM H2O2, LB adjusted to pH 9.5, or in 1 ml prewarmed MSgg minimal medium [5 mM potassium phosphate (pH 7.0), 100 mM 3-(N-morpholino)propane-sulphonic acid (pH 7.0), 2 mM MgCl2, 700 μm CaCl2, 50 μM MnCl2, 100 μM FeCl3, 1 μM ZnCl2, 2 μM thiamine, 0.5% glycerol, 0.5% glutamate] [Citation32,Citation44] supplemented with 0.8 M NaCl. The raw data (Excel sheets) of the plate reader measurements were further converted to growth curve illustrations using GraphPad Prism 8.
Construction of 6S RNA deletion strains
To generate derivatives of B. subtilis NCIB 3610, which is poorly susceptible to transformation, that lack one or both 6S RNAs, we used a thermosensitive vector (pMAD) for efficient allelic replacement [Citation40]. Plasmids and primers are listed in . Successful gene deletion was confirmed by DNA sequencing of PCR fragments derived from the 6S RNA loci and by Northern blot analyses. For more details, see Results section.
6S RNA complementation strains
Complementation strains were constructed to demonstrate the 6S RNA deletion as the cause of phenotypes. Deleted 6S RNA genes were reintroduced at the amyE locus of B. subtilis in two steps. In the first step, an integrative plasmid, encoding the bsrA (6S-1 RNA) or bsrB (6S-2 RNA) gene flanked by amyE sequences (522 and 650 bp) and a chloramphenicol acetyltransferase resistance cassette in opposite orientation, was integrated into the genome of the naturally competent NCIB 3610 comIQCitation12L strain (strain DK1042 [Citation41]). In the second step, chromosomal DNA was extracted from comIQCitation12L transformants and used to transfer the genotypes into the NCIB 3610 wild type and its 6S RNA knockout derivative strains. Transformation in the two steps was carried out according to the following procedure: 7 ml LB preculture inoculated with a single colony of the recipient strain was grown overnight at 30°C using a 100-ml Erlenmeyer flask. Then, 10 ml of modified competence medium (MCM; [Citation62]) in a 250-ml baffled Erlenmeyer flask were inoculated with overnight culture to an OD600 of 0.08, followed by incubation at 37°C under shaking at 200 rpm in a water bath shaker (Aquatron) until an OD600 of 1.4 was reached; 1 ml of the culture was transferred to a Sarstedt 13-ml PP tube and transformed with either linearized plasmid DNA (1.5 μg; first step) or genomic DNA (500 ng; second step) for 2 h at 37°C under shaking (200 rpm in a warm air shaker), followed by plating (200 μl directly and cell pellet from the remaining 800 μl resuspended in 200 µl) on selective antibiotic agar plates and incubation overnight at 37°C. The double crossover of DNA sequences at the amyE locus was verified by screening for amylase degradation activity [Citation63] on starch agar plates (7.5 g/l Difco Nutrient Broth, 5 g/l starch, 15 g/l agar).
Extraction of cellular RNA and Northern Blotting
B. subtilis precultures were used to inoculate 150–200 ml prewarmed LB medium to a starting OD600 of 0.05, followed by incubation at 37°C or 20°C and 220 rpm in the water bath shaker. In , samples were harvested at different time points as indicated; in and S1, either at an OD600 of 0.3 to 0.5 for exponential phase, at 3.5 OD600 for transition phase or after 24 h for stationary phase. In each case, cell material corresponding to 1 ml cell suspension with an OD600 of 10 was collected by centrifugation for 10 min at 8230 x g and 4°C; cell pellets were frozen in liquid nitrogen and stored at −80°C. Extraction of total RNA was carried out as described [Citation64] using the RNA isolation Method 1 (three times with hot phenol). For Northern blotting of 6S-1 and 6S-2 RNA, 0.6 µg (in ) or 3 μg (in ) of total RNA were separated by 10% or 12% denaturing (8 M urea) polyacrylamide gel electrophoresis (PAGE) following the protocol described in [Citation65] for intermediate-sized RNAs. Digoxigenin-labelled antisense T7 transcripts of both 6S RNAs (190 nt covering mature 6S-1 RNA, 211 nt covering 6S-2 RNA) and 5S rRNA (loading control, covering nt 1–115 of 5S rRNA) were used as probes [Citation65]. Full-length T7 sense transcripts of 6S RNAs served as markers.
