ABSTRACT
Xrn1-resistant RNA structures are multifunctional elements employed by an increasing number of RNA viruses. One of such elements is the coremin motif, discovered in plant virus RNAs, of which the structure has been hypothesized to form a yet unelucidated pseudoknot. Recently, the coremin motif was shown to be capable of stalling not only Xrn1, but scanning ribosomes as well. Following that observation, in this study we demonstrate that the coremin motif can promote −1 ribosomal frameshifting, similar to better-characterized viral frameshifting pseudoknots. Since this function was lost in concert with substitutions that were known to disturb Xrn1-resistance, we developed a frameshifting screen for finding novel Xrn1-resistant RNAs by randomizing parts of the coremin motif. This yielded new insights into the coremin motif structure, as Xrn1-resistant variations were identified that more clearly indicate a pseudoknot interaction. In addition, we show that the Xrn1-resistant RNA of Zika virus promotes frameshifting as well, while known −1 programmed ribosomal frameshifting pseudoknots do not stall Xrn1, suggesting that promoting frameshifting is a universal characteristic of Xrn1-resistant RNAs, but that Xrn1-resistance requires more than just a frameshifting pseudoknot.
Introduction
RNA viruses employ a large variety of structural motifs within their genome, of which pseudoknots are ubiquitous and involved in an extensive set of functions [Citation1,Citation2]. Pseudoknots are formed when part of the single-stranded loop sequence of a secondary structural eleent base-pairs with a – usually complementary – set of nucleotides on the same RNA molecule [Citation3]. The structure that follows can be highly thermally stable and its topology will strongly depend on the sizes and identities of its constituent stems and loops [Citation4,Citation5]. Which functions a pseudoknot may confer depend on its topology, stability, and perhaps most notably, its position within the viral genome. In positive-strand RNA viruses, structures involving pseudoknots are often present within the untranslated regions (UTRs), where examples of regulatory roles are modulation of translation initiation [Citation6–8] and genome replication [Citation9,Citation10]. In addition, pseudoknots located in coding sequences may stimulate programmed −1 ribosomal frameshifting (−1 PRF) when combined with a proximal, upstream slippery sequence [Citation5,Citation11,Citation12]. A translating ribosome that encounters and is stalled before such a structure frequently slips back a single nucleotide, allowing for regulation of relative expression levels of frameshifted versus non-frameshifted products.
A relatively novel class of RNA structures called Xrn1-resistant RNAs (xrRNA) is generally located in the 3’ UTRs of several plant viruses and the Flaviviridae family [Citation13–19] but recent studies have indicated their presence in 5’ UTRs, coding regions and intergenic regions as well [Citation20,Citation21]. Here, they stall 5’ to 3’ degradation by the conserved exoribonuclease Xrn1, which causes accumulation of subgenomic RNAs. In turn, this accumulation is likely involved in promoting several aspects of the viral infection [Citation22–25], which either follows from sequestering of Xrn1, but may depend on the nature of the protected genomic RNA as well. Crystallographic and functional analyses have indicated that in order to stall Xrn1, pseudoknot interactions are required within the classes of xrRNA found in Flaviviridae [Citation26,Citation27], and Tombusviridae and Solemoviridae [Citation28,Citation29]. The crystal structure of the flaviviral Zika virus xrRNA (xrRNAZIKV) shows a ring-like fold that forms a highly mechanically stable, topological blockade for the Xrn1 enzyme as it approaches the structure [Citation30].
A shorter, seemingly less elaborate, type of xrRNA called the coremin motif (xrRNAC) was initially discovered in Beet necrotic yellow vein virus (BNYVV) as a short hairpin (hp1) with a four-nucleotide loop (lp1), followed by a conserved spacer of roughly ten nucleotides [Citation17]. Recently, the supposed conservation of lp1 size was disputed by showing how xrRNAC with lp1s of six or more nts were Xrn1-resistant (XR) as well, and these variations were present in a large variety of viruses (Dilweg et al. submitted for publication). No crystal structure has been elucidated for an xrRNAC yet, but analysis of the structural requirements of this motif revealed that the spacer has to be followed by another hairpin (hp2) of which the structure is conserved, but not the sequence () [Citation31]. This downstream hairpin, not a feature that commonly follows known pseudoknots, likely plays a part in what distinguishes this XR motif from a more regular pseudoknot. Due to the lack of possible anti-parallel Watson-Crick base pairs (bps) between lp1 and the spacer, a pseudoknot was not initially apparent in xrRNAC. However, further mutational analysis suggested that a (non-canonical) pseudoknot, or similar tertiary interaction, is necessary between the conserved 5’-CUAC-3’ spacer sequence and lp1, of which the latter requires a 5’-YGAD-3’ consensus (or 5’-YGNNAD-3’ in the case of a 6-nt lp1 [Dilweg et al. submitted for publication]) in order to retain Xrn1 stalling capacity. The overall structure and stem-sizes that would result from a pseudoknot interaction in the lp1 and spacer portion of xrRNAC resembles an H-type pseudoknot that in, for instance, Beet western yellows virus (BWYV) [Citation32] and Potato leaf roll virus (PLRV) [Citation33] confers −1 PRF stimulation (). In these luteoviral frameshifting pseudoknots, additional tertiary interactions are formed between the bridging loop elements L1 and L2, with adjacent stem elements S2 and S1, respectively, in order to achieve the resistance against elongating ribosomes that is necessary for promoting −1 PRF [Citation34]. It is however unlikely that simply a stable structure is sufficient for resisting Xrn1, as it is known to degrade structures of high thermal stability [Citation35–37].
