ABSTRACT
Methylation is a common post-transcriptional modification of tRNAs, particularly in the anticodon loop region. The cytosine 38 (C38) in tRNAs, such as tRNAAsp-GUC, tRNAGly-GCC, tRNAVal-AAC, and tRNAGlu-CUC, can be methylated by human DNMT2/TRDMT1 and some homologs found in bacteria, plants, and animals. However, the substrate properties and recognition mechanism of DNMT2/TRDMT1 remain to be explored. Here, taking into consideration common features of the four known substrate tRNAs, we investigated methylation activities of DNMT2/TRDMT1 on the tRNAGly-GCC truncation and point mutants, and conformational changes of mutants. The results demonstrated that human DNMT2/TRDMT1 preferred substrate tRNAGly-GCC in vitro. L-shaped conformation of classical tRNA could be favourable for DNMT2/TRDMT1 activity. The complete sequence and structure of tRNA were dispensable for DNMT2/TRDMT1 activity, whereas T-arm was indispensable to this activity. G19, U20, and A21 in D-loop were identified as the important bases for DNMT2/TRDMT1 activity, while G53, C56, A58, and C61 in T-loop were found as the critical bases. The conserved CUXXCAC sequence in the anticodon loop was confirmed to be the most critical determinant, and it could stabilize C38-flipping to promote C38 methylation. Based on these tRNA properties, new substrates, tRNAVal-CAC and tRNAGln-CUG, were discovered in vitro. Moreover, a single nucleotide substitute, U32C, could convert non-substrate tRNAAla-AGC into a substrate for DNMT2/TRDMT1. Altogether, our findings imply that DNMT2/TRDMT1 relies on a delicate network involving both the primary sequence and tertiary structure of tRNA for substrate recognition.
Introduction
The tRNA modification reportedly contributes to the subcellular localization and stability of tRNACitation[1,Citation2] and the fidelity and efficiency of protein translation [Citation3,Citation4]. Moreover, 172 different modifications of RNA are currently listed in the ‘Modomics’ database, 90 of which are determined in tRNAs, particularly in the anticodon loop regions [Citation5–7]. Strikingly, methylation is a common post-transcriptional modification of tRNAs [Citation5]. The cytosine 38 (C38) in tRNAs, such as tRNAAsp-GUC, tRNAGly-GCC, tRNAVal-AAC, and tRNAGlu-CUC, can be methylated by human DNMT2/TRDMT1 and some homologs found in bacteria, plants, and animals [Citation2,Citation8,Citation9]. Notably, DNMT2/TRDMT1 is the most conserved member of the DNMT family, and C38 also exists in many tRNA molecules. Thus, new tRNA substrates need to be further investigated.
Previous studies have reported that the activities of DNMT2/TRDMT1 homologs, Pmt1 in Schizosaccharomyces pombe (S. pombe) and DnmA in Dictyostelium discoideum (D. discoideum), are significantly enhanced after queuosine (Q) 34 modification of tRNAAsp-GUC [Citation10,Citation11]. Moreover, the 5ʹ-tRNAAsp-GUC half effectively guides DNMT2/TRDMT1 to methylate single-stranded tRNA fragments derived from nuclease cleavage [Citation12]. Additionally, the variable loops of all preferred DNMT2/TRDMT1 substrates (tRNAAsp-GUC from all species except tRNAGlu-UUC in Geobacter sulfurreducens) have at least one nucleotide A at the site 45 or 46, but all the inactive counterparts contain a dinucleotide GG [Citation8]. Surprisingly, the variable loop of murine tRNAGlu-UUC is replaced by the corresponding part from murine tRNAAsp-GUC, which does not dramatically increase human DNMT2/TRDMT1 activity [Citation8]. Therefore, the variable loop acts as an important determinant for DNMT2/TRDMT1 activity. Nevertheless, the other substrate properties and recognition mechanism of DNMT2/TRDMT1 remain to be researched.
CD spectroscopy is a practical technique for exploring subtle conformational changes of modifications and mutations of tRNA in structural analyses, especially when crystallography and NMR techniques are not available [Citation13,Citation14]. The standard tRNA molecule is characterized by an L-shaped tertiary structure composed of two perpendicularly arranged double-helical domains (acceptor and T-stem domain, and anticodon- and D-stem domain) [Citation15,Citation16]. CD spectrum of normal tRNA is a typical A-form helix, where there are four major peaks (two negative peaks near 210 and 240 nm, and two positive peaks near 223 and 265 nm) [Citation17]. As reported earlier, A conformation is characterized by the formations of intense negative and positive peaks, near 210 and 265 nm, respectively [Citation18]. DNA methyltransferase-like catalysis mechanism mediated by DNMT2/TRDMT1 and a surface cleft accommodating the anticodon stem and loop in the protein structure have been discovered through the site-directed mutagenesis of DNMT2/TRDMT1 [Citation19,Citation20]. Therefore, it is necessary to determine key tRNA elements which maintain the conformational stability of tRNA structure and affect the DNMT2/TRDMT1 recognition via CD spectroscopy analysis of tRNA mutants.
The tRNA recognition pattern of DNMT2/TRDMT1, especially how this enzyme discriminates tRNA substrates and whether it modifies other tRNAs, is an interesting topic. Although crystal structures of DNMT2/TRDMT1 homologs in human, Entamoeba histolytica (E. histolytica), Spodoptera frugiperda (S. frugiperda), and S. pombe have been analysed, the DNMT2/TRDMT1-tRNA complex structure has been largely unknown. Hence, it is difficult to reveal the recognition mechanism of DNMT2/TRDMT1 on tRNA. This study revealed substrate tRNA properties based on DNMT2/TRDMT1 activities on tRNAGly-GCC mutants, and conformational changes of mutants. We identified new substrates tRNAVal-CAC and tRNAGln-CUG in vitro, and elucidated the conversion of non-substrate tRNAAla-AGC into a substrate for DNMT2/TRDMT1 through a single nucleotide substitution (U32C). Our findings suggest that DNMT2/TRDMT1 depends on a delicate network involving tRNA primary sequence and tertiary structure for substrate recognition.
Results
Methylation preference of DNMT2/TRDMT1
Although purified human DNMT2/TRDMT1 has weak methylation activity at the CG site of ttnCGga(g/a) sequence, the enzymatic activity on DNA remains controversial in vivo [Citation21]. In recent years, the RNA methyltransferase activity of DNMT2/TRDMT1 has been confirmed, and new substrates have also been continuously discovered [Citation2,Citation8,Citation9]. To clarify the structural mechanism by which DNMT2/TRDMT1 binds tRNA substrate, tertiary L-shaped structure of human tRNAGly-GCC was first modelled according to conserved tertiary interactions and the predicted secondary structure. The result demonstrated that the conserved tertiary interactions except G18-U55 existed in nonmodified tRNAGly-GCC structure (). The tertiary interaction G18-U55 was disrupted in the tRNA structure, which might be attributed to the lack of in vivo natural modification at sites 18, 55, and elsewhere [Citation22]. As displayed in the docked DNMT2/TRDMT1-tRNA complex, the anticodon stem and loop electrostatically interacted with a positive contact potential cleft of the DNMT2/TRDMT1 surface, while D-stem and loop came into contact with the negative potential surface of DNMT2/TRDMT1 ().
