ABSTRACT
The zinc finger CCHC-type (ZCCHC) superfamily proteins, characterized with the consensus sequence C-X2-C-X4-H-X4-C, are accepted to have high-affinity binding to single-stranded nucleic acids, especially single-stranded RNAs. In human beings 25 ZCCHC proteins have been annotated in the HGNC database. Of interest is that among the family, most members are involved in the multiple steps of RNA metabolism. In this review, we focus on the diverged roles of human ZCCHC proteins on RNA transcription, biogenesis, splicing, as well as translation and degradation.
1. Introduction
The zinc finger CCHC-type (ZCCHC) superfamily protein contains at least one consensus ZnF-like domain with C-X2-C-X4-H-X4-C sequence (where X is any amino acid, C is cysteine and H is histidine). This motif is also called as zinc-knuckle (see ) which are seen in all ZCCHC proteins (see ) [Citation1]. That ZCCHC domains are generally composed of two short β-strands connected by a zinc knuckle followed by a short helix. First observed in the transcription factor IIIA (TFIIIA) from Xenopus oocytes Citation2, ZCCHC domains have been regarded as a typical nucleic acid-recognizing motifs [Citation3]. It is suggested that this type of zinc finger has high-affinity binding to single-stranded nucleic acids [Citation1]. Until recently a total of 25 ZCCHC proteins have been identified in this superfamily, from ZCCHC1 to ZCCHC25. Each member plays diverse roles in both cellular physiological function and disease aetiology (see , their characteristics and cellular functions are listed). Most of them are RNA-binding proteins (RBPs), while some mediate DNA binding and protein–protein interactions through their ZCCHC domains, such as ZCCHC3, 8, 9, 10, 11, 13, 22 [Citation4–10].
Table 1. The CCHC sequences and structure of zinc finger CCHC-type superfamily
Table 2. Main characterization and cellular functions of zinc finger CCHC-type superfamily
Figure 1. The CCHC/zinc-knuckle motif in the CCHC-type zinc finger proteins. C and H refers to cysteine and histidine, respectively. X is any amino acid, whereas there is still regularity to conform to. The conservatively substituted residues have been mentioned in the figure. That conserved array has high affinity to Zn+ and participates directly in nucleic acid recognition and binding, especially the single-stranded nucleic acids
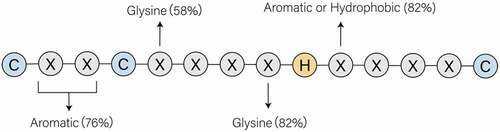
Several members of the superfamily are correlated with diverse diseases. For examples, ZCCHC2 regulates retinoblastoma tumorigenesis by inhibiting the activity of c-Myc [Citation11]; ZCCHC4 associates with the aetiology of attention deficit hyperactivity disorder (ADHD) [Citation12]; ZCCHC7 disrupted by sequences 3ʹ of FRG1B and LOC1499503 is potential genetic targets in acute lymphoblastic leukaemia [Citation13]; ZCCHC8 is identified as a fusion partner of ROS1 in congenital glioblastoma multiforme [Citation14] and a novel cause of human short telomere syndromes–familial pulmonary fibrosis [Citation15]; ZCCHC12 is referred to as a novel oncogene of papillary thyroid carcinoma [Citation16,Citation17]; ZCCHC17 regulates synaptic gene expression in Alzheimer’s disease [Citation18]; abnormal expression of ZCCHC22 is a potential onset in myotonic dystrophy type 2 (DM2) [Citation19]. ZCCHC1, ZCCHC5, ZCCHC9, ZCCHC13, ZCCHC20 and ZCCHC21 are related to various solid tumours, such as breast, lung, liver, colorectal and renal cancers [Citation20–27].
More importantly, increasing literatures have implicated the ZCCHC superfamily in the regulation of RNA metabolism. RNA metabolism includes four distinct steps. First, selected segments of the genome are transcribed into primary RNAs by an RNA polymerase. Second, these primary transcripts are processed to be functionally mature RNAs. Splicing, the most striking event in RNA processing, occurs to remove introns and join exons together in eukaryotic cells. Other processing events include protection of both ends of the transcript. Third, these specific RNAs may encode proteins (coding RNAs) or play structural or catalytic roles (non-coding RNAs). Finally, the RNAs are degraded after finishing their functions. All these steps are essential to sustain cellular functions. Despite the growing published studies on the ZCCHC superfamily, many key questions remain to be answered. Based on the existing progresses, this review summarizes and discusses the emerging concept of ZCCHC superfamily as vital molecules in the regulation of RNA metabolism including transcription, splicing, translation and degradation.
2. Transcription
RNA transcription is a process in which genetic information is transformed from DNA to RNA, including the synthesis of messenger RNA and non-coding RNA (transfer RNA, ribosomal RNA, etc.). This process follows the base pairing principle and consists of three stages: initiation, chain elongation, and termination. By the action of an RNA polymerase, segments of DNA are transcribed into RNA under the strict regulation. Transcriptional co-activation/co-suppression occurs via multi-protein complexes that dock on transcription factors and recruit chromatin-modifying enzymes. Several ZCCHC proteins may function as a transcriptional activator or suppressor and then influence the progress of RNA transcription. Some are well illustrated, whereas others remain unfathomable so far.
2.1 ZCCHC12/Sizn1
ZCCHC12/Sizn1, identified as a novel modulator that functions as a transcriptional co-activator of bone morphogenic protein (BMP) signalling, has an impact on the progress of transcription initiation [Citation28]. In the nucleus, the Smad complex can directly bind to DNA and then recruit either transcriptional co-activators or co-repressors to enhance or repress the transcription, respectively, [Citation29]. CBP, a chromatin-modifying enzyme for acetylation, interacts with components of the basal transcription machinery and various different DNA-binding factors so as to activate the transcription [Citation30,Citation31]. Although ZCCHC12 does not directly interact DNA or RNA in vitro, ZCCHC12 come up close to DNA through its interaction with Smad1 and CBP in vivo, thus positively regulating Smad1-mediated transcription [Citation28]. The N-terminus of ZCCHC12 interacts with CBP, while its C-terminus associates with Smad1 [Citation32]. Unlike other ubiquitously expressed transcriptional co-regulators, ZCCHC12 shows restricted spatial and temporal expression patterns [Citation28]. ZCCHC12 localizes to promyelocytic leukaemia protein nuclear bodies (PML-NBs) [Citation32], which are large ring-shaped protein complexes involved in diverse nuclear functions including transcription [Citation33,Citation34], through two SIMs (SUMO interaction motif) and the MA homologous domain. The necessity of SIMs in ZCCHC12 for full co-activation of BMP signalling pathway is verified by the SBEx4-luciferase reporter assay [Citation32]. ZCCHC12 also activates the transcriptional activities mediated by three important transcription factors, c-Jun, AP-1 and CREB, for which CBP is a co–activator [Citation35–37].
Recent studies indicate the important impact of ZCCHC12 on the oncogenesis of papillary thyroid cancer [Citation17] and specific gene expression of basal forebrain cholinergic neuron [Citation28]. ZCCHC12 is also a potential molecular marker of Cajal–Retzius cells, which is worth investigating in the development and pathogenesis of mental retardation [Citation38], and a potential endometrial receptivity-related marker as well [Citation39].
2.2 ZCCHC22/CNBP
ZCCHC22/CNBP, containing seven Zn knuckles and an Arg-Gly-rich box, regulates gene expressions majorly at the transcriptional levels. It acts as either a positive or negative transcription factor. Binding to the CT-rich element, ZCCHC22 enhances the transcription of a proto-oncogene, c-Myc [Citation40]. Further studies show that ZCCHC22 binds the G-rich sequence in the nuclease hypersensitivity element (NHE) III of c-Myc promoter to promote the formation of parallel G-quadruplexes (G4), which are folded from guanine-rich DNA strands to function as cis-regulatory elements surrounding transcription start sites [Citation41,Citation42]. In contrast, a different conclusion is drawn that in vitro ZCCHC22 unfolds the G4 in the NHE III of c-MYC promoter to stimulate c-Myc transcription through relieving the suppression caused by G4 formation [Citation43,Citation44]. More interestingly, by stabilizing G4 structure, ZCCHC22 may inhibit heterogeneous ribonucleoprotein K (hnRNPK) gene transcription [Citation45]. Again, on the contrary, by unfolding parallel G4 structure, it enhances c-KIT, KRAS, PDGF, RET and VEGF transcriptions while down-regulates the transcription of NOGGIN/noggin3 [Citation43]. Additionally, ZCCHC22 has been identified as a transcription regulator of β-myosin heavy chain (β-MHC), components of the Wnt signalling pathway [Citation46,Citation47], and inflammatory genes such as IL-6 and IL-12 [Citation48,Citation49].
Moreover, at the post-transcriptional level ZCCHC22 cooperates with a long non-coding RNA, LncRNA-assisted stabilization of transcripts (LAST), to stabilize the Cyclin D1 (CCND1) messenger RNA via binding to 5ʹ untranslated region of CCND1 mRNA, and then exerts a protective effect against the nuclease degradation [Citation50].
2.3 ZCCHC-containing factors have an impact on rRNA biogenesis
Ribosomal RNA (rRNA) processing starts in the nucleolus. Combined with other components, rRNAs form a nucleoprotein called a ribosome serves as the place for protein synthesis. ZCCHC17, also named pNO40, acts as a negative regulator of rDNA transcription. When ZCCHC17 interacts with the upstream binding factor (UBF), whose binding to the rDNA promoter is reduced [Citation51]. Human ZCCHC4 has been reported as a novel m6A methyltransferase that methylates human 28S rRNA [Citation52]. Moreover, a knockdown of ZCCHC9 that results in an overexpression of 21S pre-rRNA [Citation53].
2.4 Other ZCCHC-containing proteins that are also involved in RNA transcription
ZCCHC3 is considered as a positive regulator of RIG-I and MDA5 transcription [Citation54]. Overexpression of ZCCHC3 enhances the transcription efficiency of the poly(I:C)-induced downstream antiviral genes [Citation55]. By contrast, knockdown of ZCCHC3 in 293-TLR3 cells and HT1080 cells downregulates the transcriptions of IFNB1, ISG56 and CXCL10 genes [Citation56].
ZCCHC9 is proved to inhibit the transcription of NF-kappaB and SRE, in which manner MAPK signalling cascade is modulated [Citation6]. Overexpression of ZCCHC9 has a positive correlation with transcriptions of p‐JNK, Cyclin D1, and MMP7 and then facilitates the proliferation and invasion in human non‐small cell lung cancer [Citation26]. Moreover, ZCCHC10 cooperates with pared-like homeodomain 1 (PITX1) to repress the transcription of the human telomerase reverse transcriptase (hTERT) [Citation10]. Through changing telomere length, hTERT serves as a contributor to cancerous unlimited replication; thus, ZCCHC10 may have a function of tumour suppressor. Meanwhile, miR-410-3p promotes the NF-κB pathway activation by targeting ZCCHC10 [Citation57].
3. Splicing
Splicing, as a step of mRNA maturation, is a part of post-transcriptional regulation. Constitutive splicing refers to the process of removing introns and retaining exons, whereas alternative splicing (AS) is responsible for exon selection and isoform regulation [Citation58,Citation59]. Disregulation of splicing including exon skipping, intron retention, or alternative exon usage, etc., may produce deleterious transcripts and lead to RNA-related diseases. Apart from the functional classification, splicing can also be classified according to numbers of pre-mRNA targets. The traditional cis-splicing takes place in single-molecule mRNAs, whereas the less common trans-splicing procedure represents exon recombinations of multiple independent pre-mRNAs [Citation60]. No matter which form it happens to be, a spliceosome is assembled most of the time to identify splicing sites, which is generally believed to be constituted by five major small nuclear ribonucleoprotein particles (snRNPs) and 100 non-snRNP protein splicing factors (SFs) [Citation61,Citation62]. The whole process requires trans-acting proteins to conduct positive or negative regulation of splicing by binding to cis-regulatory elements that can be divided into four categories: exonic splicing enhancers (ESEs), exonic splicing silencers (ESSs), intronic splicing enhancers (ISEs) and intronic splicing silencers (ISSs) [Citation58,Citation63]. Those factors and their associated proteins, for example, SR proteins (serine/arginine-rich proteins), can be stored and assembled in subnuclear compartments called nuclear speckles, during which they undergo post-translational modifications, enabling their recruitment to trancriptional sites [Citation64,Citation65].