Figure 1. (A) Depiction of the secondary structure of xrRNABNYVV-fs, as determined in our previous study [Citation31], with some changes as described in the main text. Nucleotides are numbered from the first residue in the hp1 stem, and coloured according to the hypothesized position in the pseudoknot interaction, as depicted in (B). (B) Pseudoknot depictions of xrRNABNYVV-fs, the BWYV−1 PRF pseudoknot (BWYV-PK) (adapted from Su et al. [Citation32]), the SRV-1 -1 PRF pseudoknot (SRV-1-PK) [Citation38], and xrRNAZIKV (adapted from Dilweg et al. [Citation18]). Red arrows depict the nucleotide substitutions that cause loss of XR, which were used in the−1 PRF assay. (C) Autoradiogram from−1 PRF assay for determination of frameshifting efficiencies for xrRNABNYVV-fs and xrRNAZIKV (wt), and corresponding mutant constructs (mut), with SRV-1-PK as a positive control. Image was obtained from exposure of phosphoimager screen following SDS-PAGE analysis of RRL samples containing 35S-methionine- and cysteine-labelled translation products. Frameshifting efficiencies are calculated from the ratio of − 1 PRF (FS) and non-frameshifted (NFS) products, as explained in ‘Materials and methods’, and given here as the mean (± SD) percentage from three independent experiments. Constructs of xrRNABNYVV-fs tested here correspond with those given in , and constructs of xrRNAZIKV correspond with the structure found in ZIKV isolate 15,555 (accession number MN025403) tested for XR in Dilweg et al. [Citation18].
![Figure 1. (A) Depiction of the secondary structure of xrRNABNYVV-fs, as determined in our previous study [Citation31], with some changes as described in the main text. Nucleotides are numbered from the first residue in the hp1 stem, and coloured according to the hypothesized position in the pseudoknot interaction, as depicted in (B). (B) Pseudoknot depictions of xrRNABNYVV-fs, the BWYV−1 PRF pseudoknot (BWYV-PK) (adapted from Su et al. [Citation32]), the SRV-1 -1 PRF pseudoknot (SRV-1-PK) [Citation38], and xrRNAZIKV (adapted from Dilweg et al. [Citation18]). Red arrows depict the nucleotide substitutions that cause loss of XR, which were used in the−1 PRF assay. (C) Autoradiogram from−1 PRF assay for determination of frameshifting efficiencies for xrRNABNYVV-fs and xrRNAZIKV (wt), and corresponding mutant constructs (mut), with SRV-1-PK as a positive control. Image was obtained from exposure of phosphoimager screen following SDS-PAGE analysis of RRL samples containing 35S-methionine- and cysteine-labelled translation products. Frameshifting efficiencies are calculated from the ratio of − 1 PRF (FS) and non-frameshifted (NFS) products, as explained in ‘Materials and methods’, and given here as the mean (± SD) percentage from three independent experiments. Constructs of xrRNABNYVV-fs tested here correspond with those given in Figure 4, and constructs of xrRNAZIKV correspond with the structure found in ZIKV isolate 15,555 (accession number MN025403) tested for XR in Dilweg et al. [Citation18].](/cms/asset/8751e8e5-2ee5-417a-8fa6-3d0a2746c280/krnb_a_2205224_f0001_oc.jpg)
Guided by the hypothesis that xrRNAC forms a non-canonical pseudoknot that resembles frameshifting pseudoknots, and the recent discovery that xrRNAC is able to block scanning ribosomes (Dilweg et al. submitted for publication), this study aimed to determine whether xrRNAC could stimulate frameshifting as well. Efficient −1 PRF is indeed demonstrated by xrRNAC here, which inspired us to setup a frameshifting-based XR screen utilizing this function for discovery of new XR sequences within the coremin motif context. As such, a novel type of xrRNA was identified, one which, in contrast to xrRNAC, does appear to form a more canonical pseudoknot with Watson-Crick base pairing. This interaction was further investigated by digesting a variety of mutant constructs derived from the XR hits, leading to the conclusion that a pseudoknot interaction is indeed likely, although additional tertiary interactions are required as well. The discovery of this Watson-Crick-based coremin motif (xrRNAWCC) led to the identification of such putative xrRNAs within two viral species. These results shed new light on the variety of potential functions of xrRNAs and what exactly is needed for a structure to stall Xrn1, while providing further indication of the widespread distribution of xrRNA motifs.
Materials and methods
Plasmid for frameshifting −1 PRF assay
The frameshifting reporter plasmid pSF208 [Citation38] was used, which is a version of the pSF2 frameshift reporter plasmid that carries a UUUAAAC slippery sequence instead of the original GGGAAAC [Citation39]. Digestion of pSF208 with SpeI and NcoI allowed insertion of various xrRNA constructs. Due to usage of constructs with varying lengths, maintenance of reading frame was ensured by addition of one U or two A’s downstream of the xrRNA sequences (). Inserts were produced from complementary oligonucleotides that were purchased from Sigma Aldrich in desalted form and designed to leave compatible single-stranded overhangs. Complementary oligonucleotides were annealed and subsequently inserted into the digested plasmid, followed by transformation in E. coli XL10, selection for ampicillin resistance and verification through Sanger sequencing (LGTC, Leiden). A list of oligonucleotides is available upon request.