Figure 1. Human DNMT2/TRDMT1-tRNA complex structure and DNMT2/TRDMT1 methylation preference for tRNA substrates. A. Tertiary L-shaped nucleotide sequence representation of human tRNAGly-GCC predicted via RNAComposer. The tertiary interactions were conservative in all the cytoplasmic tRNAs and highlighted in dashed lines. B. Tertiary L-shaped structure of human tRNAGly-GCC presented in a cartoon by PyMOL 1.7.0. Structural domains of tRNAGly-GCC were indicated by different colours. C. Human DNMT2/TRDMT1-tRNA complex structure. tRNAGly-GCC was docked into the crystal structure of human DNMT2/TRDMT1 (PDB ID: 1G55) by a web server, HDOCK. The protein contact potential of DNMT2/TRDMT1 was calculated by PyMOL 1.7.0. S-adenosyl-L-homocysteine (SAH) and cytosine 38 (C38) were shown in sticks. D. Bar graph of 3H incorporated into different tRNA substrates by DNMT2/TRDMT1 at 70 min. E. Hyperbola plot of methylation activities of DNMT2/TRDMT1 on tRNAGly-GCC calculated using Michaelis-Menten equation. Data were expressed as the mean ± SD from three independent measurements
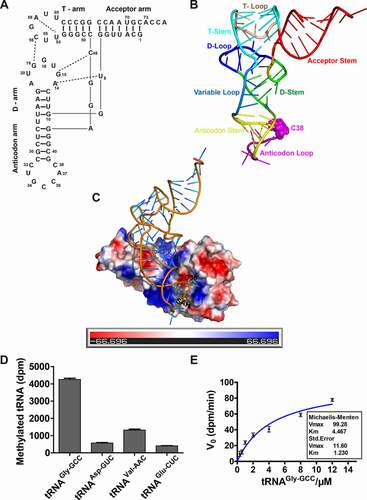
The purity of GST-DNMT2/TRDMT1 and His-DNMT2/TRDMT1 was more than 95%, and the enzymes were subsequently used in enzymatic assays (Figure S1 A). As shown in Figure S1 B, His-DNMT2/TRDMT1 activity was significantly improved compared to GST-DNMT2/TRDMT1 activity. Thus, His-DNMT2/TRDMT1 was applied for the subsequent activity detection. Four tRNA substrates (tRNAAsp-GUC, tRNAGly-GCC, tRNAVal-AAC, and tRNAGlu-CUC) of DNMT2/TRDMT1 homologs were transcribed by T7 RNA polymerase to explore the methylation preference of DNMT2/TRDMT1 (Figure S2). Their cloverleaf structures were shown in Figure S3 A. The activity characterization of human DNMT2/TRDMT1 on these substrates was revealed despite the lack of native post-transcriptional modifications, demonstrating that human DNMT2/TRDMT1 preferred substrate tRNAGly-GCC in vitro ( and Figure S3 B). It should be noted that the Km value of human DNMT2/TRDMT1 on tRNAGly-GCC transcript was 4.5 ± 1.2 μM, and the kcat value was 0.268 ± 0.031 s−1 (), which was similar to the reported Km (4.2 μM) of this enzyme on tRNAAsp-GUC [Citation12]. Due to the low concentration of purchased 3H-labelled S-adenosine, only a similar kcat/Km experimental condition was applied to determine the kinetic characterization of DNMT2/TRDMT1 on tRNA mutant in the following assay [Citation8,Citation20,Citation23].
Kinetic characterization of deletion mutants of tRNAGly-GCC
In crystal structures of tRNATrp-CCA mutants, A9C mutation enhances the tRNA flexibility and G24A mutation forms an additional hydrogen bond, which indeed distorts the native tRNA conformation, resulting in the miscoding in the process of protein translation [Citation24]. We performed a sequence alignment of tRNA substrates to investigate determinative elements in the tRNA structure for the DNMT2/TRDMT1 activity. The results demonstrated that a large number of conserved bases were located in the D-loop (A14, G15, U16, G18, G19, U20, and A21), T-loop (G53, U54, U55, C56, A58, and C61), and anticodon loop (C32, U33, C36, A37, and C38) (). Hence, point mutations and deletion mutations of tRNAGly-GCC were conducted (). Subsequently, methylation activities of DNMT2/TRDMT1 on these mutants were detected.
Figure 2. Sequence conservation of tRNA substrates and tRNAGly-GCC mutant structures. A. Sequence alignment of tRNA substrates of human DNMT2/TRDMT1 under ‘default’ mode by ClustalW multiple sequence alignment program in CLUSTAL X (2.0.12) software. B. Cloverleaf structures of tRNAGly-GCC mutants predicted by tRNAscan-SE 2.0
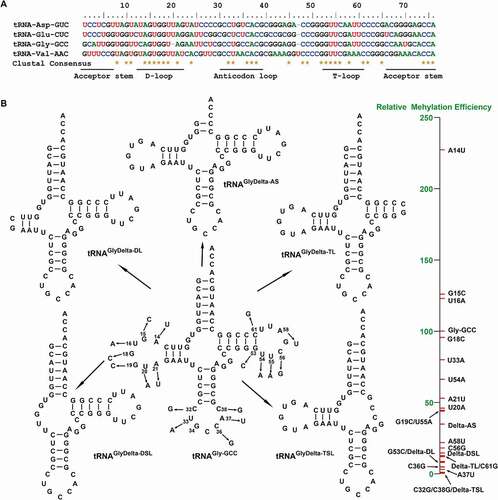
The RNA methyltransferase activity is closely related to the primary sequence and tertiary structure of substrate tRNAs. The truncated mutants of acceptor arm, D-loop, D-stem loop, T-loop, and T-stem loop in tRNAGly-GCC were synthesized (Figure S2) and named as tRNAGlyDelta-AS, tRNAGlyDelta-DL, tRNAGlyDelta-DSL, tRNAGlyDelta-TL, and tRNAGlyDelta-TSL, respectively (). As shown in and Figure S4 A, the enzymatic activity result demonstrated that DNMT2/TRDMT1 could still methylate tRNAGlyDelta-AS, but the transfer rate of 3H to tRNA was decreased, and the transfer amount was about one-third of wild-type tRNAGly-GCC. Besides, the amount and rate of 3H transfer to tRNAGlyDelta-DL were slightly lower than those to tRNAGlyDelta-TL by DNMT2/TRDMT1, about 1/10 of those to the wild-type, indicating that D- and T-loops might play more important roles than acceptor arm in the methylation catalysis of DNMT2/TRDMT1. After the further deletion of the D-stem loop, the DNMT2/TRDMT1 activity on tRNAGlyDelta-DSL was slightly higher than on tRNAGlyDelta-DL, and still far lower than on wild-type tRNAGly-GCC. Interestingly, following the T-stem loop truncation, DNMT2/TRDMT1 lost enzymatic activity on tRNAGlyDelta-TSL, indicating that the T-stem base pair was essential for this activity. Overall, the importance of tRNA structural elements on DNMT2/TRDMT1 activities was in the following order: acceptor arm < D-stem loop < T-loop < D-loop < T-stem loop.
Figure 3. Methylation and structural characterizations of tRNAGly-GCC deletion mutants. A. Bar graph of 3H incorporated into tRNAGly-GCC and its deletion mutants by DNMT2/TRDMT1 at 70 min. B. CD spectra of tRNAGly-GCC deletion mutants. C. Tertiary structures of tRNAGly-GCC deletion mutants predicted. Data were expressed as the mean ± SD from three independent measurements
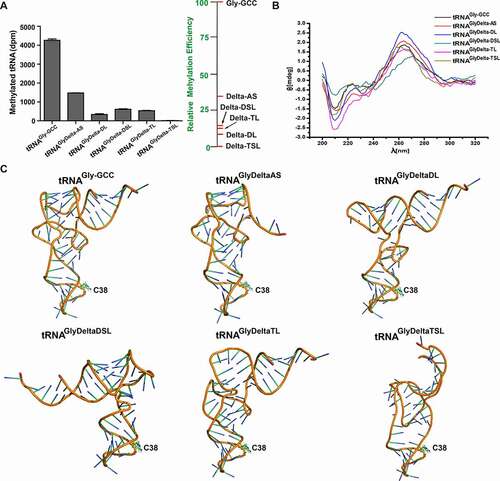
CD spectroscopy is widely applied to investigate the folding and conformational changes induced by tRNA modification and mutation. The formations of positive peak near 265 nm and negative peak near 240 nm are due to the exciton splitting of p-p* transitions caused by base pairing and stacking, while the negative peak at 208 nm is formed by the n-π* transition of nucleotide base [Citation25,Citation26]. Notably, the CD spectrum results showed that the four peaks at 208, 223, 240, and 265 nm were significantly changed, indicating that structural conformations of deletion mutants were altered (). Deletion mutations, especially TSL mutations, exerted no effect on anticodon loop conformations, but significantly affected the other structures of tRNA, which could be related to the decreased activity or inactivation of DNMT2/TRDMT1 (). The negative peak of DSL mutant at 208 nm was decreased, and the red shift of positive peak at 265 nm was observed (). These CD spectrum changes responded to the distortion of the receptor arm and T-stem (). Consequently, the L-shaped conformation of classical tRNA could be important for DNMT2/TRDMT1 activity. DNMT2/TRDMT1 did not require a complete sequence and structure of tRNA to maintain its activities, but T-arm was essential.