Biofilm formation assay
Five microlitres of a B. subtilis overnight culture were spotted on MSgg agar plates (1.5% Bacto agar, dried overnight at 30°C) supplemented with Congo Red (40 μg/ml, Sigma-Aldrich) and Coomassie Brilliant Blue R250 (20 μg/ml, Roth) [Citation66]. After drops had dried, the plates were incubated for several days at the indicated temperatures. Colony morphology was documented using a gel documentation instrument (Dark Hood DH-50 transilluminator with Gerix 1000 CCD camera; biostep) for black and white images and additionally with a digital camera (QV-5700, Casio) for coloured images. Pictures were processed utilizing the ImageJ software [Citation67]. For each strain, at least three independent biological replicates were analysed.
Quantitative RT-PCR (qRT-PCR)
RNA was collected from bacterial cultures at different growth stages as described above. Total RNA was treated with DNase I (Ambion) and RNA quality was checked by 6% denaturating PAGE and ethidium bromide staining. Equal amounts of total RNA (50 ng/reaction) were subjected to a one-step qRT-PCR reaction in a volume of 20 μl (Luna Universal one-step Kit, NEB) according to the protocol of the manufacturer (for primers, see ). Analysis was performed with a QuantStudio3 instrument (Applied Biosystems) using the standard run mode. Individual expression profiles of tested mRNAs were normalized to the 5S rRNA control. Relative expression levels were determined with the comparative CT method [Citation68]. From the raw data collected by the QuantStudio3 instrument (stored in Excel files), ∆CT and ∆∆CT values were calculated; results were illustrated as column graphs using GraphPad Prism 8. For more details, see legend to Fig. S1.
Motility/swarming assay
To study the influence of 6S-2 RNA on the motility of B. subtilis, bacterial growth was investigated on swarm plates. Single colonies of NCIB 3610 derivative strains WT amyE::cat, Δ6S-2 amyE::cat and Δ6S-2 amyE::6S-2:cat (see ) grown on LB plates (1.5% agar, 5 μg/ml chloramphenicol [CM]) overnight at 37°C were used to inoculate 3 ml LB medium containing 5 μg/ml CM, followed by growth at 37°C overnight in a warm air shaker. Then, 20 μl of overnight culture were introduced into 20 ml of LB medium (in 100-ml unbaffled Erlenmeyer flasks) containing 5 μg/ml CM prewarmed to 37°C (1:1000 dilution), followed by growth at 37°C in a water bath shaker (Aquatron, 220 rpm) to an OD600 of 0.3 to 0.6. This preculture was used to inoculate 50 ml LB medium (in a 250-ml baffled Erlenmeyer flask) to a starting OD600 of 0.05; cultures were grown at 37°C under shaking (220 rpm in the water bath shaker). At an OD600 of 2.0, 2 μl of cell suspension were spotted onto a 0.7% TG swarming plate [peptone 1% (w/v), meat extract 1% (w/v), NaCl 0.5% (w/v), 55 mM glucose, 0.3 mM 2,3,5 tri-phenyl tetrazolium chloride (Sigma-Aldrich Merck), 0.7% (w/v) agar] [Citation69]. TG plates were freshly poured, air-dried for 20 min at room temperature without lid and then stored with cover at room temperature for use on the next day. After spotting, plates were incubated at 28°C and the distance from the spot centre to the outer swarm front (= radius) was documented every hour with the aforementioned documentation equipment (Dark Hood DH-50 + Gerix 1000, adjusting the lens focus manually to f/4 iris, 12x zoom, 7/20 focus, 0.04x illumination). For improved recognizability of the swarm front, a radius line was drawn manually on the plate bottom which was measured digitally using the ImageJ 1.51p software; the graph shown in was generated with GraphPad Prism 8.