In vitro transcription for −1 PRF assay
About 1.5 μg of cloned plasmids were linearized by digestion with BamHI and subsequently purified with the PureYield™ Plasmid Miniprep System (Promega). SP6 polymerase transcription reactions were carried out in a 5 μL volume containing 2 μL of eluted DNA, and 10 U SP6 RNA polymerase, 40 mM Tris-HCl (pH 7.9), 6 mM MgCl2, 1 mM DTT, 2 mM spermidine and 0.5 mM of each ATP, CTP, GTP and UTP. Mixtures were incubated for 2 h at 37°C, after which samples were taken of transcripts for checking the quality and quantity on an agarose gel. After adjusting for variation in yield where necessary, mixtures were diluted five-fold with Milli-Q water in order to reach a concentration of approximately 50 ng/uL.
In vitro translation for −1 PRF assay
Reaction mixtures consisted of ~ 50 ng RNA, 0.5–1 μL of 35S-labelled amino acid mixture (Easytag Express 35S protein labelling mix, PerkinElmer, Netherlands), 2.5 μL nuclease-treated rabbit reticulocyte lysate (RRL, Promega) and Milli-Q water up to a total volume of 5 μL. Samples were incubated for 1 h @ 28°C, after which 5 μL of 2× Laemmli buffer was added, and the samples were heated to 65°C for 5 mins to allow denaturation of protein products. Half volume of resulting samples was loaded on 12.5% SDS polyacrylamide gels, which ran on 20 mA in SDS-containing electrophoresis buffer. Gels were dried and used to expose phosphoimager screens for at least 48 h. Screens were scanned with a Typhoon™ BioMolecular Imager (GE Healthcare), and the resulting image was used for analysis using Quantity One software. Frameshift percentages were calculated by quantification of the ratio of −1 PRF to non-frameshifted products, while adjusting for the number of incorporated methionines and cysteines (10 in the non-frameshifted product, 28–30 in the −1 PRF product, depending on the insert). All constructs were tested at least in triplicate.
Design of plasmid for frameshifting-based XR screen
In the T7 RNA polymerase-inducible expression plasmid pET52b(+) was placed a synthetic DNA fragment containing genes for the SmallBiT and LargeBiT domains that form a functional Nano Luciferase when expressed and reconstituted, inspired by the NanoBiT complementation reporter (Supplementary Table S1) [Citation40]. This fragment was inserted in the multiple cloning site using digestion with XbaI and SacI. Initially meant for studying peptide-peptide interactions, the resulting plasmid pET52b-PR-KE was repurposed for use in a frameshifting-based XR screen. For this, pET52b-PR-KE was digested with Acc65I and EcoRV, which removed the SmallBiT stop codon and downstream spacer, and the following oligonucleotide (annealed to a complementary oligonucleotide with compatible single-stranded overhangs) was inserted: 5’-GTACCTTTTTAAACTAGTGAGCGGCCAGCTCCAGGCCGCCAAACAATATGGAGCTGTAAGCTT-3’ (SpeI and HindIII sites are underlined, the slippery sequence is italicized, the in-frame stop codon is shown in bold) to make pET52b-cor11. Due to insufficient efficiency gained with transformation in E. coli BL21, it was decided to try expression in the XL10 strain instead. Since XL10 does not express T7 RNA polymerase, the frameshifting cassette of pET52b-cor11 was amplified with pETfor and pETrev3 primers (Supplementary Table S1), in order to clone it into pUC19 under control of a lac promoter. Since insertion of randomized nucleotides required digestion with HindIII, the innate HindIII-site of pUC19 was inactivated by digestion with HindIII and filling in the 5’ overhangs by Klenow fragment in the presence of dNTPs, followed by blunt-end ligation. The amplified frameshifting cassette and pUC19ΔHindIII were digested with AvaI, purified by ethanol precipitation and subsequently incubated with XbaI. Following isolation of digested fragments from an agarose gel, they were ligated, transformed and clones were verified using Sanger sequencing (LGTC, Leiden), leading to isolation of the plasmid termed pMOFS.
Frameshifting-based XR screen
Inserts carrying an xrRNAC randomized at positions 5–8 and 18–21 () were designed and annealed to complementary oligonucleotides (randomized at corresponding positions) (Supplementary Table S1), such that overhangs compatible with SpeI- and HindIII-digested pMOFS are created for ligation. This mixture of randomized plasmids was transformed in E. coli XL10 on LB-plates containing ampicillin and 1 mM IPTG. A 500-fold dilution in Milli-Q of NanoGlo® Luciferase Assay Substrate (Furimazine, Promega) was prepared. Using a nebulizer, a thin layer of this solution was sprayed on each plate and incubated for 3 minutes at 20°C. After incubation, chemiluminescence was measured for 300 seconds of exposure on a Bio-Rad EZ Gel Documentation System, followed by taking a colorimetric picture in exactly the same position. These two images were compared and light-positive colonies were selected, streaked on a back-up plate and analysed through direct colony sequencing.
Figure 2. Frameshifting-based XR screen leads to the discovery of a new variation of xrRNAC. (A) Schematic of pMOFS (top) that carries the split NLuc domains separated by the partially randomized frameshift cassette that is inserted during the screen (bottom). Two potential stop codons are indicated with their frame given in parentheses. Secondary structure of the xrRNAC is given as two hairpins (hp1, hp2) with numbers indicating the positions of nucleotides involved in the motif. Nucleotides that are randomized (orange in lp1, blue in spacer) are given as ‘N’. Denaturing polyacrylamide gels depict results of in vitro Xrn1 degradation assay on Hit1 and Hit2 (B) that followed as hits from the frameshifting-based XR screen, and on an SPlV5 sequence (C) that followed from subsequent GenBank searches (accession number K×883721). Boxes above the gels depict the sequences that were determined in these XR-positive hits for lp1 and spacer. Note that Hit1 differs in length due to using a different type of DNA template which omits the 5’-UGUC-3’ sequence from positions 36–39. This downstream sequence was determined to not be necessary for XR earlier [Citation31], but was added for testing XR of other frameshifting hits here in order to match the downstream sequence as present in the frameshift cassette.