Several members of ZCCHC superfamily have different roles in splicing as illustrated in . Both ZCCHC17 and ZCCHC20 act like a shuttle cargo that either recruits splicing factors to splice sites or accompanies splicing-completed mRNAs export to cytoplasm. ZCCHC20, ZCCHC21 and ZCCHC25 are distinct alternative splicing (AS) regulators, participating in various physiological and pathological processes by altering functional protein isoform profiles. ZCCHC25 is also indispensable in spliceosome assembly and 3ʹ splice site recognition.
Figure 2. Four members of the ZCCHC family are associated with pre-mRNA splicing. Constitutive splicing is the process of removing intron, while alternative splicing takes part in the selection of exons. Together with other mRNAs, trans-splicing is performed. A spliceosome is assembled during the process. Splicing-associated proteins localize in some special nuclear compartments named nuclear speckles, meanwhile can be released into the nucleoplasm once activated. ZCCHC17/pNO40 is a ribosome protein located within the nucleolus, but it can shuttle to nuclear speckles and bind to splicing factor SRSF2 indirectly affecting the splicing process. ZCCHC21/RBM4 is a critical RNA binding protein (RBP) which decides the inclusion or omission of exons by interacting with the cis-regulatory elements. Apart from its role in alternative splicing, evidence shows that it can also regulate trans-splicing. ZCCHC20/SRSF7 is also RBP, and it acts to regulate constitutive and alternative splicing of various proteins on binding the exon/intron splicing enhancer (ESE/ISE) region of pre-mRNA. SRSF7 also mediates mature mRNA nuclear export by recruiting NXF1. ZCCHC25/SF1, however, participates in the pre-spliceosome assembly
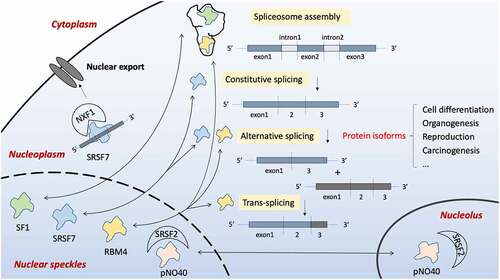
3.1 ZCCHC17/pNO40
ZCCHC17, also named as pNO40, is initially known as an SR-like protein pinin (pnn)-interacting protein. As a core component of 60S ribosomal subunit, it often resides in the nucleoli to negatively regulate rRNA biogenesis [Citation51]. It is highly conserved between human and mouse, and has its highest expressions in brain, heart and testis [Citation66,Citation67]. ZCCHC17 has an S1 RNA binding region on its N-terminus, a CCHC-type zinc finger motif in the middle, and clusters of basic amino acid representing potential nucleolar targeting signal on its C-terminus [Citation66]. Moreover, the N-terminus also mediates protein–protein interaction, as ZCCHC17 is proved to interact with SR-family splicing factors including Pnn and recruit them from nuclear speckles to nucleoli, contributing to rRNA splicing regulation [Citation66]. Aberrant expression of ZCCHC17 also influences overall mRNA quality control. For example, pNO40 overexpression reduces pre-mRNA splicing, ploy(A)+ RNA nuclear export, and RNAP II activity [Citation65].
3.2 ZCCHC20/SRSF7
ZCCHC20, also named as SRSF7 or 9G8, is a member of SR family serine–arginine-rich splicing factors (SRSF). It is highly conserved across species and expressed most abundantly in bone marrow and lymph node [Citation67]. Sharing characteristics with other members of SRSF family, ZCCHC20 possesses an N-terminal RNA recognition motif (RRM), a C-terminal repeated serine-arginine dipeptide domain (RS), and a CCHC-type zinc-finger motif in between [Citation68]. By binding to the pre-mRNA exon or intron splicing enhancers (ESE, ISE) through RRM, ZCCHC20 promotes the inclusion or exclusion of exons, respectively, highlighting its regulative role in both constitutive and alternative splicing [Citation69]. For examples, by binding to splicing enhancers, ZCCHC20 indeed regulates constitutive and alternative splicing of various transcripts including CD44, BRCA1 and Tau [Citation70,Citation71]. CCHC-type zinc-finger motif of ZCCHC20 increases the binding specificity[Citation70], while its RS domain mediates protein–protein and/or RNA–protein interaction [Citation72]. It undergoes phosphorylation–dephosphorylation cycle, which is crucial for the dynamic splicing process including the spliceosome assembly, splicing catalysis and termination [Citation72].
In some circumstances, ZCCHC20 acts competitively with other SRSFs in AS regulation of CD44 [Citation73]. ZCCHC20 regulates alternative splicing of Fas/CD95 to balance the ratio of pro/anti-apoptotic isoform, and knockdown of ZCCHC20 inhibits proliferation and induces apoptosis in multiple cancer cell lines [Citation74]. Interestingly, its zinc-containing motif is antagonized by iron, so as to be regulated by local iron homoeostasis [Citation74], demonstrating an unique functional regulation pathway of CCHC-type zinc-finger superfamily. In addition to its role in AS-dependent exon selection, ZCCHC20 also antagonizes other SRSFs in splicing-independent alternative polyadenylation (APA) [Citation74]. Depletion of ZCCHC20 results in more inclusion of exons and longer 3ʹUTR mRNA isoforms [Citation75].
ZCCHC20 also participates in other downstream events including mRNA export, nonsense-mediated RNA decay and translation [Citation75,Citation76]. Splicing-completed mRNA bound by ZCCHC20 can recruit an adaptor of nuclear transporter 1 (NXF1) facilitating mRNA export from nucleus to cytoplasm [Citation75]. Interestingly, the hypophosphorylation state of ZCCHC20 RS domain is requisite for its binding to NXF1, suggesting that ZCCHC20 carries primarily mature, splicing-completed mRNAs to the cytoplasm [Citation77]. Therefore, ZCCHC20 indeed serves as a shuttle cargo that links mRNA splicing and APA to mRNA export. Moreover, ZCCHC20 also binds intron-derived non-coding RNAs (ncRNAs) and helps them export to cytoplasm [Citation69]. However it turns out that ZCCHC20 binds to a much lower extent to intergenic long non-coding RNA (lncRNA) exons than protein-coding exons [Citation78].
ZCCHC20 has established its role in RNA metabolism and is crucial for common physiological processes. It is actually responsible for yielding juvenile-specific splicing isoforms in multiple organs examined in C57BL/6 mice, which is indispensable in deciding proper anabolic state for juveniles [Citation79]. Furthermore, dysregulation of ZCCHC20 leads to carcinogenesis. ZCCHC20 is overexpressed in lung and colon cancer tissues [Citation80]. Single-nucleotide-polymorphisms (SNP) rs10197412 of ZCCHC20 is associated with an increased risk for lung cancer in a Chinese population [Citation81].
3.3 ZCCHC21/RBM4
ZCCHC21, also named as RNA-binding motif protein 4 (RBM4) or Lark, is initially reported as a protein involved in circadian rhythm control of Drosophila, but has also been brought in sight in mammalian cells. It has a ubiquitous expression, with particularly high levels in testis, ovaries, heart and pancreas [Citation82]. ZCCHC21 structurally contains two RNA recognition motifs (RRMs) and a CCHC-type zinc finger in its N-terminus [Citation83], as well as an alanine repeat-containing C-terminal domain (CAD) in its C-terminus [Citation84]. Its C-terminus and RRMs are both responsible for binding a set of pre-mRNA cis-regulatory elements, necessary for conducting splicing regulation [Citation85]. The C-terminus also mediates protein–protein interactions, as well as nuclear speckle localization [Citation84,Citation86]. RRM2 is crucial for the nucleolar localization [Citation83]. The cellular function of zinc-finger motif has not yet been discovered, but it may act in concert with RRM domains and contribute to RNA binding specificity [Citation83]. ZCCHC21 mainly resides in nucleoli and nuclear speckles, where it colocalizes with SR-proteins and participates in pre-mRNA alternative splicing regulation [Citation83]. It can also translocate to cytoplasm with a perinuclear distribution, acting a role in mRNA translation regulation [Citation86].
ZCCHC21 is initially recognized as a regulator of AS. By binding with ISE downstream of Tau exon10, ZCCHC21 inhibits AS process and promotes the inclusion of Tau exon10, similarly but in opposite to ZCCHC20 [Citation87,Citation88]. Although its role is predominantly negative, ZCCHC21 also positively regulates AS by binding different cis-regulatory elements, as is the case for alpha-tropomyosin (TPM1) splicing regulation [Citation89]. ZCCHC21 antagonizes the suppressive splicing regulation of PTB protein by competitive binding to intronic CU-rich elements, stimulating exon skipping [Citation90]. To summarize, ZCCHC21 probably exhibits a binding site-dependent regulatory pattern: a binding to both exonic and intronic elements promotes splicing and leads to exon exclusion; on the contrary, a binding only to downstream intronic elements inhibits splicing and facilitates exon inclusion [Citation85]. Besides its bidirectional role in cis-splicing, ZCCHC21 also undergoes trans-splicing regulation. Trans-splicing between pre-mRNAs of ZCCHC21 and another splicing regulator CoAA (RBM14) yields a zinc finger-containing coactivator CoAZ and a non-coding splicing variant ncCoAZ during stem/progenitor cell neural differentiation, which influences Tau exon10 selection, presumably important for neural cell differentiation [Citation91]. In this feedback model, the trans-splicing product CoAZ antagonizes ZCCHC21 through the shared zinc-finger motif.
Splicing process regulated by ZCCHC21 seemingly requires the cooperation of other factors in order to expect a precise modulation. LncRNA TPM1-AS, which is naturally anti-sense transcribed from TPM1 intronic region, interacts and therefore hinders ZCCHC21 from binding to TPM1 pre-mRNA and inhibits its alternative splicing function, resulting in an increase of exon 2a inclusion[Citation92]. WT1 (+KTS), an isoform of tumour suppressor protein WT1, directly binds ZCCHC21 and abrogates its effect on splicing site selection[Citation93]. Moreover, ZCCHC21 is a part of supraspliceosome assembly, regulating alternative splicing sites selection of minigenes SMN2 and SRp20 [Citation86,Citation93].
ZCCHC21 is involved in common physiological process particularly cell differentiation and organogenesis by balancing of protein isoforms. Pyruvate kinase M2 (PKM2) to PKM1 isoform shift occurs at the time of neuron induction when ZCCHC21 generates more PKM1 through AS regulation, promoting neural differentiation [Citation94]. ZCCHC21 also plays a role in neuronal migration via regulating the expression of Dab1 splicing isoforms[Citation95]. By modulating IR-B, Pref-1 and PPAR-r isoform shift, ZCCHC21 manages the reprogramming splicing cascade during differentiation of brown adipocytes[Citation96]. ZCCHC21 alters isoform balance of Pax4 and Isl1 to activate the transformation to insulin-producing cells in β-islets[Citation97]. In myogenesis, ZCCHC21 downregulates and antagonizes PTB/mPTB, acting together to generate a set of muscle-specific protein isoforms through AS [Citation90]. Moreover, ZCCHC21 modulates splicing profiles change upon enteric infection in Drosophila, suggesting its potential role in host against infection [Citation98].