Kinetic characterization of D-loop mutants of tRNAGly-GCC
The important bases in D- and T-loops of tRNA can determine the catalytic activities and substrate recognition of some RNA methyltransferases, such as TrmH, TrmY, and Trm5 [Citation27–30]. Considering the conserved bases in D-loop and DNMT2/TRDMT1 activity on tRNAGlyDelta-DL, the point mutants, including A14U, G15C, U16A, G18C, G19C, U20A, and A21U, were transcriptionally synthesized (Figure S2). As shown in and Figure S4 B, DNMT2/TRDMT1 activity result demonstrated that the transfer amount and rate of 3H to G18C mutant were similar to wild-type tRNAGly-GCC. However, both the transfer amount and rate of 3H to A14U, G15C, and U16A mutants were enhanced, and especially those to A14U mutant were significantly up-regulated, to about twice the level of wild-type. By contrast, G19C, U20A, and A21U mutants reduced the transfer amount and rate of 3H, to about 50% of the level of wild-type tRNAGly-GCC. Hence, these results further support our above-mentioned result of DNMT2/TRDMT1 activity on D-loop-deleted mutant ().
Figure 4. Methylation and structural characterizations of tRNAGly-GCC D-loop mutants. A. Bar graph of 3H incorporated into tRNAGly-GCC mutants in D-loop by DNMT2/TRDMT1 at 70 min. B. CD spectra of tRNAGly-GCC D-loop mutants. C. Tertiary structures of tRNAGly-GCC D-loop mutants predicted. Data were expressed as the mean ± SD from three independent measurements
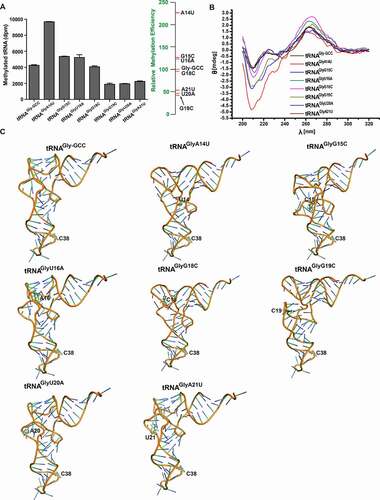
Conserved tertiary interactions of G19-C56, G15-C48, and A14-U8 in all the cytoplasmic tRNAs are crucial for the constitution of the tRNA elbow and the stabilization of close contact between D- and T-loops [Citation16]. Thus, A14U, G15C, U16A, G18C, and G19C mutations caused tRNA conformational changes, which might enhance the negative peak at 208 nm and significantly alter the other three peaks (). Additionally, the peaks of U20A and A21U mutants at 208 nm or 265 nm exhibited almost no changes, which might be due to a weak interaction between U20 or A21 and T-loop (). D-loop point mutations changed the tRNA whole conformation. However, the conformations of anticodon stem and loop were not altered, which could explain why DNMT2/TRDMT1 still had methylation activity on D-loop mutants. Importantly, conformational changes induced by A14U, G15C, and U16A mutations rendered tRNAGly-GCC a better substrate for DNMT2/TRDMT1. Conversely, G19 C, U20A, and A21U mutations made the tRNA conformation detrimental to the DNMT2/TRDMT1 catalysis.
Kinetic characterization of T-loop mutants of tRNAGly-GCC
T-arm plays a crucial role in some RNA methyltransferase activities. For instance, tRNAVal or tRNAPhe T-arm fragments, comprising the 7-base T-loop and 2–5 base-pair stem, can be discriminated and modified by E. coli tRNA m5U54 methyltransferase [Citation31]. Considering conserved bases in T-loop and DNMT2/TRDMT1 activity on tRNAGlyDelta-TL, G53C, U54A, U55A, C56G, A58U, and C61G mutants were transcribed in vitro (Figure S2). Methylation activity results showed that DNMT2/TRDMT1 dramatically down-regulated the transfer rate and total amount of 3H to T-loop mutants in the order: wild-type > U54A > U55A > A58U > C56G > C61G > G53C ( and Figure S4 C). Most strikingly, G53C mutation attenuated DNMT2/TRDMT1 activity by 12-folds. These results also confirmed our above DNMT2/TRDMT1 activity on T-loop-truncated mutant (). In conclusion, conserved bases G53, C56, A58, and C61 in T-loop were very important for the recognition and catalysis of DNMT2/TRDMT1.
Figure 5. Methylation and structural characterizations of tRNAGly-GCC T-loop mutants. A. Bar graph of 3H incorporated into tRNAGly-GCC mutants in T-loop by DNMT2/TRDMT1 at 70 min. B. CD spectra of tRNAGly-GCC T-loop mutants. C. Tertiary structures of tRNAGly-GCC T-loop mutants predicted. Data were expressed as the mean ± SD from three independent measurements
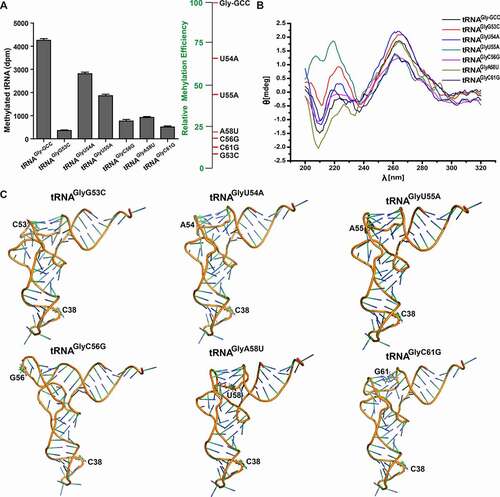
Watson–Crick pair G19-C56 assembles the outermost corner of the tRNA elbow to maintain D- and T-loop interaction, while reverse-Hoogsteen pair U54-A58 stabilizes sharp bending at bases 55–57 in T-loop [Citation32]. The peaks of D-loop mutants at 208 nm were enhanced compared with that of wild-type tRNAGly-GCC, but the peaks of T-loop mutants were weakened, which might be directly related to the interaction between D- and T-loops (). Like D-loop mutation, T-loop mutation did not cause structural changes of anticodon stem and loop, but significantly affected the conformations of T- and D-loops (). The base-stacking force is mainly responsible for maintaining the stability of the tRNA three-dimensional (3D) structure [Citation33]. Compared to that of wild-type tRNAGly-GCC, the stacking force of the T-loop mutant was significantly attenuated to make the tRNA structure unstable, which might elucidate why DNMT2/TRDMT1 activity on T-loop mutant was considerably reduced. G53C or C61G mutation caused the disappearance of the G53-C61 base pair, which further shortened the T-stem and reduced base stacking, thus leading to a weakened peak at 208 nm (). Therefore, conformation and spectroscopy changes of T-loop mutants were closely related to the decreased DNMT2/TRDMT1 activities.