Sporulation assay
To quantify spores in a liquid culture, we exploited the heat-resistant nature of B. subtilis spores to distinguish them from heat-sensitive vegetative cells [Citation24,Citation70]. Preculture preparation was done as described above under ‘Bacterial strains, plasmids, media and growth conditions, General methods’. Cells of the preculture grown to early exponential phase (0.3 to 0.5 OD600) were centrifuged and the cell pellet was resuspended in DSM medium after removal of the LB medium. Main cultures were then inoculated to an OD600 of 0.05 in 100 ml prewarmed DSM medium in 1-l baffled Erlenmeyer flasks (DSM medium, per 1 l: 8 g Difco nutrient broth, 1 g KCl, 1 ml of 1 M MgSO4, 1 ml of 10 mM MnCl2; after autoclaving, 0.5 ml 1 M CaCl2 and 100 µl FeSO4 [100 µM] were added). Cultures were agitated in a water bath shaker at 37°C, 220 rpm. After 8 h of incubation, 1-ml culture samples of each strain were withdrawn to make serial ten-fold dilutions in Gibco PBS buffer (pH 7.4; Thermo Fisher Scientific). 100 µl of each dilution sample were directly plated onto LB agar plates (quantification of all types of viable cells). The remaining 900 µl were heated to 80°C for 20 min and again 100 µl of each dilution sample were plated onto LB agar plates (quantification of heat-resistant spores only). After incubation of plates for 24 h at 37°C, colonies were counted (using ImageJ) on plates with streaked dilutions that yielded 10 to 700 distinguishable colonies per plate. To determine the fraction of spores within the cultures, the ratio of heated to non-heated colony numbers in the same volume of cell suspension was calculated. The presented data are based on three independent biological replicates.
Supplemental Material
Download MS Word (3.2 MB)Acknowledgments
We like to thank Julia Renz for her valuable contributions to the construction of the 6S RNA deletion strains and Jana C. Wiegard for fruitful discussions. We are grateful to Patricia Bedrunka and Gert Bange for providing the NCIB 3610 comIQ12L strain.
Disclosure statement
No potential conflicts of interest were disclosed.
Supplementary material
Supplemental data for this article can be accessed here.
Additional information
Funding
References
- Wehner S, Damm K, Hartmann RK, et al. Dissemination of 6S RNA among bacteria. RNA Biol. 2014;11:1467–1478.
- Barrick JE, Sudarsan N, Weinberg Z, et al. 6S RNA is a widespread regulator of eubacterial RNA polymerase that resembles an open promoter. RNA. 2005;11:774–784.
- Chen J, Wassarman KM, Feng S, et al. 6S RNA mimics B-form DNA to regulate Escherichia coli RNA polymerase. Mol Cell. 2017;68:388–397.
- Köhler K, Duchardt-Ferner E, Lechner M, et al. Structural and mechanistic characterization of 6S RNA from the hyperthermophilic bacterium Aquifex aeolicus. Biochimie. 2015;117:72–86.
- Willkomm DK, Minnerup J, Hüttenhofer A, et al. Experimental RNomics in Aquifex aeolicus: identification of small non-coding RNAs and the putative 6S RNA homolog. Nucleic Acids Res. 2005;33:1949–1960.
- Nickel AI, Wäber NB, Gößringer M, et al. Minimal and RNA-free RNase P in Aquifex aeolicus. Proc Natl Acad Sci USA. 2017;114:11121–11126.
- Trotochaud AE, Wassarman KM. 6S RNA function enhances long-term cell survival. J Bacteriol. 2004;186:4978–4985.
- Trotochaud AE, Wassarman KM. 6S RNA regulation of pspF transcription leads to altered cell survival at high pH. J Bacteriol. 2006;188:3936–3943.
- Cavanagh AT, Chandrangsu P, Wassarman KM. 6S RNA regulation of relA alters ppGpp levels in early stationary phase. Microbiology. 2010;156:3791–3800.
- Cavanagh AT, Wassarman KM. 6S RNA, a global regulator of transcription in Escherichia coli, Bacillus subtilis, and beyond. Annu Rev Microbiol. 2014;68:45–60.