Dysregulation of alternative splicing results in protein isoform imbalance, and is relevant to carcinogenesis signatures including immortality, migration, angiogenesis, etc[Citation89].. ZCCHC21 is recognized as a tumour suppressor in various malignancies, including lung, breast, ovarian, pancreatic, liver, prostate and gastric cancer [Citation89,Citation99,Citation100]. Notably, the reduced expression of ZCCHC21 is associated with poor overall survival in lung, breast, and ovarian cancer in a Chinese population [Citation24]. ZCCHC21 can modulate isoform proportions of Bcl-x through alternative splicing towards a pro-apoptotic profile, suppressing proliferation and migration of various cancer cell lines [Citation89]. In breast cancer cells, the phosphorylation at Ser309 in the RDRSP domain of ZCCHC21 mediated by an SR protein kinase 1 (SRPK1) induces an cytoplasmic accumulation of ZCCHC21 [Citation85], which reduces pro-apoptotic protein isoforms MCL-1s and IR-B [Citation85,Citation101]. ZCCHC21 also mediates inflammatory response via splicing regulation of TNIP1 in HeLa cells [Citation102]. In colorectal cancer (CRC) cells, ZCCHC21 participates in reprogramming splicing profiles of FGFR2 and PKM2 genes, as well as mediates alternative splicing cascades of a set of genes including Nova1, SRSF6, VEGF165, modulating cell migration, metabolic and angiogenetic signature [Citation65,Citation103]. In human CRC tissues, ZCCHC21 can also antagonize another splicing factor, SRSF3, which binds to MAP4K4 exon16 CU-elements yielding a MAP4K4 variant that promote migration and invasion of CRC cells [Citation23,Citation100]. Similarly, ZCCHC21 and SRSF1 antagonistically regulate splicing profiles of HIF-1α, influencing oncogenic signatures of lung cancer cells [Citation104].
3.4 ZCCHC25/SF1
ZCCHC25, also named as splicing factor 1 (SF1), participates in early steps of pre-spliceosome assembly. It is highly conserved and has a particular high expression in bone marrow [Citation67]. It contains an N-terminal U2AF ligand motif (ULM), a central hnRNPK homology motif which specifically recognizes branch point sequence (BPS), as well as a CCHC-type zinc finger, and a C-terminal proline-rich domain [Citation105]. The spliceosome assembly follows a series of ordered events, during which ZCCHC25 cooperates with U2 ribonucleoprotein auxiliary factor 65-kDa subunit (U2AF[Citation64]) in recognizing 3ʹ splice-sites of pre-mRNAs and facilitating the ongoing of complex E to A evolution. As ZCCHC25 being a part of early complex E formation, U2AF[Citation64] is recruited by ZCCHC25 to splice sites [Citation106]. With its hnRNPK homology motif, ZCCHC25 can recognize BPS in the first place; however, this interaction is strengthened upon the presence of U2AF [Citation64,Citation107]. Additionally, ZCCHC25 associates with U2AF[Citation64] in nuclear speckles where they accumulate when not engaged in splicing [Citation108]. As for U2AF[Citation64], it bears the ability to recognize pre-mRNAs polypyrimidine (py) tract and 3ʹ splice site. Once the ZCCHC25/U2AF[Citation64]/pre-mRNA complex is established, it undergoes a conformational change with the presence of ATP [Citation109], which joins U2 snRNP and the branch point together [Citation105,Citation110], making the completion of complex A. Notably, ZCCHC25 is regulated by post-translational modification that is crucial for the machinery. Phosphorylation of Ser80/82 within a highly conserved SPSP motif of ZCCHC25 enhances the interaction of ZCCHC25/U2AF[Citation64] and pre-mRNAs [Citation111], whereas its phosphorylation by cGMP-dependent protein kinase-I (PKG-I) inhibits its binding to U2AF [Citation64,Citation111,Citation112]. Computational methods predict a potential phosphortylation site of ZCCHC25 nuclear localization signal (NLS), may turn out to be another regulatory pathway [Citation113]. Apart from spliceosome assembly and 3ʹ splice site recognition, ZCCHC25 is expected to participate in alternative splice site selection, especially by contacting branch-site like sequences. ZCCHC25 silencing results in disturbance of several endogenous AS variants, including changes of exon inclusion/skipping ratio [Citation114]. ZCCHC25 is regulated by the β-catenin/TCF4 complex, and conversely it interacts the complex to trans-activate gene expression and splicing activities[Citation115].
Evidence is accumulating that ZCCHC25 is a potential regulator of reproduction and germ cell tumorigenesis. The ZCCHC25 3ʹUTR variant g.423146327 T > C polymorphism increases litter size in small Tail Han sheep, suggesting its role in mammalian reproduction [Citation116]. Cross-breeding of Sf1± mice with 129-Ter and M19 mice exhibits decreased incidence of testicular germ cell tumours (TGCTs), indicating its oncogenic role in TGCTs [Citation117].
4. Translation
Translation is responsible for converting genetic information coded by mRNAs into functional proteins. The biological processes of translation includes initiation, elongation, termination and ribosome recycling. The first step, initiation, is the most regulated, with ZCCHC21 and ZCCHC22 involved (see for details). However, the translation initiation procedure differs a lot among species. In eukaryotes, the most predominant way is the canonical cap-dependent translation initiation, which involves m7G cap recognition at the 5ʹ end of mRNAs and ribosomal scanning [Citation118,Citation119]. The m7G cap is added at the 5ʹ end of mRNAs during transcription and is recognized by eukaryotic initiation factor 4E (eIF4E), which together with helicase eIF4A and scaffold protein eIF4G, forms a complex eIF4F [Citation120]. This complex then recruits the small ribosomal subunit 40S to active mRNAs, leading following events. A quite distinct mechanism of translation initiation, known as the cap-independent manner, is found in certain viral mRNAs possessing with internal ribosome entry sites (IRES) in the 5ʹ UTR [Citation121,Citation122]. Some eukaryotic mRNAs can also undergo cap-independent translation under certain circumstances such as stress [Citation64,Citation120]. N[Citation6]-methyladenosine (m6A) in 5ʹ UTR of eukaryotic mRNAs allows translation initiation to bypass m7G cap [Citation123]. Therefore, the concept of cap-independent translation enhancers (CITEs) is proposed to explain the cap-independent translation process in mammalians. Unlike IRESs, CITEs recognize both 5ʹ and 3ʹ UTRs and undergo ribosomal scanning as in canonical cap-dependent translation [Citation120]. Several zinc finger CCHC-type superfamily proteins, such as ZCCHC4, ZCCHC21/RBM4 and ZCCHC22/CNBP, are found to play key roles in mRNAs translation. The details will be discussed as follows.
Figure 3. ZCCHC21 and ZCCHC22 regulate translation initiation of eukaryotic mRNAs with a 5′-end m7G cap and a 3′-end poly(A)tail. The cap structure is recognized and combined by a group of eukaryotic initiation factors, eIF4E, eIF4G, eIF4A and eIF4B. Then the initiation complex recruits the 43S pre-initiation complex (PIC) to bind the m7G cap structure to activate cap-dependent translation. ZCCHC22 positively regulates whereas ZCCHC21 plays a dual role in this manner. If the mRNAs contain IRES motifs in the 5ʹ-end, m7G cap is no longer necessary. The phosphorylated ZCCHC21 at serine 309 stimulated by cell stress can stabilize eIF4A with IRES-containing mRNAs and initiate IRES-dependent translation. ZCCHC22 also promote this way in an unknown way. In addition, ZCCHC21 associates with Ago2 in myoblast cells to suppress mRNA translation. ZCCHC22 resolves G4 structure in 5ʹ-UTR RNA to implement its translational up-regulation
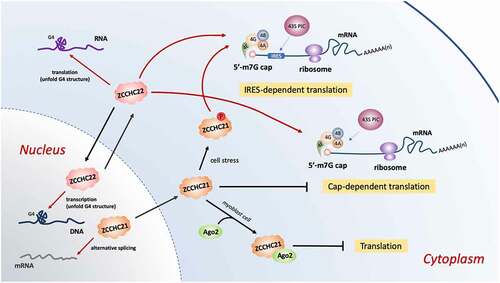
4.1 ZCCHC4
The ribosome consisting of rRNAs and proteins is where the translation takes place. The post-transcriptional modifications on rRNAs may influence translation efficiency. RNA m6A modification is ubiquitously present in mRNAs, rRNAs and snRNAs in human. The distribution of mRNA m6A regulates a wide range of biological processes, such as miRNA biogenesis [Citation96], stem cell pluripotency [Citation124,Citation125], RNA structural switches [Citation126], cancer and stress responses [Citation127,Citation128]. However, rRNA m6A still remains poorly understood.
ZCCHC4 is a newly discovered RNA m6A methyltransferase, which primarily catalyzes human 28S rRNA methylation at the position A4220 both in vitro and in vivo [Citation52,Citation129]. ZCCHC4 resides in the nucleolus and interacts with nucleolar proteins such as ASC-1 complex as well as m6A-reader YTHDC2 [Citation130]. The functional domains of ZCCHC4 are shown in . Structural analysis implicates that ZCCHC4-mediated rRNA methylation is critical for ribosome structure maintenance and subunit assembly[Citation52]. Through its 28S rRNA m6A methylation activity as well as interaction with a subset of mRNAs, ZCCHC4 influences global translation and cell proliferation. Loss of 28S rRNA m6A modification by knockout of ZCCHC4 reduces the strict control of translation, thus increasing mRNAs translation [Citation52]. ZCCHC4 is overexpressed in human hepatocellular carcinoma (HCC) tissues, while knockout of which inhibits the growth of HCC tumours in vivo [Citation52]. However, the detailed implication of ZCCHC4 in tumour biology remains to be further explored.
Figure 4. ZCCHC4 as RNA m6A methyltransferase methylates human 28S rRNA. By recognizing a stem-loop structure inherent in the human 28S rRNA, ZCCHC4 is activated to form a multi-domain RNA-binding surface. The integrated RNA-binding platform of ZCCHC4 is composed of a methyltransferase (MTase) domain, an N-terminal GRF-type and C2H2 zinc finger domains, and a C- terminal CCHC domain
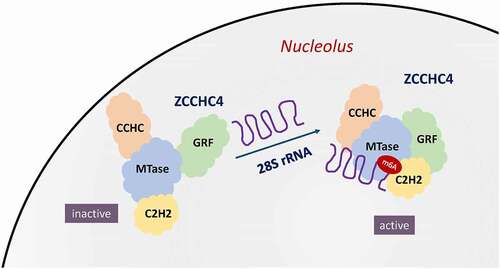
4.2 ZCCHC21/RBM4
ZCCHC21, also known as RNA-binding motif protein 4 (RBM4), is a multifunctional nucleocytoplasmic shuttling protein which not only participates in alternative splicing of pre-mRNA, but also has an impact on RNA translation [Citation86]. RBM4 is involved in the cell stress-induced signalling on translation initiation control. Arsenite exposure induces phosphorylation of RBM4 at serine 309 and drives its cytoplasmic accumulation to stress granule and nucleus via the MKK3/6-p38 signalling pathway, which subsequently suppresses cap-dependent translation in a cis-element-dependent manner [Citation64]. Accordingly, under arsenite exposure, RBM4 activates internal ribosome entry site (IRES)-mediated translation through enhancing the interaction between eIF4A and IRES-containing mRNAs [Citation64]. Hypoxia is another cellular stress which sequesters eIF4E thus repressing the cap-mediated translation [Citation131]. RBM4, along with hypoxia-inducible factor 2α (HIF-2α) and the cap-binding eIF4E2, forms an oxygen-regulated translation initiation complex that mediates selective cap-dependent protein synthesis under low oxygen tension. The complex captures the 5′ cap and recruits mRNAs to polysomes to activate translation, which ensures the protein synthesis in hypoxic cells [Citation132]. EGFR, known as a driver of tumorigenesis in certain cancer and a biomarker of tumour drug resistance [Citation133], is one of the representative proteins synthesized with the help of this complex under hypoxia [Citation132]. Interestingly, EGFR is found overexpressed in tumours that harbour a wild-type EGFR gene. Since tumour microenvironment is often hypoxic, overexpression of EGFR in tumours might be explained by this protein synthesis mechanism. Moreover, upregulated expression of RBM4 in tumour tissues is correlated with a better overall survival rate and disease-free survival rate in HCC patients and gastric cancer patients [Citation24,Citation134]. However, whether RBM4 is a potential target for cancer treatment, more investigations on its detailed roles in tumour biology are needed.
Besides, it is also found that RBM4 can directly interact with the 3ʹ-UTR of Period1 (Per1), an important circadian clock gene, without a splicing junction, which suggests that it upregulates Per1 expression at the level of translation in a cap/poly(A)-dependent manner [Citation135]. In myoblast cells, RBM4 co-localizes and interacts directly with Argonaute2 (AGO2) protein, a key component of miRNAs induced silencing complex (miRISC), therefore, to suppress the translation of target mRNAs. Moreover, it associates with some muscle cell-specific miRNAs thus enhancing the association between miRISCs and target mRNAs, which leads to more efficient translation suppression during muscle cell differentiation [Citation136]. This protein translation suppression mechanism directed by miRNAs-based mechanism can be rescued while RBM4 is phosphorylated at serine 309 by p38 MAPK. The phosphorylation of RBM4 leads to its nuclear re-localization, thus disrupting the interaction between RBM4 and AGO2 in the cytosol [Citation137].