Kinetic characterization of anticodon loop mutants of tRNAGly-GCC
Since the anticodon is responsible for base pairing to mRNA codon under a unique conformation of native tRNA, C38 methylation in the anticodon loop can strongly affect the accuracy and efficiency of protein translation through DNMT2/TRDMT1 [Citation3,Citation4]. The anticodon stem-loop closely interacted with DNMT2/TRDMT1 (), and thus the anticodon loop might be of utmost importance for DNMT2/TRDMT1 recognition. C32G, U33A, C36G, A37U, and C38G mutants were synthesized via in vitro transcription (Figure S2). Methylation activity results showed that all the anticodon loop mutations inhibited the transfer amount and rate of 3H, and that enzymatic activities on these mutants were down-regulated in the following order: wild-type >U33A > C36G > A37U > C32G = C38G ( and Figure S4 D). Among them, U33A mutation decreased the transfer amount and rate of 3H to about three-fourths of wild-type tRNAGly-GCC. However, the transfer amount and rate of 3H could be significantly suppressed by C32G, C36G, A37U, and C38G mutations. Particularly, enzymatic activities on C36G and A37U mutants decreased by about 1/172 folds, compared to that on the wild-type. Besides, both C32G and C38G mutations resulted in the loss of DNMT2/TRDMT1 activities. Hence, the mutation of C38 (the very target cytosine of DNMT2/TRDMT1) could be regarded as a negative control in the tRNA methylation assay.
Figure 6. Methylation and structural characterizations of tRNAGly-GCC anticodon loop mutants. A. Bar graph of 3H incorporated into tRNAGly-GCC mutants in anticodon loop by DNMT2/TRDMT1 at 70 min. B. CD spectra of tRNAGly-GCC anticodon loop mutants. C. Tertiary structures of tRNAGly-GCC anticodon loop mutants predicted. Hydrogen bond interactions between C38 and mutational bases were indicated in yellow dashed lines. Data were expressed as the mean ± SD from three independent measurements
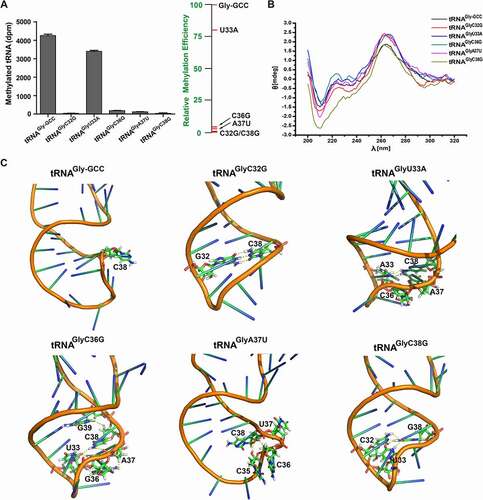
CD spectrum results of these mutants further demonstrated that the positive peak at 265 nm and the negative peak at 208 nm were enhanced, indicating that tRNA structural conformations were affected (). However, CD spectrum changes induced by anticodon loop mutations were weaker than those induced by D- and T-loop mutations. This might be because the bases in the anticodon loop were away from the tRNA structure centre (). Modifications near anticodon bases, such as those at positions 32, 37, 38, and 39, can maintain the 3D structural stabilization of the anticodon loop by restraining functional nonessential intra-loop base pairing [Citation34]. In the present study, the anticodon loop mutations mainly altered the conformation of the anticodon loop and contributed to intra-loop base interactions, causing C38 or G38 to twist into the loop, eventually resulting in the decrease or loss of DNMT2/TRDMT1 activities ( and Figure S5). C32G or C38G mutation contributed to the formation of Watson-Crick pair C32-G38 or G32-C38, and then the anticodon stem was prolonged, which eliminated DNMT2/TRDMT1 activity on C32G or C38G mutant (). Overall, U33, C36, A37, and the unpaired C32-C38 (CUXXCAC sequence) in the anticodon loop were key determinants for this enzyme activity on tRNAGly-GCC.
tRNAVal-CAC and tRNAGln-CUG as new substrates of DNMT2/TRDMT1 in vitro
The tRNA binding site in the DNMT2/TRDMT1 structure has been mapped through the activity and binding detection of enzyme mutants according to electrostatic interactions between positively charged residues of protein surface and the phosphodiester backbone of tRNA [Citation20]. Moreover, DNMT2/TRDMT1 activity on short single-stranded DNA has been detected in vitro by reducing tRNA contents and gradually converting tRNA into DNA [Citation12]. To identify new substrates of DNMT2/TRDMT1, six human C38-containing tRNAs with five conserved bases (tRNAVal-CAC), four conserved bases (tRNAAla-AGC, tRNAGln-CUG, and tRNALys-UUU) or three conserved bases (tRNALeu-AAG and tRNAHis-GUG) were transcribed according to CUXXCAC sequence in the anticodon loop of known substrate tRNA (Figure S2). Coverleaf structures of these tRNAs were shown in Figure S6 A. In the present study, DNMT2/TRDMT1 had no methylation activity on tRNAHis-GUG, tRNALeu-AAG, tRNAAla-AGC, and tRNALys-UUU, but it showed weak activity on tRNAAlaU32C mutant, tRNAVal-CAC, and tRNAGln-CUG ( and Figure S6 B).
Figure 7. Methylation, sequential and structural characterizations of C38-containing non-substrate tRNAs. A. Bar graph of 3H incorporated into non-substrate tRNAs by DNMT2/TRDMT1 at 70 min. B. Sequence alignment of tRNAAla-AGC and four known tRNA substrates of DNMT2/TRDMT1. C. CD spectra of tRNAAla-AGC and tRNAAlaU32G. D. Tertiary structures of tRNAAla-AGC and tRNAAlaU32G predicted. The hydrogen bond interactions between C38 and mutational bases were denoted in yellow dashed lines. E. Sequence alignment of tRNAVal-CAC, tRNAGln-CUG, and four known substrates of DNMT2/TRDMT1. Data were expressed as the mean ± SD from three independent measurements
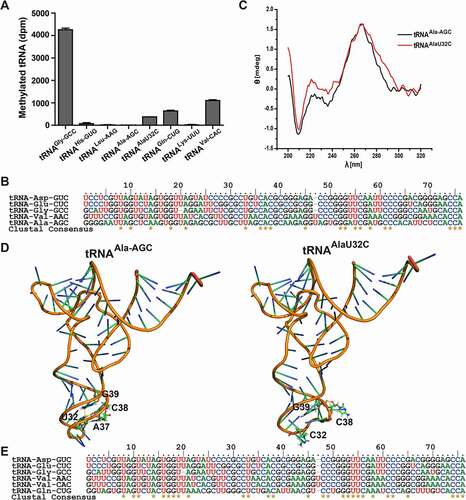
The U32C mutation led to five conserved bases in the anticodon loop of tRNAAla-AGC, consistent with the anticodon loop of the known substrate tRNA (). Moreover, the CD spectrum of tRNAAlaU32C mutant demonstrated two weakened negative peaks at 210 and 240 nm and one strengthened positive peak at 223 nm, indicating that tRNA structural conformation was altered (). In the predicted tRNAAla-AGC tertiary structures, U32C mutation only induced conformational change of anticodon loop (). The tertiary interaction U32-C38 in wild-type tRNAAla-AGC caused C38 to extend into the anticodon loop, whereas the clockwise twist of U32C mutant made C38 stretch out of the anticodon loop. For the above reasons, DNMT2/TRDMT1 showed weak methylation activity on tRNAAlaU32C, indicating that five conserved bases in the anticodon loop could promote the substrate tRNA C38 recognition by DNMT2/TRDMT1. Later, the sequence alignment result of tRNAVal-CAC, tRNAGln-CUG, and four known substrates demonstrated, tRNAVal-CAC had the same conserved bases as that of known substrates, while there was only a different base in tRNAGln-CUG G36 (). However, there were significant differences in primary sequences and secondary structures between tRNALys-UUU and four known substrates. Consistently, DNMT2/TRDMT1 exhibited a weak activity on tRNAVal-CAC or tRNAGln-CUG, whereas it was inactivated on tRNALys-UUU. These indicated that the conserved bases in the anticodon loop played a primary role in the substrate recognition by DNMT2/TRDMT1, and other conserved bases played an auxiliary role. As mentioned above, the conserved bases in known substrate tRNAs contributed to the enzymatic recognition, and tRNAVal-CAC and tRNAGln-CUG were discovered as new substrates for DNMT2/TRDMT1 in vitro.