![Figure 2. Frameshifting-based XR screen leads to the discovery of a new variation of xrRNAC. (A) Schematic of pMOFS (top) that carries the split NLuc domains separated by the partially randomized frameshift cassette that is inserted during the screen (bottom). Two potential stop codons are indicated with their frame given in parentheses. Secondary structure of the xrRNAC is given as two hairpins (hp1, hp2) with numbers indicating the positions of nucleotides involved in the motif. Nucleotides that are randomized (orange in lp1, blue in spacer) are given as ‘N’. Denaturing polyacrylamide gels depict results of in vitro Xrn1 degradation assay on Hit1 and Hit2 (B) that followed as hits from the frameshifting-based XR screen, and on an SPlV5 sequence (C) that followed from subsequent GenBank searches (accession number K×883721). Boxes above the gels depict the sequences that were determined in these XR-positive hits for lp1 and spacer. Note that Hit1 differs in length due to using a different type of DNA template which omits the 5’-UGUC-3’ sequence from positions 36–39. This downstream sequence was determined to not be necessary for XR earlier [Citation31], but was added for testing XR of other frameshifting hits here in order to match the downstream sequence as present in the frameshift cassette.](/cms/asset/62304178-f53b-4ddc-95fb-4bf0bb3d7dde/krnb_a_2205224_f0002_oc.jpg)
In vitro Xrn1 degradation assay
Oligonucleotides functioning as templates for transcription were designed to be the reverse complement of a T7 promoter, a 12-nt leader and the construct of interest [Citation41]. As such, another oligo bearing the T7 promoter and part of the leader (5’-GTAATACGACTCACTATAGATTA-3’) could be annealed to a portion of the prior oligo’s, which served as the DNA template for transcription by T7 RNA polymerase as described in Dilweg et al. [Citation31]. Xrn1 digestion was performed and analysed using 14% denaturing gels as described in Dilweg et al. [Citation18]. All constructs were tested at least twice. A list of oligonucleotides is available upon request.
Results
XrRNAs stimulate −1 ribosomal frameshifting
In order to investigate whether the coremin motif stimulates frameshifting, constructs of interest were inserted in a reporter plasmid that has previously been used to research frameshifting efficiencies of other pseudoknot and stem-loop structures [Citation11,Citation38]. As such, they were placed downstream of a slippery sequence (UUUAAAC) known to efficiently promote −1 PRF [Citation39]. An in-frame stop codon located just between the slippery sequence and the inserted sequence ensures that regular translation is terminated to produce a ~ 19kDa product, but continues in the case of a ribosomal slippage to produce a ~ 57kDa product. Included in this −1 PRF assay were two types of xrRNA, the xrRNAC from BNYVV RNA3 (xrRNABNYVV) and the xrRNA found in the flaviviral xrRNAZIKV, and mutant versions that have previously been determined to disturb Xrn1-stalling ability () [Citation18,Citation27,Citation31]. The xrRNABNYVV construct was adjusted slightly with a more stable version of its hp1 stem, and a U14A substitution in the spacer and lp2 substitution for a GAAA tetraloop, both meant to prevent the presence of a premature stop codon (xrRNABNYVV-fs). However, these changes are known not to influence XR [Citation31]. As a control, the pseudoknot conferring the simian retrovirus-1 (SRV-1) gag-pro frameshifting signal (SRV-1-PK) was included as well () [Citation38]. The −1 PRF assay, performed in Rabbit reticulocyte lysate, yielded frameshifting efficiencies of 22.4% and 8.9% for xrRNABNYVV-fs and xrRNAZIKV, respectively (). Disturbing the putative pseudoknot of xrRNABNYVV-fs by changing lp1 from CGAA to AGAA reduced this efficiency roughly ten-fold. For xrRNAZIKV, substituting the base triple from a U•A-U to a C•A-U reduced frameshifting roughly three-fold. This puts frameshifting stimulation by xrRNABNYVV-fs on par with SRV-1-PK, although it should be noted that contrary to the xrRNA constructs tested here, the SRV-1-PK control employed the wild-type, and less efficient GGGAAAC slippery sequence.
Establishment of a frameshifting-based XR screen
The frameshifting stimulation by xrRNABNYVV-fs, and the loss of this function expectedly coinciding with the loss of XR, inspired utilizing this ability in a screen aimed at identifying novel XR, coremin-like sequences. For this, a split NanoLuc luciferase (NLuc) reporter system [Citation40] was adapted (see Materials & methods), such that the upstream SmallBiT ORF is linked by a frameshift cassette to the downstream LargeBiT ORF located in the −1 reading frame (). In this way, expression of the full-length NLuc only continues in the case of a −1 PRF event (). This allowed insertion of xrRNAC motifs with nucleotides randomized in lp1 and positions 18–21 of the spacer in order to screen for frameshifting-positive sequences. Plasmids carrying these partially randomized inserts were expressed in E. coli, and NLuc production was screened by applying a furimazine solution onto the transformed colonies. Manual selection of light-positive colonies over multiple individual experiments yielded 46 putative hits, of which 28 had a well-distinguishable sequence, e.g. no heterogenous signal or plasmid without insert (Supplementary Table S2).