4.3 ZCCHC22/CNBP
ZCCHC22/CNBP is involved in both transcription and translation. It participates in translational regulation and serves as a molecular chaperone through binding G-rich single-stranded nucleic acids [Citation138]. ZCCHC22 is found to be associated with the 5ʹ-terminal oligopyrimidine tract (5ʹTOP) mRNAs which encode RPS17 (ribosomal protein), poly(A)-binding protein 1 (PABP1), and two elongation factors eEF1A and eEF2, thus regulating the rate of cap-dependent translation[Citation139]. In Drosophila, ZCCHC22 promotes the c-myc Drosophila ortholog (dMyc) translation through an IRES-dependent mechanism [Citation140]. Similar to RBM4 mentioned above, under cellular stress, ZCCHC22 accumulates in stress granules, which supports a potential function for ZCCHC22 in mRNAs translation suppression [Citation141]. Based on the fact that ZCCHC22 functions as a G-quadruplex-unfolding protein in vitro, it may boost translation through resolving stable secondary structures within 5ʹ-UTR mRNAs [Citation44].
5. Degradation
RNA degradation exists ubiquitously in all organisms, which is a complex process accomplished via different pathways in the presence of various enzymes and cofactors. It is finally achieved by two major pathways: (1) the 5ʹ-3ʹ pathway including the Lsm1-7 complex, the decapping complex and 5ʹ-monophosphate-dependent exoribonuclease; (2) the 3ʹ-5ʹ pathway including a multi-subunit exosome complex [Citation142]. Certain members of ZCCHC superfamily play crucial roles in the regulation of RNA degradation by marking RNAs for the two separated degradation pathways, as illustrated in .
Figure 5. ZCCHC family play crucial roles in regulation of RNA degradation. In cytoplasm, ZCCHC11 and ZCCHC6 function redundantly as uridylyl-transferase to facilitate degradation of global mRNAs with short poly(A) tails and certain pre-miRNAs. For global mRNAs, for example, they enhance the mRNA degradation induced by miRNA (miR-1) and LINE-1 mRNA degradation in retro-transposition. For miRNA, ZCCHC11 and ZCCHC6 mark different type of miRNA for degradation. In the nucleus, ZCCHC11 participates in degradation of histone mRNA. ZCCHC8 and ZCCHC7 are a key component of the NEXT complex in nucleoplasm and the TRAMP-like complex in nucleolus, respectively. Both two complexes are related to the nuclear exosome, which is responsible for degradation of abnormal RNAs and the accurate 3′-end formation of functional RNAs
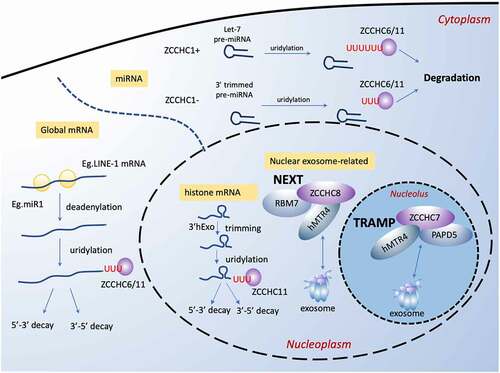
5.1 ZCCHC11/TUT4, ZCCHC6/TUT7, and ZCCHC1/Lin28A
ZCCHC11 and ZCCHC6, widespread in almost all human tissues, facilitate the global mRNA degradation as uridylyltransferases that selectively recognize and uridylate mRNA with a short poly(A) tail of fewer than ∼25 nucleotides (nt) [Citation143]. It is worth noting that such specificity results from the intrinsic capacity of ZCCHC11 and ZCCHC6 to detect the length of the poly(A) and the disassociation of poly(A) binding protein (PABP) as the poly(A) shortened [Citation143]. It is also indicated that oligo-uridylated mRNAs (with ≥2 uridines), rather than mono-uridylated mRNAs, are more specific products of uridylation by ZCCHC11 and ZCCHC6. Oligo-uridylated mRNAs serve as a main mark of degradation and have a stronger effect. Both 5ʹ-3ʹ and 3ʹ-5ʹ decay pathways are involved in the ZCCHC11 and ZCCHC6-regulated mRNAs degradation. The LSM1-7 complex (for 5ʹ-3ʹ pathway) and DIS3L2 (for 3ʹ-5ʹ pathway) are the downstream factors to recognize the oligo-U marks.
In murine zygotic cells, ZCCHC11 and ZCCHC6 expressions are triggered by YAP1-TEAD4 transcription factor to help clear up murine maternal transcripts, a process required for mouse early embryo development beyond the four-cell stage [Citation144]. Moreover, abnormal accumulation of maternal transcripts accompanied by decreased expression of ZCCHC11 and ZCCHC6 are observed in the development-arrested human embryos, indicating the role of ZCCHC11 and ZCCHC6 in the degradation of mammalian maternal mRNAs [Citation145]. Similarly, ZCCHC11 and ZCCHC6 are required for 3ʹ uridylation-programmed zygotene mRNA clearance in pachytene spermatocytes that is essential for male meiotic progression [Citation146].
Uridylation of LINE-1 mRNA 3ʹ ends by ZCCHC11 or ZCCHC6 in cooperation with the helicase/RNPase MOV10 counteracts the RNA chaperone activity of the L1-ORF1p retrotransposon protein, thus restricting the retro-transposition of LINE-1s at the mRNA level through mRNA degradation, which enhances the genome stability [Citation147]. In addition to mRNAs with short poly(A) tails, ZCCHC11 also uridylates mRNAs that are not polyadenylated, such as histone mRNA end in a conserved stem-loop rather than a poly(A) tail [Citation148]. Once DNA replication is inhibited, usually at the end of the S stage [Citation149], histone mRNA will be trimmed by 3ʹhExo at the 3ʹ terminal near its stem loop and then uridylated by ZCCHC6/TUT7 [Citation150]. The uridylation leads to degradation with both 5ʹ-3ʹ degradation pathway via decapping and 3ʹ-5ʹ degradation pathway via RNA exosome [Citation149]. However, ZCCHC11 has not been reported to function like that.
Besides mature mRNAs, ZCCHC6 and ZCCHC11 play an important role in pre-miRNA degradation as well. In stem cells and cancer cells, ZCCHC11 and ZCCHC6 serve as suppressors of let-7 biogenesis by uridylating let-7 pre-miRNA for degradation hence augmenting mRNA of certain genes [Citation151,Citation152], which seems to be inconsistent with studies in global mRNA degradation. Yet, double knockdown of ZCCHC11 and ZCCHC6 does not affect the let-7 levels in the presence of ZCCHC1/Lin28A [Citation153], instead, the overall mRNAs are upregulated [Citation143], suggesting that ZCCHC11 and ZCCHC6 determine general mRNA levels through mRNA degradation but not let-7 miRNA biogenesis.
ZCCHC1/Lin28A is enriched in human testis and spermatids. It selectively binds to the terminal loop of let-7 pre-miRNA with a CCHC-type zinc finger RNA-binding domain, and subsequently recruit ZCCHC11 or ZCCHC6 [Citation154], which drives the formation of a stable ternary complex between pre-let-7 and the Lin28-interacting motif (LIM) of ZCCHC11 [Citation155,Citation156]. As a result of recruitment, ZCCHC11 adds an oligouridine tail of 10–30 nt to the 3ʹ-terminus by recognizing a specific RNA sequences motif of GGAG in the terminal loop [Citation151,Citation154]. The uridylated let-7 pre-miRNA is resistant to Dicer1 and may be sequestered in the cytoplasm [Citation157]. ZCCHC6 plays a similar role but has weaker activity [Citation154]. A set of other pre-miRNAs with GGAG are also subject to uridylation in this ZCCHC1-dependent manner, such as pre-miR-107,-143 and −200 C [Citation154]. In addition to the zinc finger RNA-binding domain, ZCCHC1 also harbour a cold shock domain (CSD) which recognizes a (U)GAU motif in a subclass of let-7 pre-miRNA family and enhances its binding [Citation158].
The repression of let-7 expression is required for normal development and ZCCHC1 contributes to maintain the pluripotent state by preventing let-7–mediated differentiation of embryonic stem cells and neural stem cell commitment [Citation154,Citation159]. Accordingly, aberrant expression of ZCCHC1 is associated with cancer progression in multiple tissues [Citation160–162]. We recently find that in cancer hypoxia environment, activation of ZCCHC1 is driven by SUMOylation [Citation163]. Thereby, its inhibition of let-7 maturation is promoted, resulting in cancer cell proliferation, migration, invasion, and tumour growth in vivo [Citation163]. Interestingly, a recent study has screened a nanobody called Nb-S2A4 for therapeutic purposes, which targets the ZCCHC1:let-7 interaction fragment of ZCCHC11/TUT4 thus blocking uridylation of let-7 [Citation164].
In somatic cells with absence of ZCCHC1, ZCCHC6 or ZCCHC11 recognizes the 3′ trimmed group II pre-miRNAs, which the 3ʹ end of pre-miRNAs is further recessed into the stem, and generates an oligo-U tail that leads to degradation. This is deemed as a clearance mechanism of non-functional pre-miRNAs [Citation165]. In contrast to typical group I pre-miRNAs with a 2 nt overhang at the 3ʹ end, group II pre-miRNAs acquire a shorter (1 nt) 3ʹ overhang to form a bulge next to the Drosha cleavage site [Citation153]. As shown in , this mechanism expands the field of miRNAs regulation at the post-transcriptional level. Besides pre-miRNA degradation, ZCCHC6 and ZCCHC11 are also reported to uridylate cytokine-targeting mature miRNAs, thus regulating the stability of transcripts for interleukin-6 (IL-6) and IGF-1 [Citation166,Citation167]. For example, ZCCHC6 enhances IL-6 expression in chondrocytes by uridylating miR-26b for degradation, which then alleviates the severity of osteoarthritis in mice [Citation168].
Figure 6. ZCCHC11 and ZCCHC6 regulate miRNA biogenesis in the presence or absence of ZCCHC1. (1) In embryonic stem cells and some cancer cells, ZCCHC1/Lin28A recruits ZCCHC6 or ZCCHC11 to oligouridylate pre-let-7 at the 3′end that inhibits its maturation. (2) In somic cells where lack of ZCCHC1, Group II pre-miRNA with a shorter 3′ overhang can be monouridylated by ZCCHC11 or ZCCHC6 that enhances miRNA biogenesis
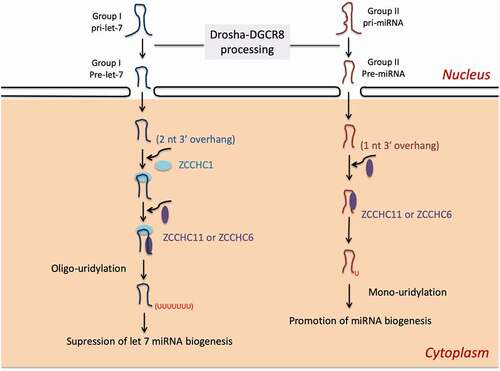
The role of ZCCHC6 and ZCCHC11 in regulating mature miRNA dynamically has been recently reported. They play a crucial part in turnover of mature miRNAs bound by Argonaute proteins in the RNA-induced silencing complex (RISC). The exposed 3ʹ ends of miRNA, which is caused by frequent sequence modifications, are uridylated by ZCCHC6 and ZCCHC11 to facilitate DIS3L2-mediated degradation [Citation169]. Moreover, in miRNA strand selection, uridylation of pre-miR-324 by ZCCHC11 and ZCCHC6 re-positions Dicer1 on the pre-miRNA and shifts the cleavage site. This alternative processing produces a duplex with a different terminus from which the 3ʹ strand (3p) is selected instead of the 5ʹ strand (5p), resulting in different post-transcriptional effects [Citation170].