Discussion
So far, the DNMT2/TRDMT1 activity on DNA has remained controversial. Given the ability of this enzyme to modify some specific C38-containing tRNAs, it is urgent to reveal the recognition and catalysis mechanism of DNMT2/TRDMT1. Since GST-DNMT2/TRDMT1 showed weaker methylation activity on tRNAGly-GCC than His-DNMT2/TRDMT1, we speculated that GST tag with large molecular weight (26 kDa) might cause either active conformation changes in DNMT2/TRDMT1 or steric hindrance effect to block tRNA binding to DNMT2/TRDMT1. Notably, conformation changes of important motifs, such as motif VI (ENV) and motif IV (PCQ) in DNMT2/TRDMT1 structure, can affect the enzymatic activity [Citation19], while some elements in the tRNA cloverleaf structure determine the accuracy of tRNA discrimination by DNMT2/TRDMT1. E. histolytica DNA methyltransferase (Ehmeth), as a member of DNMT2/TRDMT1 family, can bind to the anticodon stem-loop (ACSL) construct of tRNAAsp-GUC, but tRNA full-length sequence is indispensable to the formation of a stable tRNA-Ehmeth complex in vitro [Citation35]. In addition, an earlier study has demonstrated that the tRNAAsp-GUC ACSL construct alone does not serve as DNMT2/TRDMT1 substrate in vitro [Citation12]. Coincidentally, the independent ACSL construct of tRNAGln-UUG is not modified by tRNA Am37 methyltransferase (RlmN) [Citation36]. RlmN-tRNAGlu-UUC crystal structure analysis has indicated that RlmN can interact with D-arm and 3ʹ acceptor end to discriminate the overall structure of tRNA [Citation37]. Similarly, the intact tRNA structure is also necessary for the activities of tRNA Um32/Cm32 methyltransferase TrmJ (EcTrmJ) in E. coli and tRNA m1G37 methyltransferase 5 (Trm5) in eukaryotes and archaea [Citation38,Citation39]. However, E. coli tRNA C/U34 2´-O-methyltransferase (TrmL) can recognize and modify ACSL minihelice extended by 2 base pairs [Citation40]. Besides, only an ACSL and D-stem chimeric structure is necessary for tRNA m1G37methyltransferase (TrmD) activity in bacteria [Citation38]. In this study, T-stem could maintain the L-shaped tertiary structure of tRNAGly-GCC, and it was essential for DNMT2/TRDMT1 catalysis.
The key elements in the tRNA primary sequence and tertiary structure can modulate the recognition and catalysis of RNA methyltransferase for substrate tRNAs [Citation41]. Base pair G19-C56 is precisely discriminated by Trm5 structural domain 1, enabling catalytic domain 2 and domain 3 to execute m1G37 modification [Citation30]. Base pairs G19-C56 and G18-U55 can stabilize D- and T-loop interactions at the elbow region of tRNA [Citation16]. T54-A58 stabilizes the sharp bend of T-loop, while U8-A14 also steadies sharp bend to modulate the acceptor stem direction [Citation32]. G15-C48 maintains the joining of D-stem and T-stem via stacking and hydrogen bonding forces [Citation32]. In this study, the aforementioned tertiary interactions except G18-U55 in tRNAGly-GCC were predicted, of which G19-C56 and T54-A58 interactions were crucial for DNMT2/TRDMT1 activities, indicating that the mutual contact between D- and T-loops at the outer corner of tRNA contributed to this enzyme recognition. Also, conserved U20, A21, U55, and the paired G53-C61 in D- and T-loops were vital components for the functional activity of DNMT2/TRDMT1.
In addition to the anticodon itself, the anticodon loop possesses the restricted sequence variation, albeit as a gathering region of tRNA modification [Citation6]. N33 in the elongator tRNA is nearly always occupied by uridine, and a purine (A or G) is predominantly located at position 37 [Citation42]. Moreover, N32–N38 pair is most likely to be C-A, U-A, U-C, or U-U [Citation43,Citation44]. The conserved anticodon loop sequence impacts the efficiency of tRNA modification to a great extent. For example, G36G37 is a substrate determinant of TrmD, and A37 modification (ms2i6 A37) is a prerequisite for 2´-O-methylation at tRNALeu-C/UAA C/U34 by TrmL [Citation45,Citation46]. In the tRNAGln-UUG recognition by RlmN, U35A/G and G36C mutants exhibit minimal effects on RlmN activities, but U38A and A37G mutants abolish enzymatic activities, and U33G mutant causes a twofold reduction [Citation36]. Conformably, U33 and U35 only indirectly contact the RlmN surface. G or C base can be accommodated at position 36 in the RlmN-tRNA crystal structure; however, U38 directly interacts with several active residues [Citation36]. In the present study, anticodon loop mutations resulted in intra-loop base interactions thus inducing C38 or G38 to twist into the loop, eventually attenuating DNMT2/TRDMT1 activities. Therefore, U33, C36, A37, and the unpaired C32-C38 (CUXXCAC sequence) played critical roles in discriminating and modifying tRNA substrates by DNMT2/TRDMT1.
DNMT2/TRDMT1 specifically distinguishes tRNA C38 through determinative elements in the tRNA structure, and exhibits different preferences for tRNA substrates in various species. For instance, S. pombe Pmt1 has less methylation efficiency for tRNAGlu-UUC than for tRNAAsp-GUC in vitro and in vivo [Citation47]. D. discoideum DnmA can modify tRNAAsp-GUC in vitro and in vivo, but tRNAGlu-UUC and tRNAGly-GCC are only weaker substrates in vitro [Citation48]. Conversely, Geobacter sulfurreducens DNMT2/TRDMT1 homolog shows a good activity for tRNAGlu-UUC in vitro and in vivo, whereas tRNAAsp-GUC C38 methylation is abolished in vivo and relatively weak in vitro [Citation8]. In this study, we proposed that tRNAGly-GCC was more prone to methylation than other substrates, and that tRNAVal-CAC and tRNAGln-CUG could be modified by human DNMT2/TRDMT1 in vitro. These inconsistencies can be attributed to selection differences of detected isoacceptor tRNAs, post-transcriptional modifications of native tRNA, and regulation complexities of methylation modification in vivo. Post-transcriptional modifications endow native tRNA with more orderly base-stacking features [Citation6]. These modifications are interconnected with each other, and drive subsequent modifications to stabilize tRNA active conformation or serve as a recognition component [Citation6]. Mass spectrometry and computational docking analysis of Pmt1-tRNAAsp complex indicates that native tRNAAsp-GUC Q34 is situated in the active site of DNMT2/TRDMT1, contributing to the catalytic activity of this enzyme [Citation49]. Besides, C38 modification by DNMT2/TRDMT1 homologs can also be dynamically modulated in response to nutrient availability [Citation50], oxidative stress [Citation51] and heat shock [Citation2]. However, influence factors of C38 methylation in different tRNA substrates still need to be further explored in detail.
As a molecular genetic medicine, aptamer is a single-stranded DNA or RNA oligonucleotide fragment (20 to 80 nucleotides) that can bind to desired targets with high affinity and specificity [Citation52,Citation53]. Single-stranded oligonucleotides, especially RNA, are easily degraded by serum nucleases; thus, they have a short plasma half-life. Notably, proper chemical modifications, including base and phosphodiester backbone modifications of RNA, can prolong the plasma half-life [Citation54,Citation55]. C38 methylation in tRNA can prevent the tRNA cleavage under stress via DNMT2/TRDMT1 [Citation2]. We discovered determinative elements in tRNA structure by analysing DNMT2/TRDMT1 activities on tRNAGly point and deletion mutants. It is possible that DNMT2/TRDMT1 modifies appropriate RNA aptamers designed based on these elements and stabilizes them. Moreover, siRNA-mediated DNMT2/TRDMT1 silencing and azacytidine- or decitabine-induced inactivation can inhibit the metabolism and proliferation of different cancer cells [Citation56,Citation57]. Overall, this study provides an innovative strategy for designing RNA aptamer to develop anti-tumour drugs via suppressing the DNMT2/TRDMT1 activity.