- Steuten B, Hoch PG, Damm K, et al. Regulation of transcription by 6S RNAs: insights from the Escherichia coli and Bacillus subtilis model systems. RNA Biol. 2014;11:508–521.
- Madhugiri R, Pessi G, Voss B, et al. Small RNAs of the Bradyrhizobium/Rhodopseudomonas lineage and their analysis. RNA Biol. 2012;9:47–58.
- Elkina D, Weber L, Lechner M, et al. 6S RNA in Rhodobacter sphaeroides: 6S RNA and pRNA transcript levels peak in late exponential phase and gene deletion causes a high salt stress phenotype. RNABiol. 2017;14:1627–1637.
- Axmann IM, Holtzendorff J, Voss B, et al. Two distinct types of 6S RNA in Prochlorococcus. Gene. 2007;406:69–78.
- Heilmann B, Hakkila K, Georg J, et al. 6S RNA plays a role in recovery from nitrogen depletion in Synechocystis sp. PCC6803. BMC Microbiol. 2017;17:229.
- Ren J, Sang Y, Qin R, et al. 6S RNA is involved in acid resistance and invasion of epithelial cells in Salmonella enterica serovar Typhimurium. Future Micobiol. 2017;12:1045–1057.
- Faucher SP, Friedlander G, Livny J, et al. Legionella pneumophila 6S RNA optimizes intracellular multiplication. Proc Natl Acad Sci USA. 2010;107:7533–7538.
- Weissenmayer BA, Prendergast JG, Lohan AJ, et al. Sequencing illustrates the transcriptional response of Legionella pneumophila during infection and identifies seventy novel small non-coding RNAs. PLoS One. 2011;6(3):e17570.
- Beckmann BM, Burenina OY, Hoch PG, et al. In vivo and in vitro analysis of 6S RNA-templated short transcripts in Bacillus subtilis. RNA Biol. 2011;8:839–849.
- Beckmann BM, Hoch PG, Marz M, et al. A pRNA-induced structural rearrangement triggers 6S-1 RNA release from RNA polymerase in Bacillus subtilis. EMBO J. 2012; 31:1727–1738
- Burenina OY, Hoch PG, Damm K, et al. Mechanistic comparison of Bacillus subtilis 6S-1 and 6S-2 RNAs – commonalities and differences. RNA. 2014;20:348–359.
- Hoch PG, Schlereth J, Lechner M, et al. Bacillus subtilis 6S-2 RNA serves as a template for short transcripts in vivo. RNA. 2016;22:614–622.
- Wassarman KM, Saecker RM. Synthesis-mediated release of a small RNA inhibitor of RNA polymerase. Science. 2006;314(5805):1601–1603.
- Cavanagh AT, Wassarman KM. 6S-1 RNA function leads to a delay in sporulation in Bacillus subtilis. J Bacteriol. 2013;195:2079–2086.
- Trotochaud AE, Wassarman KM. A highly conserved 6S RNA structure is required for regulation of transcription. Nat Struct Mol Biol. 2005;12:313–319.
- Ando Y, Asari S, Suzuma S, et al. Expression of a small RNA, BS203 RNA, from the yocI-yocJ intergenic region of Bacillus subtilis genome. FEMS Microbiol Lett. 2002;207:29–33.
- Cabrera-Ostertag IJ, Cavanagh AT, Wassarman KM. Initiating nucleotide identity determines efficiency of RNA synthesis from 6S RNA templates in Bacillus subtilis but not Escherichia coli. Nucleic Acids Res. 2013;41:7501–7511.
- Hoch PG, Burenina OY, Weber MH, et al. Phenotypic characterization and complementation analysis of Bacillus subtilis 6S RNA single and double deletion mutants. Biochimie. 2015;117:87–99.
- Wassarman KM, Storz G. 6S RNA regulates E. coli RNA polymerase activity. Cell. 2000;101:613–623.
- Cavanagh AT, Sperger JM, Wassarman, KM. Regulation of 6S RNA by pRNA synthesis is required for efficient recovery from stationary phase in E. coli and B. subtilis. Nucleic Acids Res. 2012;40:2234–2246.