A subset of 17 frameshifting hits was selected for testing XR, based either on subjective judgement of highest light expression – thus frameshifting efficiency, or on the number of potential Watson-Crick or wobble pair interactions between the randomized sequences (Supplementary Table S2). Transcripts for an in vitro Xrn1 degradation assay carrying a 10-nt long leader were produced from DNA oligonucleotides and incubated with RppH and Xrn1. In this assay, 15 frameshifting hits did not show XR (Supplementary Figure S1), while two XR hits became apparent: the lp1-spacer combinations of GGGG – CCUA (Hit1) and GGCG – CGCU (Hit2, ) suggesting the potential of a (regular, anti-parallel) pseudoknot interaction (stem 2 [S2]) with at least three Watson-Crick or wobble pairs. Such an interaction would deviate from the coremin motif of xrRNABNYVV, in which a canonical, anti-parallel pseudoknot utilizing Watson-Crick base pairings does not seem likely [Citation31]. A BLAST search on GenBank using the conserved hp1 and spacer sequences of these two hits yielded one aligned sequence starting 8 nt downstream of the 3’ UTR of Sanxia picorna-like virus 5 (SPlV5). This contained exactly the hp1 stem and loop region found in xrRNABNYVV, but with an lp1-spacer interaction of GGAG – CUCA that could theoretically form a three-bp pseudoknot as well. Between Hit2 and SPlV5 ×rRNA, nucleotides 7 and 19 are structurally covaried, which could indicate that this interaction indeed occurs and is functionally important. Furthermore, one nucleotide further downstream, the SPlV5 sequence is followed by a 4-bp hp2, following the parameters needed for an xrRNA. Digesting a transcript carrying the SPlV5 motif, in its own context, indeed shows that this sequence is resistant to Xrn1 and thus, a candidate xrRNA (). Overall, these findings suggest a novel Watson-Crick-type coremin motif (xrRNAWCC) that utilizes an antiparallel pseudoknot interaction that forms an S2 of nt 6 with 20, 7 with 19, and 8 with 18 (see ).
Figure 3. In vitro Xrn1 digestion assays analysing the predicted S2 element for xrRNAWCC. (A) Secondary structure of the SPlV5 xrRNA candidate sequence as present in KX883721 (top), based on the secondary structure prediction of xrRNABNYVV from KX665538 (bottom). (B) Models depicting the prediction of a pseudoknot interaction in the SPlV5 and BNYVV xrRNA motifs, showing the designation of stem elements S1 and S2, and loop elements L1 and L2. Dotted lines between S1 and L2 depict predicted tertiary interactions of adenosines with the S1 minor groove. Grey box depicts the L1-S2 pseudoknot interaction that is under consideration here. (C) Denaturing polyacrylamide gels showing results for in vitro Xrn1 digestion assays on constructs that carry the L1-S2 sequences as depicted above. Nucleotides substituted in comparison to the SPlV5 context are given in red. For PK17 and PK18 instead, nucleotides substituted in comparison to the BNYVV context are given in red.
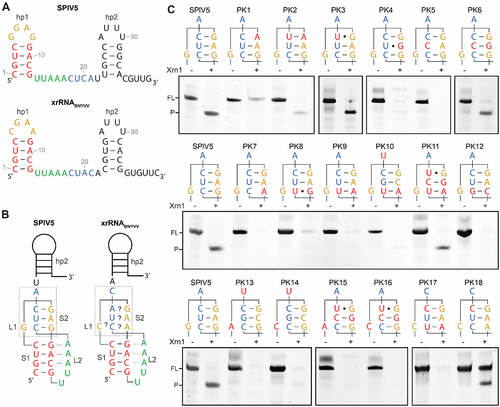
Investigation of the putative pseudoknot interaction
In an attempt to determine whether the predicted nucleotide interactions are indeed formed and responsible for a functional xrRNAWCC, we produced constructs in which these were systematically disturbed and restored, and subjected them to an in vitro Xrn1 degradation assay. Constructs were derived from the predicted S2 interaction GAG – CUC that is present in the SPlV5 candidate xrRNA, in context of the well-established xrRNABNYVV (). shows the structure of this putative pseudoknot composed of stems 1 and 2 (S1, S2) and loops 1 and 2 (L1, L2). Note that hp2, which is required for Xrn1-resistance as well, is not shown to interact with the pseudoknot portion directly, as its role in the overall topology is yet elusive. The putative interaction between S2 nucleotides G6 and C20 was tested by introducing either single G6A or C20U mutations or the combined G6A, C20U mutation, preserving purines and pyrimidines at the indicated positions (). In the case of mutating just the loop nucleotide (PK1), Xrn1 resistance was lost, indicating a perturbed structure. This effect was partially reversed in the double mutant, in which the potential three Watson-Crick pairs were restored (PK2). The C20U-mutation alone however, did not lead to a loss in XR (PK3), possibly due to formation of a G-U base pair, which reproduces the same potential G-U pair as found within the XR Hit1. In a similar fashion, A7G and U19C mutations were introduced in the SPlV5 sequence to establish whether a bp is formed between these nucleotides as well. In this case, both the A7G (PK4) and U19C (PK5) singly mutated constructs lost their Xrn1 stalling capacity. Notably, PK4 almost reproduces the Hit1 S2 interaction, but with the predicted G-U pair in the middle of the loop-linker interaction, potentially serving as a disrupting factor when flanked by two Watson-Crick G-C pairs. A combination of the A7G and U19C mutations did yield a construct that stalled Xrn1 (PK6). These results indicate that within the SPlV5 construct, nucleotides G6 and C20, and A7 and U19, respectively, form Watson-Crick bps that are necessary for xrRNA formation.