To summarize, ZCCHC11 and ZCCHC6 redundantly regulate the general degradation of mRNAs with short poly(A) tails in mammalian cells [Citation143]. Particularly, ZCCHC6 can regulate the degradation of histone mRNA. They also facilitate degradation of certain pre-miRNAs such as let–7 family after recruited by ZCCHC1 [Citation171] and turnover of mature miRNAs bound by Argonaute proteins [Citation166–169]. In mammalian gametes and embryos, they clear up parental mRNAs to facilitate mammalian spermatogenesis and embryonic development [Citation144,Citation145]. As uridylyl transferases for RNA, ZCCHC6 and ZCCHC11 are widely involved in process related to cell fate through the regulation of stability of genomes and transcripts, which makes them underlying targets for development abnormality, cancer and tissue regeneration.
5.2 ZCCHC7
ZCCHC7, together with PAP‐associated domain‐containing protein 5 (PAPD5) and RNA helicase hMTR4/DOB1/SKIV2L2, constitutes the TRAMP-like complex in mammalian cells [Citation172]. The well-established TRAMP complex model in Saccharomyces cerevisiae suggests that TRAMP complex recognizes and polyadenylates abnormal coding and non-coding RNAs, hence working as a cofactor of nuclear exosomes to promote RNA degradation [Citation173], but the structure and function of the mammalian TRAMP-like complex are still poorly studied. It is now clear that ZCCHC7 and PAPD5 interdependently interact with the exosome in the presence of the catalytic subunit RRP6, which takes place only in nucleoli [Citation174], unlike their homologues in S. cerevisiae [Citation173]. Th exosome functions include degradation of abnormal RNAs and the accurate 3ʹ‐end formation of functional RNAs, such as rRNAs, small nuclear RNAs (snRNAs), and small nucleolar RNAs (snoRNAs) [Citation175], which may provide a hint of ZCCHC7 function in guiding RNA degradation. Indeed, it has been revealed that ZCCHC7 together with PAPD5 contribute to the 3ʹend adenylation of abortive RNA polymerase I (Pol I) transcripts as marks for degradation by the nuclear exosome [Citation176] and interact closely with components of the small ribosomal subunit (SSU) processome which is essential in the early steps of pre‐rRNA processing [Citation174]. Consistently, the further investigation has shown that ZCCHC7 and PAPD5 are involved in the degradation of 5ʹ-ETS by‐product RNAs excised from the pre‐rRNA [Citation177], similar to their homologues in the yeast. In brief, ZCCHC7 is related closely to the nucleolar exosome function and acts interdependently with PAPD5 to affect pre‐rRNA 5ʹ-ETS elimination. However, whether ZCCHC7 facilitates degradation of other RNAs via exosome remains to be observed.
5.3 ZCCHC8
ZCCHC8 is first identified as a substrate of GSK-3, and Intriguingly, it is phosphorylated by GSK-3 at the spindle checkpoint [Citation7]. Contrary to ZCCHC7, ZCCHC8 exists in the nucleoplasm rather than in the nucleoli. ZCCHC8 binds with two proteins: hMTR4, an RNA helicase, and RBM7, an RNA binding protein, to form a nuclear exosome targeting complex (NEXT complex) [Citation174]. The NEXT complex directs a large scale of non-coding RNA for the nuclear exosome degradation, such as lncRNAs, non-coding promoter-upstream transcripts, 3ʹ-extended products of histone and small nuclear RNA transcription [Citation174,Citation178,Citation179]. Taken the zinc-knuckle domain of ZCCHC8 and the similarity between the NEXT complex and the TRAMP complex into consideration, both ZCCHC8 and RBM7 might act to recognize and target RNAs for degradation. Thus, together with hMTR4, ZCCHC8/RBM7 and target RNAs into the exosome for degradation [Citation174]. The depletion of ZCCHC8 of the NEXT complex in mouse leads to developmental defects, a shortened lifespan, and infertility, for which the underlying mechanism is proposed that the ZCCHC8-mediated degradation of transcripts of retrotransposon element LINE1 in early embryos and embryonic stem cells (ESCs) [Citation180].
Moreover, the crystal structure of the NEXT displays a proline-rich segment of ZCCHC8 as the interaction site for the RNA-recognition motif (RRM) of RBM7 [Citation181]. But interestingly, RBM7 is also competitively bound to a splicing factor SAP145a [Citation182] which consists of a proline-rich segment with strong similarity to ZCCHC8 [Citation181], thereby directing to the spliceosome other than the exosome. It still remains unclear whether there is a correlation between these traits of ZCCHC8 and its function, whether and how ZCCHC8 competes with SAP145a. In addition, ZCCHC8 contains short linear motifs that bind to the hMTR4 arch domain in a mutually exclusive manner with a ribosome processing adapter NVL, suggesting ZCCHC8 as an adapter to direct exosomes for small nuclear RNA degradation [Citation183]. The question concerning about the exact role of ZCCHC8 in the exosome-mediated RNA degradation is still open.
In RNA degradation, similarities exist among certain ZCCHC family proteins. For instance, ZCCHC1 use its zinc finger domain to recognize the targeted let-7 pre-miRNA. ZCCHC6 and ZCCHC11 act redundantly as uridylyl-transferases to mark RNAs for degradation [Citation143], while ZCCHC7 and ZCCHC8 are the main components of the TRAMP-like complex in nucleoli and the NEXT complex in nucleoplasm, respectively, and both are assumed to be cofactors of the nuclear exosome [Citation173,Citation174], which finally degrades those marked RNAs. However, the functions of ZCCHC family proteins in RNA degradation have not yet been fully understood. Whether and how their common zinc finger domain work as key motifs for their functions, such as RNA substrate selectivity and the correlations between those proteins, have to be elucidated since they seem to coordinate in the same regulating axis/pathway of RNA degradation to guide the fate of the cells.
6. Summary
The regulation of RNA metabolism is regarded to be crucial in various biological processes, such as gene expression, cell proliferation, differentiation, as well as catabolic process and cellular response to stimulus. The CCHC-type zinc fingers exist in all family members of ZCCHC proteins and some even harbours more than one consensus sequence, except for ZCCHC23, on account of its histidine (H) residue being substituted by asparagine (N). As the high affinity to single-stranded nucleic acids (not including ZCCHC18) of the distinct ZCCHC motif, the relevance of CCHC-type zinc finger proteins to RNA metabolism are doomed. It is the consensus motif that serves as the guide to target RNAs, despite the unnecessariness to play the most significant role in performing molecular functions.
ZCCHC superfamily has emerged as distinct roles in various aspects of RNA metabolism. (1) ZCCHC12 and ZCCHC22 function as the transcriptional activator thus influencing the progress of RNA transcription/biogenesis. ZCCHC3, ZCCHC9, and ZCCHC10 are also found to affect RNA transcription, but more works are needed to elucidate the mechanisms. (2) The roles of ZCCHC family in splicing are diverged. ZCCHC17, ZCCHC20 and ZCCHC25 are associated with SR protein family, while ZCCHC21 functions as a regulator of alternative splicing (AS). (3) Translation control is another important facet. ZCCHC21 and ZCCHC22 regulate the translation initiation stage, and ZCCHC4 methylates human 28S rRNA to affect global translation. (4) As for degradation, ZCCHC1 mediates the processing of miRNA let-7 together with ZCCHC6 and ZCCHC11, as uridylyl-transferases, to facilitate RNA degradation. Related closely to nuclear exosome function, ZCCHC7 and ZCCHC8 also promote RNA degradation.
The conservation of ZCCHCs in different species indicates the biologically relevant functions of the specific CCHC motif. As far as we know, it has been associated with tumorigenesis, stem cell pluripotency and differentiation, reprogramming, metabolism, inflammation and so on. Although a census of ZCCHC family as RBPs comes to the fore, research concerning their exact roles on RNA are divided, and many even achieved opposite conclusions. Besides, only a limit part of ZCCHC proteins have explicit structure, while most still lacks structural research. Many disease states are derived from long-term RNA metabolism dysregulation, thus diving deep into the regulation mechanism of RNA metabolism via ZCCHC family proteins should shed light on both the physiological and pathological functions in human beings.
Disclosure statement
No potential conflict of interest was reported by the author(s).
Additional information
Funding
References
- Summers MF Zinc finger motif for single-stranded nucleic acids? Investigations by nuclear magnetic resonance. J Cell Biochem. 1991; 45(1):41–48.
- Miller J, McLachlan AD, Klug A Repetitive zinc-binding domains in the protein transcription factor IIIA from Xenopus oocytes. Embo J. 1985; 4(6):1609–1614.
- Gerstberger S, Hafner M, Tuschl T A census of human RNA-binding proteins. Nat Rev Genet. 2014; 15(12):829–845.
- Rajavashisth TB, Taylor AK, Andalibi A, et al. Identification of a zinc finger protein that binds to the sterol regulatory element. Science. 1989; 245(4918):640–643.
- Minoda Y, Saeki K, Aki D, et al. A novel Zinc finger protein, ZCCHC11, interacts with TIFA and modulates TLR signaling. Biochem Biophys Res Commun. 2006; 344(3):1023–1030.
- Zhou A, Zhou J, Yang L, et al. A nuclear localized protein ZCCHC9 is expressed in cerebral cortex and suppresses the MAPK signal pathway. J Genet Genomics. 2008; 35(8):467–472.
- Gustafson MP, Welcker M, Hwang HC, et al. Zcchc8 is a glycogen synthase kinase-3 substrate that interacts with RNA-binding proteins. Biochem Biophys Res Commun. 2005; 338(3):1359–1367.
- Lian H, Wei J, Zang R, et al. ZCCHC3 is a co-sensor of cGAS for dsDNA recognition in innate immune response. Nat Commun. 2018; 9(1):3349.
- Liu X, Chen Q, Tsai HJ, et al. Maternal preconception body mass index and offspring cord blood DNA methylation: exploration of early life origins of disease. Environ Mol Mutagen. 2014; 55(3):223–230.
- Ohira T, Kojima H, Kuroda Y, et al. PITX1 protein interacts with ZCCHC10 to regulate hTERT mRNA transcription. PLoS One. 2019; 14(8):e0217605.
- Dai H, Yan M, Li Y The zinc-finger protein ZCCHC2 suppresses retinoblastoma tumorigenesis by inhibiting HectH9-mediated K63-linked polyubiquitination and activation of c-Myc. Biochem Biophys Res Commun 2020; 521(2):533–538.
- Zayats T, Jacobsen KK, Kleppe R, et al. Exome chip analyses in adult attention deficit hyperactivity disorder. Transl Psychiatry. 2016; 6(10):e923.
- An Q, Wright SL, Moorman AV, et al. Heterogeneous breakpoints in patients with acute lymphoblastic leukemia and the dic(9;20)(p11-13;q11) show recurrent involvement of genes at 20q11.21. Haematologica. 2009; 94(8):1164–1169.
- Cocce MC, Mardin BR, Bens S, et al. Identification of ZCCHC8 as fusion partner of ROS1in a case of congenital glioblastoma multiforme with a t(6;12)(q21;q24.3). Genes Chromosomes Cancer. 2016; 55(9):677–687.
- Gable DL, Gaysinskaya V, Atik CC, et al. ZCCHC8, the nuclear exosome targeting component, is mutated in familial pulmonary fibrosis and is required for telomerase RNA maturation. Genes Dev. 2019; 33(19–20):1381–1396.
- Li QL, Lai R, Guo ZM, et al. ZCCHC12, a potential molecular marker of papillary thyroid carcinoma: a preliminary study. Med Oncol. 2012; 29(3):1409–1417.
- Wang O, Zheng Z, Wang Q, et al. ZCCHC12, a novel oncogene in papillary thyroid cancer. J Cancer Res Clin Oncol. 2017; 143(9):1679–1686.
- Tomljanovic Z, Patel M, Shin W, et al. ZCCHC17 is a master regulator of synaptic gene expression in Alzheimer’s disease. Bioinformatics. 2018; 34(3):367–371.
- Botta A, Caldarola S, Vallo L, et al. Effect of the [CCTG]n repeat expansion on ZNF9 expression in myotonic dystrophy type II (DM2). Biochim Biophys Acta. 2006; 1762(3):329–334.
- Zhang Y, Xiao X, Zhou W, et al. LIN28A-stabilized FBXL19-AS1 promotes breast cancer migration, invasion and EMT by regulating WDR66. InVitro Cell Dev Biol Anim. 2019; 55(6):426–435.