Conclusions
Taken together, the L-shaped conformation of classical tRNA could be favourable to DNMT2/TRDMT1 activity. The complete sequence and structure of tRNA were dispensable for DNMT2/TRDMT1 activity, whereas T-arm was indispensable to this activity. Additionally, G19, U20, and A21 in D-loop were identified as the important bases for DNMT2/TRDMT1 activity, and G53, C56, A58, and C61 in T-loop were found as the critical bases. Furthermore, the conserved CUXXCAC sequence in the anticodon loop was confirmed to be the most critical determinant, and it could stabilize C38-flipping to promote C38 methylation. Importantly, following these tRNA properties, new substrates tRNAVal-CAC and tRNAGln-CUG were discovered in vitro. Moreover, a single nucleotide substitution U32C could convert non-substrate tRNAAla-AGC into a substrate for DNMT2/TRDMT1. Thus, our discoveries will shed light on substrate recognition mechanism by DNMT2/TRDMT1 involving tRNA primary sequence and tertiary structure.
Materials and methods
Cloning, expression, and purification of DNMT2/TRDMT1 and T7 RNA polymerase
The cDNA sequence of DNMT2/TRDMT1 was amplified from pORF9-hDNMT2a plasmid (No. porf-hDNMT2, InvivoGen, USA). This cDNA was cloned into between NheI and XhoI restriction sites of pET28, and between BamHI and XhoI restriction sites of pGEX-4 T-1, respectively. As shown in Table S1, primer sequences were synthesized by Sangon Biotech (Shanghai, China). The expression of recombinant His-DNMT2/TRDMT1 in E. coli BL21DE3 was induced by 1 mM IPTG at 37°C, and that of recombinant GST-DNMT2/TRDMT1 at 20°C. These two recombinant proteins were separately purified by affinity binding to Ni-NTA Agarose (No. 30,210, Qiagen, Germany) and to Glutathione Sepharose 4B (No. 17–0756-01, GE Healthcare, USA). For in vitro transcription, T7 RNA polymerase was also expressed in E. coli BL21DE3 upon induction with 0.5 mM IPTG at 37°C and was purified using the protocol reported by Grodberg and Dunn [Citation58]. Finally, DNMT2/TRDMT1 was stored in the buffer (20 mM Tris-HCl pH 7.5, 100 mM NaCl, 5 mM β-Mercaptoethanol, 50% Glycerol) and T7 RNA polymerase in the buffer (20 mM Naphosphate pH 7.7, 100 mM NaCl, 1 mM EDTA, 1 mM DTT, 50% Glycerol) at −80°C. The protein concentration was measured with Bradford Protein Assay Kit (No. PP0201, Aidlab, China) and validated by SDS-PAGE.
Enzymatic synthesis of DNA template and tRNA transcript
Homo sapiens C38-containing tRNA gene sequences were derived from Genomic tRNA database (http://lowelab.ucsc.edu/GtRNAdb/Hsapi19/) and listed in the Supplementary Material. Ten tRNA isoacceptor genes (tRNA-Gly-GCC-2-3, tRNA-Asp-GTC-1-1, tRNA-Val-AAC-1-1, tRNA-Val-CAC-1-6, tRNA-Glu-CTC-1-7, tRNA-Ala-AGC-10-1, tRNA-Gln-CTG-13-1, tRNA-His-GTG-2-1, tRNA-Leu-AAG-6-1, and tRNA-Lys-TTT-17-1) were selected according to tRNA score and secondary structure stability. The tRNAs were transcribed by T7 RNA polymerase based on DNA templates synthesized using two oligonucleotide primers with a 10-bp overlap in the anticodon loop [Citation59]. Primers for preparing DNA template were chemically synthesized by Sangon Biotech (Shanghai, China) (Table S1). DNA template was synthesized in 200 μL total volume containing 4 μM forward primer, 4 μM reverse primer, 1× sequenase reaction buffer, 2.5 mM DTT, and 0.25 mM dNTPs. The reaction was triggered by 2 μL DNA Polymerase (No. 70775Y, Thermo Scientific, USA), and the reaction system was incubated at 37°C for about 8 h. In vitro transcription was performed in 500 μL total volume containing 1x transcription buffer (40 mM Tris-HCl pH 8.1, 1 mM spermidine, 20 mM DTT, 0.01% TritonX-100, 24 mM MgCl2), 4 mM NTPs (nucleoside triphosphates) mix, 4 mM GMPs, 400 ~ 750 ng template DNAs, and 20 μL 46.06 μM T7 RNA polymerase. The reaction system was then incubated at 37°C for at least 8 h, followed by the digest of 2 μL RNase-free DNase I (No. EN0525, Thermo Scientific, USA) at 37°C for 15 min [Citation60]. Transcriptional reaction system without the addition of T7 RNA polymerase and RNase-free DNase I were used as control. Then, two reactive samples both mixed with urea loading dye were, respectively, separated by 12% denaturing (7 M Urea) polyacrylamide gel in Owl Separation Systems (Thermo Scientific, USA). The band of template DNA or tRNA transcript was visualized by UV of Gel Documentation System (Clinx, China), and the gel with template DNA or tRNA transcript was cut, crushed, and soaked in TE buffer [Citation61]. After ethanol precipitation, the concentrations of template DNA and tRNA transcript were separately determined by NanoDrop 2000 (Thermo Scientific, USA).
tRNA methylation kinetics
Methylation reaction was carried out using 2 μM tRNA transcripts in 1x methylation buffer (100 mM Tris-HCl PH 7.5, 100 mM KCl, 4 mM DTT, 6 mM MgCl2, 0.1 mM EDTA) [Citation62]. To maximize the refolding of the tRNA transcript, the buffer with 2 μM tRNA was heated to 85°C for 3 min and slowly cooled for about 15 min to room temperature. Afterwards, 10 μM [3H-methyl]-AdoMet (13.5 Ci/mmol) (No. NET155001-MC, Pecrkin Elmer, USA) and 2 μM AdoMet (No. SX5231, Bomeibio, China) were added. The reaction was initiated by adding 1 μM DNMT2/TRDMT1 at 37°C. In the above reaction system, the tRNA transcript was replaced with an equal volume of TE buffer as trial control. At the indicated time interval in 70 min, 4 μL aliquots of the reaction mixture were filtered on a filter pad (No. 1003–917, Whatman, UK). Filter pads were washed with 75 mL 5% Trichloroacetic acid for four times, and afterwards with 75 mL 95% ethanol for three times both at an interval of 10 min. Subsequently, filter pads were air-dried, and bound radioactivity was quantified in scintillation solution using a Tri-carb 2810TR Liquid Scintillation Analyser (Perkin Elmer, USA) [Citation63]. Before each trial, only the scintillation solution was detected as background value. Each trial value was corrected with control and background values. The data of at least three independent experiments were expressed as mean ± standard deviation (SD) by GraphPad Prism.v 5.0. In addition, initial reaction rates of 0.2 μM DNMT2/TRDMT1 on 0–12 μM tRNAGly-GCC were determined in 24 min, and then the obtained initial rates and tRNAGly-GCC concentrations were plotted by Michaelis–Menten equation V = Vmax[S]/(Km+[S]). The enzyme constant Kcat was calculated using the equation Kcat = Vmax/C[enzyme].