Within the context of a S2 pseudoknot stem formed by three Watson-Crick or wobble pairs, the two xrRNA hits found in the frameshifting-based XR screen, and the derived SPlV5 sequence, all contain a putative G-C bp formed by nucleotides G8 and C18. Both G8A (PK7) and C18U (PK8) substitutions perturbed XR. However, combining these two substitutions (PK9) did not restore the structure. The same G-C to A-U substitution was also tested within the context of Hit2 (PK10) and Hit1 (PK11), and here, a small portion of xrRNA was retained by PK11. It was therefore hypothesized that within the SPlV5 (and Hit2) context, an A-U bp confers insufficient stability, which inspired testing for a construct in which the original G-C bp was flipped (PK12). Interestingly, this construct did not stall Xrn1 either, indicating the stringent necessity of the G8 and C18 nucleotides at those positions within SPlV5 ×rRNA, in contrast to Hit1 which do allow for a substitution with an A-U bp here.
The hypothesis of a three-bp pseudoknot stem between nucleotides 6–8 and 18–20 would necessitate that nucleotide 5 bridges the gap as a short L1. Within xrRNABNYVV, nucleotide 5 is a cytosine, which can only be substituted by a uracil in order to maintain XR. In Hit1, Hit2 and SPlV5 ×rRNAWCC, this nucleotide is conserved as a G. Whether it conveys a similar structural function in Watson-Crick-style coremin motifs, or is merely a nucleotide that bridges the gap, was tested by G5A and G5C substitutions from the context of both Hit2 (PK13, PK14) and Hit1 (PK15, PK16). All mutations yielded RNA unable to stall Xrn1, thus suggesting the requirement of a more intricate structural interaction by nucleotide 5 with S2.
The existence of Watson-Crick-style coremin motifs raises the question to what extent they match the structure and topology of BNYVV-style coremin motifs in which no canonical pseudoknot interaction is readily apparent. This topic was investigated by substituting nucleotides 18–20 within the spacer of xrRNABNYVV for those that would match loop nucleotides 6–8 in a canonical Watson-Crick fashion (PK17). For this test, we additionally included a construct that would facilitate only two Watson-Crick bps between nucleotides 6 and 7, and 20 and 19, respectively, through a single A19C substitution (PK18). Surprisingly, only the latter construct proved to be the one that retained XR, although a significant portion of PK18 RNA remained at the same length when treated with Xrn1, which indicates partial misfolding that prevented access to the 5’ end of the leader sequence. Notably, this is the first time a substitution of the conserved spacer nucleotides 18–20 is allowed within the BNYVV-style coremin motifs, whereas previously, an A19U substitution did not yield xrRNA [Citation31].
Hp2 is dispensable for frameshifting by Watson-Crick type coremin motifs
In contrast to XR exhibited by coremin motif xrRNA, small frameshifting pseudoknots do not require a hp2 downstream of the pseudoknot stem for their functioning. Therefore, we sought to investigate the relationship between XR and frameshifting ability for xrRNABNYVV and xrRNAWCC (). Compared to xrRNABNYVV, the xrRNAWCC sequence of Hit2 exhibited a slightly higher frameshifting efficiency (29.8%, ), which was lost almost completely by changing its hp1 loop from GGCG to AGCG, a substitution that yielded a complete loss of Xrn1 resistance (PK13, ). In addition to the hp1 loop mutation tested previously for xrRNABNYVV (), we tested a mutation within the predicted S2 stem (U19C), which yielded a loss of frameshifting capacity similar to that of the AGAA hp1 loop construct (). Subsequently, we tested the frameshifting capacity of xrRNABNYVV and Hit2 without the hp2 that is strictly necessary for XR (Supplementary Figure S2) [Citation31], but which does retain the nucleotides directly involved in the formation of the putative S2. This data shows the retention of frameshifting efficiency in Hit2 when hp2 is removed (30.4%, ), whereas in xrRNABNYVV, the frameshifting efficiency dropped to the level of the mutant construct (2.0%). Furthermore, frameshifting by the mutant construct of Hit2 without hp2 dropped to 2.5%. Overall, these results indicate that, while both xrRNABNYVV and xrRNAWCC exhibit XR and frameshifting stimulation abilities, only xrRNAWCC promotes frameshifting without hp2. The recently discovered variation on the coremin motif, which carries six instead of four lp1 nucleotides (Dilweg et al. submitted for publication), was tested as well. The XR CGAAAA lp1 construct demonstrated a 13.1% efficiency, 9,3% lower than the CGAA lp1 counterpart, whereas the non-XR AGAAAA lp1 dropped to 1.2%. It therefore appears to lose some frameshifting capacity due to the larger hp1 loop.
Figure 4. Determination of frameshifting efficiencies for xrRNAC, xrRNAWCC, xrRNAC with a 6-nt lp1, and corresponding constructs without a hp2. (A) Alignment showing differences between tested constructs, with dashes indicating no deviation from the frameshifting cassette as given for ‘BNYVV wt’, and empty spaces indicating deletions. Slippery sequences are shown italicized, and hp1 and hp2 stems are given in green and red, respectively. (B) Autoradiograms from−1 PRF assay that was repeated for each construct at least in triplicate. For further details, see legend to .