- Boguslawska J, Sokol E, Rybicka B, et al. microRNAs target SRSF7 splicing factor to modulate the expression of osteopontin splice variants in renal cancer cells. Gene. 2016; 595(2):142–149.
- Saijo S, Kuwano Y, Masuda K, et al. Serine/arginine-rich splicing factor 7 regulates p21-dependent growth arrest in colon cancer cells. JMed Invest. 2016; 63(3.4):219–226.
- Lin JC, Lee YC, Tan TH, et al. RBM4-SRSF3-MAP4K4 splicing cascade modulates the metastatic signature of colorectal cancer cell. Biochim Biophys Acta Mol Cell Res. 2018; 1865(2):259–272.
- Yong H, Zhu H, Zhang S, et al. Prognostic value of decreased expression of RBM4 in human gastric cancer. Sci Rep. 2016; 6(1):28222.
- Li Z, Li Z, Wang L, et al. ZCCHC13-mediated induction of human liver cancer is associated with the modulation of DNA methylation and the AKT/ERK signaling pathway. J Transl Med. 2019; 17(1):108.
- Shi X, Jiang B, Liu H, et al. ZCCHC9 promotes proliferation and invasion of lung cancer through regulating the JNK pathway. J Cell Biochem. 2019; 120(6):10596–10604.
- Li W, Li X, Gao LN, et al. Integrated analysis of the functions and prognostic values of RNA binding proteins in lung squamous cell carcinoma. Front Genet. 2020; 11:185.
- Ginam C, Youngshin L, Dina Z, et al. Sizn1 is a novel protein that functions as a transcriptional coactivator of bone morphogenic protein signaling. Mol Cell Biol. 2008; 28(5):1565–1572.
- Feng XH, Derynck R Specificity and versatility in TGF-beta signaling through Smads. Annu Rev Cell Dev Biol. 2005; 21(1):659–693.
- Janknecht R, Hunter T Versatile molecular glue. Transcriptional control. Curr Biol. 1996; 6(8):951–954.
- Janknecht R, Hunter T Transcription. A growing coactivator network. Nature. 1996; 383(6595):22–23.
- Ginam C, Youngshin L, Golden JA SUMO interaction motifs in Sizn1 are required for promyelocytic leukemia protein nuclear body localization and for transcriptional activation. J Biol Chem 2009; 284(29):19592–19600.
- Boisvert FM, Hendzel MJ, Bazett-Jones DP Promyelocytic leukemia (PML) nuclear bodies are protein structures that do not accumulate RNA. J Cell Biol. 2000; 148(2):283–292.
- Bernardi R, Pandolfi PP Structure, dynamics and functions of promyelocytic leukaemia nuclear bodies. Nat Rev Mol Cell Biol. 2007; 8(12):1006–1016.
- Li H, Liu Q, Hu X, et al. Human ZCCHC12 activates AP-1 and CREB signaling as a transcriptional co-activator. Acta Biochim Biophys Sin (Shanghai). 2009; 41(7):535–544.
- Bannister AJ, Kouzarides T CBP-induced stimulation of c-Fos activity is abrogated by E1A. Embo J. 1995; 14(19):4758–4762.
- Chrivia JC, Kwok RP, Lamb N, et al. Phosphorylated CREB binds specifically to the nuclear protein CBP. Nature. 1993; 365(6449):855–859.
- Cho G, Lim Y, Golden JA XLMR candidate mouse gene, Zcchc12 (Sizn1) is a novel marker of Cajal-Retzius cells. Gene Expr Patterns. 2011; 11(3–4):216–220.
- Li L, Wang P, Liu S, et al. Transcriptome sequencing of endometrium revealed alterations in mRNAs and lncRNAs after ovarian stimulation. J Assist Reprod Genet. 2020; 37(1):21–32.
- Michelotti EF, Tomonaga T, Krutzsch H, et al. Cellular nucleic acid binding protein regulates the CT element of the human c-myc protooncogene. J Biol Chem. 1995; 270(16):9494–9499.
- Borgognone M, Armas P, Calcaterra NB Cellular nucleic-acid-binding protein, a transcriptional enhancer of c-Myc, promotes the formation of parallel G-quadruplexes. Biochem J. 2010; 428(3):491–498.
- Huppert JL, Balasubramanian S Prevalence of quadruplexes in the human genome. Nucleic Acids Res 2005; 33:2908–2916. 9
- David AP, Pipier A, Pascutti F, et al. CNBP controls transcription by unfolding DNA G-quadruplex structures. Nucleic Acids Res. 2019; 47(15):7901–7913.
- Benhalevy D, Gupta SK, Danan CH, et al. The human CCHC-type Zinc Finger nucleic acid-binding protein binds G-rich elements in target mRNA coding sequences and promotes translation. Cell Rep. 2017; 18(12):2979–2990.
- Qiu J, Liu J, Chen S, et al. Role of Hairpin-Quadruplex DNA secondary structural conversion in the promoter of hnRNP K in gene transcriptional regulation. Org Lett. 2015; 17(18):4584–4587.
- Flink IL, Morkin E, Alternatively processed isoforms of cellular nucleic acid-binding protein interact with a suppressor region of the human beta-myosin heavy chain gene. J Biol Chem. 1995; 270(12):6959–6965.
- Margarit E, Armas P, Garcia Siburu N, et al. CNBP modulates the transcription of Wnt signaling pathway components. Biochim Biophys Acta. 2014; 1839(11):1151–1160.
- Lee E, Lee TA, Kim JH, et al. CNBP acts as a key transcriptional regulator of sustained expression of interleukin-6. Nucleic Acids Res. 2017; 45(6):3280–3296.
- Chen Y, Sharma S, Assis PA, et al. CNBP controls IL-12 gene transcription and Th1 immunity. J Exp Med. 2018; 215(12):3136–3150.
- Cao L, Zhang P, Li J, et al. LAST, a c-Myc-inducible long noncoding RNA, cooperates with CNBP to promote CCND1 mRNA stability in human cells. eLife. 2017;6(2017–12–02):6.
- Lin YM, Chu PH, Ouyang P Ectopically expressed pNO40 suppresses ribosomal RNA synthesis by inhibiting UBF-dependent transcription activation. Biochem Biophys Res Commun. 2019; 516(2):381–387.
- Ma H, Wang X, Cai J, et al. N(6-)Methyladenosine methyltransferase ZCCHC4 mediates ribosomal RNA methylation. Nat Chem Biol. 2019; 15(1):88–94.
- Tafforeau L, Zorbas C, Langhendries JL, et al. The complexity of human ribosome biogenesis revealed by systematic nucleolar screening of Pre-rRNA processing factors. Mol Cell. 2013; 51(4):539–551.
- Lian H, Zang R, Wei J, et al. The Zinc-Finger protein ZCCHC3 binds RNA and facilitates viral RNA sensing and activation of the RIG-I–like receptors. Immunity 2018; 49(3):438–48 e5.
- Yamashita M, Chattopadhyay S, Fensterl V, et al. Epidermal growth factor receptor is essential for Toll-like receptor 3 signaling. Sci Signal. 2012;5(233):ra50.
- Zang R, Lian H, Zhong X, et al. ZCCHC3 modulates TLR3-mediated signaling by promoting recruitment of TRIF to TLR3. J Mol Cell Biol. 2020;12 4:251–262.
- Ma ZH, Shi PD, Wan BS MiR-410-3p activates the NF-kappaB pathway by targeting ZCCHC10 to promote migration, invasion and EMT of colorectal cancer. Cytokine. 2021;140:155433.
- Chen M, Manley JL Mechanisms of alternative splicing regulation: insights from molecular and genomics approaches. Nat Rev Mol Cell Biol. 2009; 10(11):741–754.
- Xing Y, Lee C Alternative splicing and RNA selection pressure–evolutionary consequences for eukaryotic genomes. Nat Rev Genet. 2006;7(7):499–509.
- Mayer MG, Floeter-Winter LM Pre-mRNA trans-splicing: from kinetoplastids to mammals, an easy language for life diversity. Mem Inst Oswaldo Cruz. 2005; 100(5):501–513.
- Jurica MS, Moore MJ Pre-mRNA splicing: awash in a sea of proteins. Mol Cell 2003;12(1):5–14.
- Nilsen TW The spliceosome: the most complex macromolecular machine in the cell? Bioessays. 2003; 25(12):1147–1149.
- Cieply B, Park JW, Nakauka-Ddamba A, et al. Multiphasic and dynamic changes in alternative splicing during induction of pluripotency are coordinated by numerous RNA-binding proteins. Cell Rep 2016;15(2):247–255.
- Lin JC, Hsu M, Tarn WY Cell stress modulates the function of splicing regulatory protein RBM4 in translation control. Proc Natl Acad Sci U S A. 2007;104(7):2235–2240.
- Lin YM, Chu PH, Li YZ, et al. Ribosomal protein pNO40 mediates nucleolar sequestration of SR family splicing factors and its overexpression impairs mRNA metabolism. Cell Signal. 2017;32:12–23.
- Chang W-L, Lee D-C, Leu S, et al. Molecular characterization of a novel nucleolar protein, pNO40. Biochem Biophys Res Commun. 2003;307(3):569–577.
- Fagerberg L, Hallström BM, Oksvold P, et al. Analysis of the human tissue-specific expression by genome-wide integration of transcriptomics and antibody-based proteomics. Mol Cell Proteomics. 2014;13(2):397–406.
- Cléry A, Blatter M, Allain FHT RNA recognition motifs: boring? Not quite. Curr Opin Struct Biol. 2008;18(3):290–298.
- Brugiolo M, Botti V, Liu N, et al. Fractionation iCLIP detects persistent SR protein binding to conserved, retained introns in chromatin, nucleoplasm and cytoplasm. Nucleic Acids Res. 2017;45(18):10452–10465.
- Cavaloc Y, Bourgeois CF, Kister L, et al. The splicing factors 9G8 and SRp20 transactivate splicing through different and specific enhancers. Rna. 1999;5(3):468–483.
- Raponi M, Kralovicova J, Copson E, et al. Prediction of single-nucleotide substitutions that result in exon skipping: identification of a splicing silencer in BRCA1 exon 6. Hum Mutat. 2011;32(4):436–444.
- Shen H, Kan JLC, Green MR Arginine-serine-rich domains bound at splicing enhancers contact the branchpoint to promote prespliceosome Assembly. Mol Cell. 2004;13(3):367–376.
- Galiana-Arnoux D, Lejeune F, Gesnel MC, et al. The CD44 alternative v9 exon contains a splicing enhancer responsive to the SR proteins 9G8, ASF/SF2, and SRp20. J Biol Chem. 2003;278(35):32943–32953.
- Tejedor JR, Papasaikas P, Valcárcel J Genome-wide identification of Fas/CD95 alternative splicing regulators reveals links with iron homeostasis. Mol Cell. 2015;57(1):23–38.
- Müller-mcnicoll M, Botti V, De Jesus Domingues MA, et al. SR proteins are NXF1 adaptors that link alternative RNA processing to mRNA export. Genes development. 2016;30(5):553–566.
- Konigs V, De Oliveira Freitas Machado C, Arnold B, et al. SRSF7 maintains its homeostasis through the expression of Split-ORFs and nuclear body assembly. Nat Struct Mol Biol. 2020; 27(3):260–273.
- Shepard PJ, Hertel KJJGB. The SR protein family. Genome Biol. 2009;10(10):242.
- Krchnakova Z, Thakur PK, Krausova M, et al. Splicing of long non-coding RNAs primarily depends on polypyrimidine tract and 5ʹ splice-site sequences due to weak interactions with SR proteins. Nucleic Acids Res. 2019; 47(2):911–928.
- Kadota Y, Jam FA, Yukiue H, et al. Srsf7 establishes the juvenile transcriptome through age-dependent alternative splicing in Mice. iScience. 2020;23(3):100929.
- Fu Y, Wang Y SRSF7 knockdown promotes apoptosis of colon and lung cancer cells. Oncol Lett. 2018;15(4):5545–5552.
- Shen W, Yin R, Wang C, et al. Polymorphisms in alternative splicing associated genes are associated with lung cancer risk in a Chinese population. Lung Cancer. 2015;89(3):238–242.