- Zeigler DR, Pragai Z, Rodriguez S, et al. The origins of 168, W23, and other Bacillus subtilis legacy strains. J Bacteriol. 2008;190:6983–6995.
- Branda SS, Gonzalez-Pastor JE, Ben-Yehuda S, et al. Fruiting body formation by Bacillus subtilis. Proc Natl Acad Sci USA. 2001;98:11621–11626.
- Brown CT, Fishwick LK, Chokshi BM, et al. Whole genome sequencing and phenotypic analysis of Bacillus subtilis mutants following evolution under conditions of relaxed selection for sporulation. Appl Environ Microbiol. 2011;77:6867–6877.
- Kearns DB, Chu F, Rudner R, et al. Genes governing swarming in Bacillus subtilis and evidence for a phase variation mechanism controlling surface motility. Mol Microbiol. 2004;52:357–369.
- McLoon AL, Guttenplan SB, Kearns DB, et al. Tracing the domestication of a biofilm-forming bacterium. J Bacteriol. 2011;193:2027–2034.
- Nakano MM, Corbell N, Besson J, et al. Isolation and characterization of sfp: a gene that functions in the production of the lipo-peptide biosurfactant, surfactin, in Bacillus subtilis. Mol Gen Genet. 1992;232:313–321.
- Stannek L, Gunka K, Care RA, et al. Factors that mediate and prevent degradation of the inactive and unstable GudB protein in Bacillus subtilis. Front Microbiol. 2015;5:758.
- Albertini AM, Galizzi A. The sequence of the trp operon of Bacillus subtilis 168 (trpC2) revisited. Microbiology. 1999;145:3319–3320.
- Yi H, Chun J, Cha CJ. Genomic insights into the taxonomic status of the three subspecies of Bacillus subtilis. Syst Appl Microbiol. 2014;37:95–99.
- Arnaud M, Chastanet A, Debarbouille M. New vector for efficient allelic replacement in naturally nontransformable, low-GC-content, gram-positive bacteria. Appl Environ Microbiol. 2004;70:6887–6891.
- Nye TM, Schroeder JW, Kearns DB, et al. Complete genome sequence of undomesticated Bacillus subtilis strain NCIB 3610. Genome Announc. 2017;5(20):e00364–17.
- Hanahan D. Studies on transformation of Escherichia coli with plasmids. J Mol Biol. 1983;166:557–580.
- Youngman P, Perkins JB, Losick R. Construction of a cloning site near one end of Tn917 into which foreign DNA may be inserted without affecting transposition in Bacillus subtilis or expression of the transposon-borne erm gene. Plasmid. 1984;12:1–9.
- Freese E, Heinye JE, Galliers EM. Partial purine deprivation causes sporulation of Bacillus subtilis in the presence of excess ammonia, glucose and phosphate. J Gen Microbiol. 1979;54:49–79.
- Vlamakis H, Chai Y, Beauregard P, et al. Sticking together: building a biofilm the Bacillus sutbilis way. Nat Rev Microbiol. 2013;11:157–168.
- Jones CJ, Wozniak DJ. Congo red stain identifies matrix overproduction and is an indirect measurement for c-di-GMP in many species of bacteria. Methods Mol Biol. 2017;1657:147–156.
- Branda SS, Chu F, Kearns DB, et al. A major protein component of the Bacillus subtilis biofilm matrix. Mol Microbiol. 2006;59:1229–1238.
- Ostrowski A, Mehert A, Prescott A, et al. YuaB functions synergistically with the exopolysaccharide and TasA amyloid fibers to allow biofilm formation by Bacillus subtilis. J Bacteriol. 2011;193:4821–4831.
- Beranovà J, Jemioła-Rzemińska M, Elhottova D, et al. Metabolic control of the membrane fluidity in Bacillus subtilis during cold adaptation. Biochim Biophys Acta. 2008;1778:445–453.
- Hecker M, Pané-Farré J, Völker U. SigB-dependent general stress response in Bacillus subtilis and related gram-positive bacteria. Annu Rev Microbiol. 2007;61:215–236.