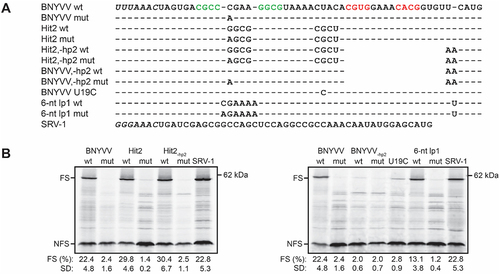
Frameshifter pseudoknots with or without hp2 are not resistant to Xrn1
Since xrRNAWCC appears to utilize a canonical pseudoknot for XR and confers frameshifting without an hp2, we wondered whether other known frameshifting pseudoknots could also stall Xrn1. Therefore, we performed in vitro Xrn1 digestion on constructs containing the BWYV P1-P2 pseudoknot [Citation32], which has comparable dimensions to the xrRNAC hp1 and spacer; the Mouse mammary tumour virus (MMTV) gag-pro pseudoknot [Citation42], which has a short S1 stem; the Avian coronavirus (IBV) pseudoknot [Citation43], which has a long S1 stem, and a version of the SRV-1 pseudoknot with a slightly adjusted S1 compared to the construct used in the −1 PRF assays, but which yielded roughly the same frameshifting efficiency in vitro, namely 24% ( [Citation38,Citation44]). For the BWYV and MMTV pseudoknots, we additionally tested constructs in which the pseudoknot was followed by the xrRNABNYVV hp2, in order to potentially mimic the characteristics needed for XR that were suggested by earlier studies [Citation31]. Expectedly, none of these constructs showed signs of XR (), and therefore it can be assumed that these pseudoknots lack a certain trait that is exhibited by xrRNAs in order to stall Xrn1.
Figure 5. In vitro Xrn1 digestion assays investigating XR of known frameshifting pseudoknots. (A) Grey letters denote the leader present on RNA constructs used in this assay, followed downstream by the S1 (green) and S2 (underlined) elements, in addition to the hp2 element from xrRNABNYVV (red for the stem) for BWYV and MMTV. Sequences used were derived from accession numbers X13063 (BWYV) and D16249 (MMTV), while for IBV the ‘minimal’ pseudoknot in which its 32-nt long, functionally redundant L2 element is shortened, is used [Citation43]. (B) Denaturing polyacrylamide gels showing results for in vitro Xrn1 digestion assays on constructs as given in (A).
![Figure 5. In vitro Xrn1 digestion assays investigating XR of known frameshifting pseudoknots. (A) Grey letters denote the leader present on RNA constructs used in this assay, followed downstream by the S1 (green) and S2 (underlined) elements, in addition to the hp2 element from xrRNABNYVV (red for the stem) for BWYV and MMTV. Sequences used were derived from accession numbers X13063 (BWYV) and D16249 (MMTV), while for IBV the ‘minimal’ pseudoknot in which its 32-nt long, functionally redundant L2 element is shortened, is used [Citation43]. (B) Denaturing polyacrylamide gels showing results for in vitro Xrn1 digestion assays on constructs as given in (A).](/cms/asset/8a1bee06-de41-4c08-b545-401ec8f6ac8c/krnb_a_2205224_f0005_oc.jpg)
Discussion
Similarities and differences between frameshifting pseudoknots and xrRNAC motifs pose an interesting area for comparison, in the absence of clear structural data on the latter. The notion of xrRNAC being able to promote frameshifting was initially inspired by the observed similarity to the BWYV frameshifting pseudoknot. Interestingly, the frameshifting stimulation that was previously determined for the BWYV pseudoknot in an equivalent in vitro system, is two-fold lower than xrRNAC and almost three-fold lower than xrRNAWCC [Citation45]. Since both xrRNAs require an additional downstream hp2 for XR, the fact that xrRNAC needs it for frameshifting, but xrRNAWCC does not, poses an interesting dichotomy. This data appears to suggest that whatever structural advantage the hp2 poses for stalling Xrn1, this is not required for stimulating frameshifting. We could therefore speculate on the role of hp2 in the folding mechanics of these structures. As xrRNAC forms a structure of sufficient stability to stall Xrn1 and ribosomes, perhaps the non-canonical nature of its putative pseudoknot interaction requires a stable, flanking hp2 in order to provide the correct hierarchy of proper folding, and stability, being less dependent on context variations. In contrast, the more canonical nature expected of the putative xrRNAWCC may result in an interaction that is more likely to form, independent of whether hp2 is present.
Keeping in mind the roughly 1.5-fold increase of frameshifting stimulation when using the more efficient UUUAAAC slippery sequence, it follows that xrRNAWCC stimulates frameshifting on par with the SRV-1-PK [Citation46], while xrRNAC reaches about 0.7-fold that level of efficiency. While intriguing from a structural perspective, it should be stressed that in this study, these efficiencies were determined in vitro, under influence of an efficient slippery sequence. As of yet, we are not aware of any xrRNAC that harbours a slippery sequence upstream at a compatible distance of the structure. Moreover, the fact that these motifs are usually located in 3’ UTRs, makes it unlikely that they would encounter a translating ribosome. Recent developments in the identification of new potential xrRNA species however, have challenged that such structures are exclusively present in untranslated regions [Citation21]. Previously, we identified several coremin-like motifs just upstream of the final translation termination site in Umbra- and Poleroviruses (Dilweg et al. submitted for publication). No clear slippery sequences are present upstream of these structures, and they are of the 6-nt lp1 type of xrRNAC, which appear to be 0.6-fold as effective as the tetraloop-variant (). However, the location of these motifs, the additional regulatory possibilities of XR, and the unknown scope of xrRNA species throughout the virome and beyond, do provide an intriguing potential for a frameshifting function of xrRNAs.