- Höck J, Weinmann L, Ender C, et al. Proteomic and functional analysis of Argonaute-containing mRNA-protein complexes in human cells. EMBO Rep. 2007;8(11):1052–1060.
- Markus MA, Morris BJ Lark is the splicing factor RBM4 and exhibits unique subnuclear localization properties. DNA Cell Biol. 2006;25(8):457–464.
- Chang SH, Chang WL, Lu CC, et al. Alanine repeats influence protein localization in splicing speckles and paraspeckles. Nucleic Acids Res. 2014;42(22):13788–13798.
- Lu CC, Chen TH, Wu JR, et al. Phylogenetic and molecular characterization of the splicing factor RBM4. PLoS One. 2013;8(3):e59092.
- Markus MA, Morris BJ RBM4: a multifunctional RNA-binding protein. Int J Biochem Cell Biol 2009; 41:740–743. 4
- Kar A, Havlioglu N, W-y T, et al. RBM4 interacts with an intronic element and stimulates Tau Exon 10 inclusion. 2006.
- Gao L, Wang J, Wang Y, et al. SR protein 9G8 modulates splicing of tau exon 10 via its proximal downstream intron, a clustering region for frontotemporal dementia mutations. Mol Cell Neurosci. 2007; 34(1):48–58.
- Wang Y, Chen D, Qian H, et al. The splicing factor RBM4 controls apoptosis, proliferation, and migration to suppress tumor progression. Cancer Cell. 2014;26(3):374–389.
- Lin J-C, Tarn W-Y RBM4 down-regulates PTB and antagonizes its activity in muscle cell–specific alternative splicing. J Cell Biol. 2011;193(3):509–520.
- Brooks YS, Wang G, Yang Z, et al. Functional pre- mRNAtrans-splicing of coactivator CoAA and corepressor RBM4 during Stem/Progenitor cell differentiation. J Biol Chem. 2009; 284(27):18033–18046.
- Huang GW, Zhang YL, Liao LD, et al. Natural antisense transcript TPM1-AS regulates the alternative splicing of tropomyosin I through an interaction with RNA-binding motif protein 4. Int J Biochem Cell Biol. 2017;90:59–67.
- Markus MA, Heinrich B, Raitskin O, et al. WT1 interacts with the splicing protein RBM4 and regulates its ability to modulate alternative splicing in vivo. Exp Cell Res. 2006;312(17):3379–3388.
- Su C-H, Hung K-Y, Hung S-C, et al. RBM4 regulates neuronal differentiation of mesenchymal stem cells by modulating alternative splicing of Pyruvate Kinase M. Mol Cell Biol. 2017;37(3):37.
- D D, Ky H, Tarn WY RBM4 modulates radial migration via alternative splicing of Dab1 during cortex development. Mol Cell Biol 2018;38(12):38.
- Lin JC, Tarn WY, Hsieh WK Emerging role for RNA binding motif protein 4 in the development of brown adipocytes. Biochim Biophys Acta. 2014;1843(4):769–779.
- Lin JC, Yan YT, Hsieh WK, et al. RBM4 promotes pancreas cell differentiation and insulin expression. Mol Cell Biol. 2013;33(2):319–327.
- Bou Sleiman M, Frochaux MV, Andreani T, et al. Enteric infection induces Lark-mediated intron retention at the 5ʹ end of Drosophila genes. Genome Biol. 2020;21(1):4.
- Markus MA, Yang YH, Morris BJ Transcriptome-wide targets of alternative splicing by RBM4 and possible role in cancer. Genomics. 2016;107(4):138–144.
- Yong H, Zhao W, Zhou X, et al. RNA-Binding Motif 4 (RBM4) suppresses tumor growth and metastasis in human gastric cancer. Med Sci Monit. 2019;25:4025–4034.
- Lin J-C, Lin C-Y, Tarn W-Y, et al. Elevated SRPK1 lessens apoptosis in breast cancer cells through RBM4-regulated splicing events. Rna. 2014;20(10):1621–1631.
- Wang WY, Quan W, Yang F, et al. RBM4 modulates the proliferation and expression of inflammatory factors via the alternative splicing of regulatory factors in HeLa cells. Mol Genet Genomics. 2020;295(1):95–106.
- Liang YCLW, Lin YJ, Lin JC The impact of RNA binding motif protein 4-regulated splicing cascade on the progression and metabolism of colorectal cancer cells. Oncotarget. 2015;35(35):38046–38060.
- Chang HL, Lin JC SRSF1 and RBM4 differentially modulate the oncogenic effect of HIF-1alpha in lung cancer cells through alternative splicing mechanism. Biochim Biophys Acta Mol Cell Res. 2019;1866(12):118550.
- Gupta A, Kielkopf CL Purification, crystallization and preliminary X-ray crystallographic analysis of a central domain of human splicing factor 1. Acta Crystallographica Section F Structural Biology and Crystallization Communications 2011; 67(4):486–490.
- Crisci A, Raleff F, Bagdiul I, et al. Mammalian splicing factor SF1 interacts with SURP domains of U2 snRNP-associated proteins. Nucleic Acids Res. 2015:gkv952. DOI: https://doi.org/10.1093/nar/gkv952
- Berglund JA, Abovich N, Rosbash M A cooperative interaction between U2AF65 and mBBP/SF1 facilitates branchpoint region recognition. Genes Dev. 1998;12(6):858–867.
- Rino J, Desterro JM, Pacheco TR, et al. Splicing factors SF1 and U2AF associate in extraspliceosomal complexes. Mol Cell Biol 2008;28(9):3045–3057.
- Gupta A, Jenkins JL, Kielkopf CL RNA induces conformational changes in the SF1/U2AF65 splicing factor complex. J Mol Biol 2011;405(5):1128–1138.
- Liu Z, Luyten I, Bottomley MJ, et al. Structural basis for recognition of the intron branch site RNA by splicing factor 1. 2001;294:1098–1102.
- Chatrikhi R, Wang W, Gupta A, et al. SF1 phosphorylation enhances specific binding to U2AF(65) and reduces binding to 3ʹ-splice-site RNA. Biophys J 2016;111(12):2570–2586.
- Wang W, Maucuer A, Gupta A, et al. Structure of Phosphorylated SF1 bound to U2AF65 in an essential splicing factor complex. Structure. 2013;21(2):197–208.
- Lin J-R, Liu Z, Hu J Computational identification of post-translational modification-based nuclear import regulations by characterizing nuclear localization signal-import receptor interaction. Proteins Struct Funct Bioinf. 2014;82(10):2783–2796.
- Corioni M, Antih N, Tanackovic G, et al. Analysis of in situ pre-mRNA targets of human splicing factor SF1 reveals a function in alternative splicing. Nucleic Acids Res. 2011;39(5):1868–1879.
- Shitashige M, Naishiro Y, Idogawa M, et al. Involvement of splicing factor-1 in β-Catenin/T-cell factor-4-mediated gene transactivation and Pre-mRNA splicing. Gastroenterology. 2007;132(3):1039–1054.
- Zhang Z, He X, Liu Q, et al. TGIF1 and SF1 polymorphisms are associated with litter size in Small Tail Han sheep. Reprod Domest Anim. 2020; 55(9):1145–1153.
- Zhu R, Heaney J, Nadeau JH, et al. Deficiency of splicing factor 1 suppresses the occurrence of testicular germ cell tumors. Cancer Res. 2010; 70(18):7264–7272.
- Marintchev A, Wagner G Translation initiation: structures, mechanisms and evolution. Q Rev Biophys. 2004;37(3–4):197.
- Sonenberg N, Hinnebusch AG Regulation of translation initiation in eukaryotes: mechanisms and biological targets. Cell. 2009;136(4):731–745.
- Shatsky IN, Terenin IM, Smirnova VV, et al. Cap-independent translation: what’s in a name? Trends Biochem Sci 2018;43(11):882–895.
- Stoneley M, Willis AE Cellular internal ribosome entry segments: structures, trans-acting factors and regulation of gene expression. Oncogene. 2004;23(18):3200–3207.
- Komar AA, Mazumder B, Merrick WC A new framework for understanding IRES-mediated translation. Gene 2012;502(2):75–86.
- Meyer KD, Patil DP, Zhou J, et al. 5′ UTR m6A promotes cap-independent translation. Cell. 2015; 163(4):999–1010.
- Batista PJ, Molinie B, Wang J, et al. m6A RNA modification controls cell fate transition in mammalian embryonic stem cells. Cell Stem Cell. 2014;15(6):707–719.
- Cui Q, Shi H, Ye P, et al. m6A RNA methylation regulates the self-renewal and tumorigenesis of glioblastoma stem cells. Cell Rep. 2017;18(11):2622–2634.
- Liu N, Dai Q, Zheng G, et al. N 6-methyladenosine-dependent RNA structural switches regulate RNA–protein interactions. Nature. 2015;518(7540):560–564.
- Liu J, Eckert MA, Harada BT, et al. m 6 A mRNA methylation regulates AKT activity to promote the proliferation and tumorigenicity of endometrial cancer. Nat Cell Biol. 2018;20(9):1074–1083.
- Huang H, Weng H, Sun W, et al. Recognition of RNA N(6)-methyladenosine by IGF2BP proteins enhances mRNA stability and translation. Nat Cell Biol. 2018;20(3):285–295.
- Ren W, Lu J, Huang M, et al. Structure and regulation of ZCCHC4 in m 6 A-methylation of 28S rRNA. Nat Commun. 2019;10(1):1–9.
- Pinto R, Vågbø CB, Jakobsson ME, et al. The human methyltransferase ZCCHC4 catalyses N 6-methyladenosine modification of 28S ribosomal RNA. Nucleic Acids Res 2020; 48:830–846. 2
- Braunstein S, Karpisheva K, Pola C, et al. A hypoxia-controlled cap-dependent to cap-independent translation switch in breast cancer. Mol Cell. 2007;28(3):501–512.
- Uniacke J, Holterman CE, Lachance G, et al. An oxygen-regulated switch in the protein synthesis machinery. Nature. 2012;486(7401):126–129.
- Sigismund S, Avanzato D, Lanzetti L Emerging functions of the EGFR in cancer. Mol Oncol. 2018;12(1):3–20.
- J- Y C, L-p L, J- F X Decrease of RBM4 indicates poor prognosis in patients with hepatocellular carcinoma after hepatectomy. Onco Targets Ther. 2017;10:339.
- Kojima S, Matsumoto K, Hirose M, et al. LARK activates posttranscriptional expression of an essential mammalian clock protein, PERIOD1. Proceedings of the National Academy of Sciences, USA. 2007; 104:1859–1864.
- Lin J-C, Tarn W-Y RNA-binding Motif Protein 4 translocates to cytoplasmic granules and suppresses translation via Argonaute2 during muscle cell differentiation. J Biol Chem. 2009;284(50):34658–34665.
- Brudecki L, Ferguson DA, McCall CE, et al. MicroRNA‐146a and RBM4 form a negative feed‐forward loop that disrupts cytokine mRNA translation following TLR4 responses in human THP‐1 monocytes. Immunol Cell Biol 2013;91(8):532–540.
- Armas P, Nasif S, Calcaterra NB Cellular nucleic acid binding protein binds G‐rich single‐stranded nucleic acids and may function as a nucleic acid chaperone. J Cell Biochem. 2008;103(3):1013–1036.
- Huichalaf C, Schoser B, Schneider-Gold C, et al. Reduction of the rate of protein translation in patients with myotonic dystrophy 2. J Neurosci. 2009;29(28):9042–9049.
- Antonucci L, D’Amico D, Di Magno L, et al. CNBP regulates wing development in Drosophila melanogaste by promoting IRES-dependent translation of dMyc. Cell Cycle. 2014;13(3):434–439.
- Rojas M, Farr GW, Fernandez CF, et al. Yeast Gis2 and its human ortholog CNBP are novel components of stress-induced RNP granules. PLoS One. 2012;7(12):e52824.
- Houseley J, Tollervey D The many pathways of RNA degradation. Cell. 2009;136(4):763–776.
- Lim J, Ha M, Chang H, et al. Uridylation by TUT4 and TUT7 marks mRNA for degradation. Cell. 2014;159(6):1365–1376.