Measurements of circular dichroism (CD) spectra
CD spectra of wild-type tRNA, point and deletion mutants in the UV region (200–320 nm) were collected by J-1500 spectrometer (Jasco, Japan). All tRNA samples were adjusted to 0.7 OD at 260 nm in 10 mM phosphate buffer (pH 6.8). A total of 300 μL samples were added into 0.1 cm optical path quartz cuvette and detected at a scan rate of 100 nm per minute and a 1.0 nm bandwidth at 25°C [Citation64]. The control containing phosphate buffer was only used to correct the baseline. Each spectrum was averaged from five successive accumulations and smoothed by Origin 7.5 [Citation65].
Prediction of tRNA secondary and tertiary structures
To investigate the effects of point and deletion mutations on tRNA structures, we predicted secondary structures of tRNAGly-GCC mutants using tRNAscan-SE 2.0 under ‘default’ search mode with a universal genetic code and ‘eukaryotic’ or ‘mixed’ sequence source [Citation66,Citation67]. Subsequently, according to the predicted secondary structure and conserved tertiary interactions (G18-U55, G19-C56, G15-C48, and A14-U8) in cytoplasmic tRNAs, the tertiary structure of tRNAGly-GCC mutant was modelled via RNAComposer under default parameters [Citation68,Citation69].
Author contributions
H.L. conceived and supervised the project. H.L., D.Z., J.W., Y. M., C.C., Y.C., M.Q, and H.D. undertook the validation and the investigation. H.L. took charge of the data curation and analysis. H.L. and H.D. wrote and revised the paper. All authors substantially read and approved the final manuscript.
Disclosure of potential conflicts of interest
No potential conflict of interest was reported by the author(s).
Supplemental Material
Download Zip (7.8 MB)Acknowledgments
We thank Dr. Cuiping Liu from the College of Veterinary Medicine, Huazhong Agricultural University for the assay instruction. Great gratitude goes to linguistics Prof. Ping Liu from Huazhong Agricultural University, Wuhan, China, for her work in English editing and language polishing.
Supplementary material
Supplemental data for this article can be accessed here
References
- Kaneko T, Suzuki T, Kapushoc ST, et al. Wobble modification differences and subcellular localization of tRNAs in Leishmania tarentolae: implication for tRNA sorting mechanism. EMBO J. 2003;22:657–667.
- Schaefer M, Pollex T, Hanna K, et al. RNA methylation by Dnmt2 protects transfer RNAs against stress-induced cleavage. Genes Dev. 2010;24:1590–1595.
- Shanmugam R, Fierer J, Kaiser S, et al. Cytosine methylation of tRNA-Asp by DNMT2 has a role in translation of proteins containing poly-Asp sequences. Cell Discov. 2015;1:15010.
- Tuorto F, Herbst F, Alerasool N, et al. The tRNA methyltransferase Dnmt2 is required for accurate polypeptide synthesis during haematopoiesis. EMBO J. 2015;34:2350–2362.
- Oerum S, Degut C, Barraud P, et al. m1A post-transcriptional modification in tRNAs. Biomolecules. 2017;7:20.
- Han L, Phizicky EM. A rationale for tRNA modification circuits in the anticodon loop. RNA. 2018;24:1277–1284.
- Krutyholowa R, Zakrzewski K, Glatt S. Charging the code - tRNA modification complexes. Curr Opin Struct Biol. 2019;55:138–146.
- Shanmugam R, Aklujkar M, Schafer M, et al. The Dnmt2 RNA methyltransferase homolog of Geobacter sulfurreducens specifically methylates tRNA-Glu. Nucleic Acids Res. 2014;42:6487–6496.
- Burgess AL, David R, Searle IR. Conservation of tRNA and rRNA 5-methylcytosine in the kingdom Plantae. BMC Plant Biol. 2015;15:199.
- Muller M, Hartmann M, Schuster I, et al. Dynamic modulation of Dnmt2-dependent tRNA methylation by the micronutrient queuine. Nucleic Acids Res. 2015;43:10952–10962.
- Ehrenhofer-Murray AE. Cross-talk between Dnmt2-dependent tRNA methylation and queuosine modification. Biomolecules. 2017;7:14.
- Kaiser S, Jurkowski TP, Kellner S, et al. The RNA methyltransferase Dnmt2 methylates DNA in the structural context of a tRNA. RNA Biol. 2017;14:1241–1251.
- Cantara WA, Bilbille Y, Kim J, et al. Modifications modulate anticodon loop dynamics and codon recognition of E. coli tRNA(Arg1,2). J Mol Biol. 2012;416:579–597.
- Le Brun E, Arluison V, Wien F. Application of synchrotron radiation circular dichroism for RNA structural analysis. Methods Mol Biol. 2020;2113:135–148.
- Messmer M, Gaudry A, Sissler M, et al. Pathology-related mutation A7526G (A9G) helps in the understanding of the 3D structural core of human mitochondrial tRNA(Asp). RNA. 2009;15:1462–1468.
- Lang BF, Lavrov D, Beck N, et al. Mitochondrial tRNA structure, identity, and evolution of the genetic code. In: Bullerwell CE, editor. Organelle genetics: evolution of organelle genomes and gene expression. Berlin, Heidelberg: Springer Berlin Heidelberg; 2012. p. 431–474.
- Pawlowska R, Janicka M, Jedrzejczyk D, et al. RNA fragments mimicking tRNA analogs interact with cytochrome c. Mol Biol Rep. 2016;43:295–304.
- Carmona P, Rodriguez-Casado A, Molina M. Conformational structure and binding mode of glyceraldehyde-3-phosphate dehydrogenase to tRNA studied by Raman and CD spectroscopy. Biochim Biophys Acta. 1999;1432:222–233.
- Jurkowski TP, Meusburger M, Phalke S, et al. Human DNMT2 methylates tRNA(Asp) molecules using a DNA methyltransferase-like catalytic mechanism. RNA. 2008;14:1663–1670.
- Jurkowski TP, Shanmugam R, Helm M, et al. Mapping the tRNA binding site on the surface of human DNMT2 methyltransferase. Biochemistry. 2012;51:4438–4444.
- Hermann A, Schmitt S, Jeltsch A. The human Dnmt2 has residual DNA-(cytosine-C5) methyltransferase activity. J Biol Chem. 2003;278:31717–31721.
- Motorin Y, Helm M. tRNA stabilization by modified nucleotides. Biochemistry. 2010;49:4934–4944.
- Elhardt W, Shanmugam R, Jurkowski TP, et al. Somatic cancer mutations in the DNMT2 tRNA methyltransferase alter its catalytic properties. Biochimie. 2015;112:66–72.
- Schmeing TM, Voorhees RM, Kelley AC, et al. How mutations in tRNA distant from the anticodon affect the fidelity of decoding. Nat Struct Mol Biol. 2011;18:432–436.
- Wells BD, Yang JT. A computer probe of the circular dichroic bands of nucleic acids in the ultraviolet region. I. Transfer ribonucleic acid. Biochemistry. 1974;13:1311–1316.
- Agris PF, Brown SC. Systems for the NMR study of modified nucleoside-dependent, metal-ion induced conformational changes in nucleic acids. Methods Enzymol. 1995;261:270–299.
- Hori H, Yamazaki N, Matsumoto T, et al. Substrate recognition of tRNA (Guanosine-2ʹ-)-methyltransferase from Thermus thermophilus HB27. J Biol Chem. 1998;273:25721–25727.
- Ochi A, Makabe K, Kuwajima K, et al. Flexible recognition of the tRNA G18 methylation target site by TrmH methyltransferase through first binding and induced fit processes. J Biol Chem. 2010;285:9018–9029.
- Chatterjee K, Blaby IK, Thiaville PC, et al. The archaeal COG1901/DUF358 SPOUT-methyltransferase members, together with pseudouridine synthase Pus10, catalyze the formation of 1-methylpseudouridine at position 54 of tRNA. RNA. 2012;18:421–433.