- Brigulla M, Hoffmann T, Krisp A, et al. Chill induction of the SigB-dependent general stress response in Bacillus subtilis and its contribution to low-temperature adaptation. J Bacteriol. 2003;185:4305–4314.
- Méndez MB, Orsaria LM, Philippe V, et al. Novel roles of the master transcription factors Spo0A and sigmaB for survival and sporulation of Bacillus subtilis at low growth temperature. J Bacteriol. 2004;186:989–1000.
- Bartolini M, Cogliati S, Vileta D, et al. Regulation of biofilm aging and dispersal in Bacillus subtilis by the alternative sigma factor SigB. J Bacteriol. 2018;201:e00473–18.
- Choi S-K, Saier MH. Transcriptional Regulation of the rsbV Promoter Controlling Stress Responses to Ethanol, Carbon Limitation, and Phosphorous Limitation in Bacillus subtilis. Int J Microbiol. 2010;2010:263410. doi:10.1155/2010/263410.
- Widderich N, Rodrigues CD, Commichau FM, et al. Salt-sensitivity of σH and Spo0A prevents sporulation of Bacillus subtilis at high osmolarity avoiding death during cellular differentiation. Mol Microbiol. 2016;100:108–124.
- Fujita M, Gonzalez-Pastor JE, Losick R. High- and low-threshold genes in the Spo0A regulon of Bacillus subtilis. J Bacteriol. 2005;187:1357–1368.
- Molle V, Fujita M, Jensen ST, et al. The Spo0A regulon of Bacillus subtilis. Mol Microbiol. 2003;50:1683–1701.
- Dragoš A, Kiesewalter H, Martin M, et al. Division of labor during biofilm matrix production. Curr Biol. 2018;28:1903–1913.
- Lopez D, Vlamakis H, Kolter R. Generation of multiple cell types in Bacillus subtilis. FEMS Microbiol Rev. 2009;33:152–163.
- Mielich-Suss B, Lopez D. Molecular mechanisms involved in Bacillus subtilis biofilm formation. Environ Microbiol. 2015;17:555–565.
- Sambrook J, Russell DW. Molecular cloning – A laboratory manual. Cold Spring Harbor (New York): Cold Spring Harbor Laboratory Press; 2001.
- Zafra O, Lamprecht-Grandio M, de Figueras CG, et al. Extracellular DNA release by undomesticated Bacillus subtilis is regulated by early competence. PLoS One. 2012;7(11):e48716.
- Cutting SM, Vander Horn PB. Genetic analysis. In molecular biological methods for Bacillus. Chichester, UK: John Wiley and Sons Ltd; 1990. p. 27–74.
- Damm K, Bach S, Müller KM, et al. Impact of RNA isolation protocols on RNA detection by Northern Blotting. Methods Mol Biol. 2015;1296:29–38.
- Damm K, Bach S, Müller KM, et al. Improved Northern blot detection of small RNAs using EDC crosslinking and DNA/LNA probes. Methods Mol Biol. 2015;1296:41–51.
- Bedrunka P, Graumann PL. New functions and subcellular localization patterns of c-di-GMA components (GGDEF domain proteins) in B. subtilis. Front Microbiol. 2017;8:794.
- Schneider CA, Rasband WS, Eliceiri KW. NIH Image to ImageJ: 25 years of image analysis. Nat Methods. 2012;9:671–675.
- Livak KJ, Schmittgen TD. Analysis of relative gene expression data using real-time quantitative PCR and the 2(-Delta Delta C(T)) method. Methods. 2001;25:402–408.
- Bordner R, Winter JA, Scarpino P Microbiological methods for monitoring the environment: water and wastes. EPA-600/8-78-017. Cincinnati (OH): Environmental Monitoring and Support Laboratory, US Environmental Protection Agency, Office of Research and Development; 1978.
- Nicholson WL, Setlow P. Sporulation, germination and outgrowth. In: Harwood CR, Cuutting SM, editors. Molecular biological methods for Bacillus. Chichester (UK): John Wiley & Sons; 1990. p. 391–450.