- Sha QQ, Zhu YZ, Li S, et al. Characterization of zygotic genome activation-dependent maternal mRNA clearance in mouse. Nucleic Acids Res. 2020;48(2):879–894.
- Sha QQ, Zheng W, Wu YW, et al. Dynamics and clinical relevance of maternal mRNA clearance during the oocyte-to-embryo transition in humans. Nat Commun. 2020;11(1):4917.
- Morgan M, Kabayama Y, Much C, et al. A programmed wave of uridylation-primed mRNA degradation is essential for meiotic progression and mammalian spermatogenesis. Cell Res. 2019;29(3):221–232.
- Warkocki Z, Krawczyk PS, Adamska D, et al. Uridylation by TUT4/7 restricts retrotransposition of human LINE–1s. Cell. 2018;174(6):1537–48.e29.
- Schmidt MJ, West S, Norbury CJ The human cytoplasmic RNA terminal U-transferase ZCCHC11 targets histone mRNAs for degradation. RNA. 2011;17(1):39–44.
- Mullen TE, Marzluff WF Degradation of histone mRNA requires oligouridylation followed by decapping and simultaneous degradation of the mRNA both 5′ to 3′ and 3′ to 5′. Genes & Development. 2008;22(1):50–65.
- Lackey PE, Welch JD, Marzluff WF TUT7 catalyzes the uridylation of the 3′ end for rapid degradation of histone mRNA. Rna. 2016;22(11):1673–1688.
- Hagan JP, Piskounova E, Gregory RI Lin28 recruits the TUTase Zcchc11 to inhibit let-7 maturation in mouse embryonic stem cells. Nat Struct Mol Biol. 2009;16(10):1021–1025.
- Thornton JE, Chang HM, Piskounova E, et al. Lin28-mediated control of let-7 microRNA expression by alternative TUTases Zcchc11 (TUT4) and Zcchc6 (TUT7). RNA. 2012; 18(10):1875–1885.
- Heo I, Ha M, Lim J, et al. Mono-uridylation of pre-microRNA as a key step in the biogenesis of group II let-7 microRNAs. Cell. 2012;151(3):521–532.
- Heo I, Joo C, Kim Y-K, et al. TUT4 in concert with Lin28 suppresses MicroRNA biogenesis through Pre-MicroRNA Uridylation. Cell. 2009;138(4):696–708.
- Faehnle CR, Walleshauser J, Joshua-Tor L Multi-domain utilization by TUT4 and TUT7 in control of let-7 biogenesis. Nat Struct Mol Biol. 2017;24(8):658–665.
- Yamashita S, Nagaike T, Tomita K Crystal structure of the Lin28-interacting module of human terminal uridylyltransferase that regulates let-7 expression. Nat Commun. 2019;10(1):1960.
- Piskounova E, Viswanathan SR, Janas M, et al. Determinants of microRNA processing inhibition by the developmentally regulated RNA-binding protein Lin28. J Biol Chem. 2008;283(31):21310–21314.
- Ustianenko D, Chiu HS, Treiber T, et al. LIN28 selectively modulates a subclass of Let–7 MicroRNAs. Mol Cell. 2018;71(2):271–83 e5.
- Rybak A, Fuchs H, Smirnova L, et al. A feedback loop comprising lin-28 and let-7 controls pre-let-7 maturation during neural stem-cell commitment. Nat Cell Biol. 2008;10(8):987–993.
- Johnson CD, Esquela-Kerscher A, Stefani G, et al. The let-7 MicroRNA represses cell proliferation pathways in human cells. Cancer Res. 2007;67(16):7713–7722.
- Sampson VB, Rong NH, Han J, et al. MicroRNA let-7a down-regulates MYC and reverts MYC-induced growth in Burkitt lymphoma cells. Cancer Res. 2007;67(20):9762–9770.
- Takamizawa J, Konishi H, Yanagisawa K, et al. Reduced expression of the let-7 MicroRNAs in human lung cancers in association with shortened postoperative survival. Cancer Res. 2004;64(11):3753–3756.
- Dou J, Zhang H, Chen R, et al. SUMOylation modulates the LIN28A-let-7 signaling pathway in response to cellular stresses in cancer cells. Mol Oncol. 2020;14(9):2288–2312.
- Yu C, Wang L, Rowe RG, et al. A nanobody targeting the LIN28: let-7interaction fragment of TUT4 blocks uridylation of let-7. Proc Natl Acad Sci U S A. 2020;117(9):4653–4663.
- Kim B, Ha M, Loeff L, et al. TUT 7 controls the fate of precursor micro RNAs by using three different uridylation mechanisms. Embo J. 2015;34(13):1801.
- Jones MR, Blahna MT, Kozlowski E, et al. Zcchc11 uridylates mature miRNAs to enhance neonatal IGF-1 expression, growth, and survival. PLoS Genet. 2012;8(11):e1003105.
- Jones MR, Quinton LJ, Blahna MT, et al. Zcchc11-dependent uridylation of microRNA directs cytokine expression. Nat Cell Biol. 2009;11(9):1157–1163.
- Ansari MY, Khan NM, Ahmad N, et al. Genetic inactivation of ZCCHC 6 suppresses Interleukin-6 expression and reduces the severity of experimental osteoarthritis in Mice. Arthritis Rheumatol. 2019;71(4):583–593.
- Yang A, Shao TJ, Bofill-De Ros X, et al. AGO-bound mature miRNAs are oligouridylated by TUTs and subsequently degraded by DIS3L2. Nat Commun. 2020;11(1):2765.
- Kim H, Kim J, Yu S, et al. A mechanism for microRNA arm switching regulated by Uridylation. Mol Cell. 2020;78(6):1224–36 e5.
- Heo I, Joo C, Cho J, et al. Lin28 mediates the terminal uridylation of let-7 precursor MicroRNA. Mol Cell. 2008;32(2):276–284.
- Houseley J, Tollervey D The nuclear RNA surveillance machinery: the link between ncRNAs and genome structure in budding yeast? Biochim Biophys Acta Gene Regul Mech. 2008;1779(4):239–246.
- Schmidt K, Butler JS Nuclear RNA surveillance: role of TRAMP in controlling exosome specificity. Wiley Interdiscip Rev RNA. 2013;4(2):217–231.
- Lubas M, Christensen Marianne S, Kristiansen Maiken S, et al. Interaction profiling identifies the human nuclear exosome targeting complex. Mol Cell. 2011;43(4):624–637.
- Chlebowski A, Lubas M, Jensen TH, et al. RNA decay machines: the exosome. Biochim Biophys Acta Gene Regul Mech. 2013;1829(6–7):552–560.
- Shcherbik N, Wang M, Lapik YR, et al. Polyadenylation and degradation of incomplete RNA polymerase I transcripts in mammalian cells. EMBO Rep. 2010;11(2):106.
- Sudo H, Nozaki A, Uno H, et al. Interaction properties of human TRAMP-like proteins and their role in5ʹETS turnover. FEBS Lett. 2016;590(17):2963–2972.
- Potesil D, Pasulka J, Zdrahal Z, et al. RBM7 subunit of the NEXT complex binds U-rich sequences and targets 3′-end extended forms of snRNAs. Nucleic Acids Res. 2015;43(8):4236–4248.
- Andersen PR, Domanski M, Kristiansen MS, et al. The human cap–binding complex is functionally connected to the nuclear RNA exosome. Nature Structural Amp Molecular Biology 2013; 20(12):1367.
- Wu Y, Liu W, Chen J, et al. Nuclear exosome targeting complex core factor Zcchc8 regulates the degradation of LINE1 RNA in early embryos and embryonic stem cells. Cell Rep. 2019;29(8):2461–72 e6.
- Falk S, Finogenova K, Melko M, et al. Structure of the RBM7-ZCCHC8 core of the NEXT complex reveals connections to splicing factors. Nat Commun. 2016;7(1):13573.
- Guo TB, Boros LG, Chan KC, et al. Spermatogenetic expression of RNA-Binding Motif Protein 7, a protein that interacts with splicing factors. J Andrology. 2003;24(2):204–214.
- Lingaraju M, Johnsen D, Schlundt A, et al. The MTR4 helicase recruits nuclear adaptors of the human RNA exosome using distinct arch-interacting motifs. Nat Commun. 2019; 10(1):3393.
- West J, Viswanathan S, Yabuuchi A, et al. A role for Lin28 in primordial germ-cell development and germ-cell malignancy. Nature. 2009;460(7257):909–913.
- Thornton JE, Gregory RI How does Lin28 let-7 control development and disease? Trends Cell Biol. 2012; 22(9):474–482.
- Velie BD, Shrestha M, Franois L, et al. Using an inbred horse breed in a high density genome-wide scan for genetic risk factors of Insect Bite Hypersensitivity (IBH). PLoS One. 2016;11(4):e0152966.
- Ziegler A, Ewhida A, Brendel M, et al. More powerful haplotype sharing by accounting for the mode of inheritance. Genet Epidemiol. 2009;33(3):228–236.
- Doxtader KA, Wang P, Scarborough AM, et al. Structural basis for regulation of METTL16, an S–Adenosylmethionine homeostasis factor. Mol Cell. 2018;71(6):1001–11 e4.
- Ma H, Wang X, Cai J, et al. N6-Methyladenosine methyltransferase ZCCHC4 mediates ribosomal RNA methylation. Nat Chem Biol 2019.; 15(1):88–94
- Alonso-Martin S, Rochat A, Mademtzoglou D, et al. Gene expression profiling of muscle stem cells identifies novel regulators of postnatal myogenesis. Front Cell Dev Biol. 2016;4:58.
- Kozlowski E, Wasserman GA, Morgan M, et al. The RNA uridyltransferase Zcchc6 is expressed in macrophages and impacts innate immune responses. PLoS One. 2017;12(6):e0179797.
- Gao Y, Liu X, Tang B, et al. Protein expression landscape of mouse embryos during pre-implantation development. Cell Rep. 2017;21(13):3957–3969.
- Pyeon D, Newton MA, Lambert PF, et al. Fundamental differences in cell cycle deregulation in human papillomavirus-positive and human papillomavirus-negative head/neck and cervical cancers. Cancer Res. 2007;67(10):4605–4619.
- Fu X, Meng Z, Liang W, et al. miR-26a enhances miRNA biogenesis by targeting Lin28B and Zcchc11 to suppress tumor growth and metastasis. Oncogene. 2014;33(34):4296–4306.
- Ginam C, Bhat SS, Jinsong G, et al. Evidence that SIZN1 is a candidate X-linked mental retardation gene. Am J Med Genet Part A. 2010;146A:2644–2650.
- Handrigan GR, Chitayat D, Lionel AC, et al. Deletions in 16q24.2 are associated with autism spectrum disorder, intellectual disability and congenital renal malformation. J Med Genet. 2013;50(3):163–173.
- Hyrina A, Jones C, Chen D, et al. A Genome–wide CRISPR screen identifies ZCCHC14 as a host factor required for Hepatitis B surface antigen production. Cell Rep. 2019;29(10):2970–8 e6.
- Li DL, Ye L, Gao L, et al. Plasma concentration of ZCCHC14 contributes to prognostic efficacy in intracerebral hemorrhage patients. Eur Rev Med Pharmacol Sci. 2019;23(19):8533–8539.
- Irie M, Yoshikawa M, Ono R, et al. Cognitive function related to the Sirh11/Zcchc16 gene acquired from an LTR Retrotransposon in Eutherians. PLoS Genet. 2015;11(9):e1005521.
- Wang H, Liu XL, Shu SN, et al. Identification of proteins that interact with murine cytomegalovirus early protein M112-113 in brain. Chin Med J (Engl). 2011;124(21):3532–3536.
- Tan YW, Hong W, Liu DX Binding of the 5ʹ-untranslated region of coronavirus RNA to zinc finger CCHC-type and RNA-binding motif 1 enhances viral replication and transcription. Nucleic Acids Res. 2012;40(11):5065–5077.
- Sammons MA, Samir P, Link AJ Saccharomyces cerevisiae Gis2 interacts with the translation machinery and is orthogonal to myotonic dystrophy type 2 protein ZNF9. Biochem Biophys Res Commun. 2011;406(1):13–19.
- Vitorino M, Correia E, Serralheiro AR, et al. Expression pattern of zcchc24 during early Xenopus development. Int J Dev Biol. 2014;58(1):45–50.