- Goto-Ito S, Ito T, Yokoyama S. Trm5 and TrmD: two enzymes from distinct origins catalyze the identical tRNA modification, m(1)G37. Biomolecules. 2017;7:32.
- Gu X, Ivanetich KM, Santi DV. Recognition of the T-arm of tRNA by tRNA (m5U54)-methyltransferase is not sequence specific. Biochemistry. 1996;35:11652–11659.
- Rich A, RajBhandary UL. Transfer RNA: molecular structure, sequence, and properties. Annu Rev Biochem. 1976;45:805–860.
- Li R, Ge HW, Cho SS. Sequence-dependent base-stacking stabilities guide tRNA folding energy landscapes. J Phys Chem B. 2013;117:12943–12952.
- Sokolowski M, Klassen R, Bruch A, et al. Cooperativity between different tRNA modifications and their modification pathways. Biochim Biophys Acta Gene Regul Mech. 2018;1861:409–418.
- Schulz EC, Roth HM, Ankri S, et al. Structure analysis of Entamoeba histolytica DNMT2 (EhMeth). PLoS One. 2012;7:e38728.
- Fitzsimmons CM, Fujimori DG. Determinants of tRNA recognition by the radical SAM Enzyme RlmN. PLoS One. 2016;11:e0167298.
- Schwalm EL, Grove TL, Booker SJ, et al. Crystallographic capture of a radical S-adenosylmethionine enzyme in the act of modifying tRNA. Science. 2016;352:309–312.
- Christian T, Hou YM. Distinct determinants of tRNA recognition by the TrmD and Trm5 methyl transferases. J Mol Biol. 2007;373:623–632.
- Somme J, Van Laer B, Roovers M, et al. Characterization of two homologous 2ʹ-O-methyltransferases showing different specificities for their tRNA substrates. RNA. 2014;20:1257–1271.
- Zhou M, Long T, Fang ZP, et al. Identification of determinants for tRNA substrate recognition by Escherichia coli C/U34 2ʹ-O-methyltransferase. RNA Biol. 2015;12:900–911.
- Long T, Li J, Li H, et al. Sequence-specific and shape-selective RNA recognition by the human RNA 5-methylcytosine methyltransferase NSun6. J Biol Chem. 2016;291:24293–24303.
- Auffinger P, Westhof E. Singly and bifurcated hydrogen-bonded base-pairs in tRNA anticodon hairpins and ribozymes. J Mol Biol. 1999;292:467–483.
- Marck C, Grosjean H. tRNomics: analysis of tRNA genes from 50 genomes of Eukarya, Archaea, and Bacteria reveals anticodon-sparing strategies and domain-specific features. RNA. 2002;8:1189–1232.
- Juhling F, Morl M, Hartmann RK, et al. tRNAdb 2009: compilation of tRNA sequences and tRNA genes. Nucleic Acids Res. 2009;37:D159–62.
- Brule H, Elliott M, Redlak M, et al. Isolation and characterization of the human tRNA-(N1G37) methyltransferase (TRM5) and comparison to the Escherichia coli TrmD protein. Biochemistry. 2004;43:9243–9255.
- Benitez-Paez A, Villarroya M, Douthwaite S, et al. YibK is the 2ʹ-O-methyltransferase TrmL that modifies the wobble nucleotide in Escherichia coli tRNA(Leu) isoacceptors. RNA. 2010;16:2131–2143.
- Becker M, Muller S, Nellen W, et al. Pmt1, a Dnmt2 homolog in Schizosaccharomyces pombe, mediates tRNA methylation in response to nutrient signaling. Nucleic Acids Res. 2012;40:11648–11658.
- Muller S, Windhof IM, Maximov V, et al. Target recognition, RNA methylation activity and transcriptional regulation of the Dictyostelium discoideum Dnmt2-homologue (DnmA). Nucleic Acids Res. 2013;41:8615–8627.
- Johannsson S, Neumann P, Wulf A, et al. Structural insights into the stimulation of S. pombe Dnmt2 catalytic efficiency by the tRNA nucleoside queuosine. Sci Rep. 2018;8:8880.
- Tovy A, Siman Tov R, Gaentzsch R, et al. A new nuclear function of the Entamoeba histolytica glycolytic enzyme enolase: the metabolic regulation of cytosine-5 methyltransferase 2 (Dnmt2) activity. PLoS Pathog. 2010;6:e1000775.
- Thiagarajan D, Dev RR, Khosla S. The DNA methyltransferase Dnmt2 participates in RNA processing during cellular stress. Epigenetics. 2011;6:103–113.
- Yazdian-Robati R, Arab A, Ramezani M, et al. Application of aptamers in treatment and diagnosis of leukemia. Int J Pharm. 2017;529:44–54.
- Zhang Y, Lai BS, Juhas M. Recent advances in aptamer discovery and applications. Molecules. 2019;24:941.
- Lipi F, Chen S, Chakravarthy M, et al. In vitro evolution of chemically-modified nucleic acid aptamers: pros and cons, and comprehensive selection strategies. RNA Biol. 2016;13:1232–1245.
- Urak KT, Shore S, Rockey WM, et al. In vitro RNA SELEX for the generation of chemically-optimized therapeutic RNA drugs. Methods. 2016;103:167–174.
- Schaefer M, Hagemann S, Hanna K, et al. Azacytidine inhibits RNA methylation at DNMT2 target sites in human cancer cell lines. Cancer Res. 2009;69:8127–8132.
- Lewinska A, Adamczyk-Grochala J, Kwasniewicz E, et al. Reduced levels of methyltransferase DNMT2 sensitize human fibroblasts to oxidative stress and DNA damage that is accompanied by changes in proliferation-related miRNA expression. Redox Biol. 2018;14:20–34.
- Grodberg J, Dunn JJ. ompT encodes the Escherichia coli outer membrane protease that cleaves T7 RNA polymerase during purification. J Bacteriol. 1988;170:1245–1253.
- Sherlin LD, Bullock TL, Nissan TA, et al. Chemical and enzymatic synthesis of tRNAs for high-throughput crystallization. RNA. 2001;7:1671–1678.
- Kao C, Zheng M, Rudisser S. A simple and efficient method to reduce nontemplated nucleotide addition at the 3 terminus of RNAs transcribed by T7 RNA polymerase. RNA. 1999;5:1268–1272.
- Liu C, Gamper H, Shtivelband S, et al. Kinetic quality control of anticodon recognition by a eukaryotic aminoacyl-tRNA synthetase. J Mol Biol. 2007;367:1063–1078.
- Christian T, Lahoud G, Liu C, et al. Control of catalytic cycle by a pair of analogous tRNA modification enzymes. J Mol Biol. 2010;400:204–217.
- Christian T, Lahoud G, Liu C, et al. Mechanism of N-methylation by the tRNA m1G37 methyltransferase Trm5. RNA. 2010;16:2484–2492.
- Vendeix FA, Murphy F, Cantara WA, et al. Human tRNA(Lys3)(UUU) is pre-structured by natural modifications for cognate and wobble codon binding through keto-enol tautomerism. J Mol Biol. 2012;416:467–485.
- Basu A, Jaisankar P, Suresh Kumar G. Binding of the 9-O-N-aryl/arylalkyl amino carbonyl methyl substituted berberine analogs to tRNA(phe.). PLoS One. 2013;8:e58279.
- Lowe TM, Chan PP. tRNAscan-SE On-line: integrating search and context for analysis of transfer RNA genes. Nucleic Acids Res. 2016;44:W54–7.
- Chan PP, Lowe TM. tRNAscan-SE: searching for tRNA genes in genomic sequences. Methods Mol Biol. 2019;1962:1–14.
- Popenda M, Szachniuk M, Antczak M, et al. Automated 3D structure composition for large RNAs. Nucleic Acids Res. 2012;40:e112.
- Antczak M, Popenda M, Zok T, et al. New functionality of RNAComposer: an application to shape the axis of miR160 precursor structure. Acta Biochim Pol. 2016;63:737–744.