In our −1 PRF assay (), both xrRNAC and xrRNAWCC mutant controls were targeted at nucleotide 5, which, in our pseudoknot model, would form the L1 element. This loop in small frameshifting, luteoviral pseudoknots, is inserted into the major groove of the S2, where it participates in a quadruple base pair by associating with the Hoogsteen edge of the G-C pair in S2, coupled with an additional interaction with a flanking adenosine [Citation32,Citation33]. In BWYV, this interaction is crucial for promoting −1 PRF [Citation45]. On the other hand, this is not the case in the SRV-1 pseudoknot, where mutation of the L1 nucleotide did not yield a significant reduction in −1 PRF [Citation46]. In both xrRNAC and xrRNAWCC contexts, the nucleotide predicted to fill in the role of L1 in our pseudoknot model, could not be substituted either. Moreover, XR is lost within both xrRNA types when the same substitutions are applied, with an exception for a C5U substitution that is tolerated for xrRNAC [Citation31]. Altogether, this points towards a similar kind of interaction conferring the stability necessary for XR and frameshifting within the coremin motif. However, the fact that a BWYV pseudoknot with an additional hp2 does not stall Xrn1, indicates that some additional interaction and/or topology is required for conferring XR.
The shared capacity for frameshifting stimulation of xrRNAWCC, xrRNAC, and other regular frameshifting pseudoknots, was by itself an argument for the presence of a pseudoknot interaction in both xrRNA types. Our mutational analysis on xrRNAWCC indicates how indeed, loop nucleotides 6 and 7, likely interact with nucleotides 20 and 19, respectively. Furthermore, the covariation demonstrated by the Hit1, Hit2 and SPlV5 sequences supported the same hypothesis. In contrast, while these three sequences all have a conserved G8-C18 pair, it is not supported as clearly by the data, due to the fact that substituting it for an A-U only restores XR within the context of Hit1. It is possible that the SPlV5 and Hit2 contexts posed a more stringent condition for the 8–18 interaction to hold the more stable G-C bp, possibly due to the key role discussed above for the L1 composed of nucleotide 5. In this way, our results provide additional indications for the nucleotides that are paired in the xrRNAC and xrRNAWCC pseudoknots. However, the identity and positional context appear to be crucial, which is unsurprising considering the expected importance of additional, stabilizing tertiary interactions.
In our previous work on xrRNABNYVV, we identified nucleotides 18–20 as those most conserved within the sequence linking hp1 and hp2, and thus speculated that a non-canonical pseudoknot with additional tertiary interactions was likely involved [Citation31]. In the current study, substituting the same nucleotides in xrRNABNYVV such that an S2 with Watson-Crick bps could be formed, led to the unexpected result of the two-bp S2 construct (PK18) being XR, while the three-bp S2 version (PK17) was not. In fact, PK18 required only a single A20C substitution (predicted to form a G-C bp with lp1 nucleotide 6), which closely resembles the A20U substitution which was tested previously and deemed unable to stall Xrn1. Assuming that the predicted S2 of PK18 involves the same nucleotides, it follows that in the wildtype xrRNABNYVV, a G-A bp is formed instead. Such a bp is widespread throughout all kinds of biological RNA structures, and poses possibilities for distorted helices, or even formation of a metal ion-binding pocket [Citation47–50], both of which could perhaps serve as the unique factor that makes this structure as stable while lacking obvious pseudoknot compatibility. Conversely, the fact that xrRNAWCC and PK18 do not appear to require such a non-canonical bp renders it doubtful that this explains the XR capacity of xrRNAC. Further elucidation of the actual interactions that occur in both xrRNAC and xrRNAWCC will be required in order to compare both pseudoknot types that are employed for gaining XR.
The yield of 28 well-distinguishable sequences from the frameshifting-based XR screen supposedly provides a selection of motifs that promote ribosomal frameshifting to such an extent that enough NLuc is expressed in order to distinguish them from NLuc-negative colonies. However, in assessing the subjectively assigned frameshifting score, and the potential for a pseudoknot interaction between the randomized sequences, no clear correlation can be determined (Supplementary Table S2). Of the 28 sequences, only 11 can potentially form a pseudoknot with an S2 of at least two W-C bps, assuming an L1 of at least one nt. Conversely, we cannot exclude the possibility that divergent XR motifs may have been missed among the sequence combinations that did not allow for a clear W-C-based S2. However, the dissimilarity to xrRNAC and its predicted lp1 consensus being YGAD [Citation31], renders it overall likely that a significant portion of these hits were false positives, indicating that the method employed for selecting NLuc-positive colonies requires further refinement.
Nonetheless, the discovery of xrRNAWCC through our frameshifting-based XR screen is intriguing, although the initial search for such sequences in nature did not yield much. The single hit in SPlV5 on the other hand, was distinctly located just 8 nt downstream of its 3’-most ORF, and was followed by a hp2, which was not a factor (that can be) included in BLAST. This virus has been discovered through transcriptome analysis of freshwater invertebrates, and was putatively assigned to the Picornavirales order on the basis of RdRp-conservation [Citation51]. We previously showed the presence of xrRNAC sequences in the Marnaviridae family of Picornavirales (Dilweg et al. submitted for publication), thus indicating that this order may utilize different types of xrRNA throughout. In order to gauge the potential distribution of xrRNAWCC throughout the virome, we employed the RNABOB tool [Citation52] for getting an idea of how many sequences comply with the structural constraints that were deemed functional here, and in the previous study on xrRNAC [Citation31]. This resulted in large set of potential hits (not shown) of which one piqued our interest in particular, due to the fact that recently, xrRNAC was identified in the 3’ UTRs of three genomic RNAs from a double-stranded RNA virus Alphachrysovirus (Dilweg et al. submitted for publication). This hit was found in another species of Alphachrysovirus, Anthurium mosaic-associated chrysovirus, which bore a sequence with xrRNAWCC characteristics in the 5’ UTR of both its dsRNA2 and dsRNA3. We tested these sequences for XR and found that the dsRNA3 version is indeed a candidate xrRNA (Supplementary Figure S3). The difference in genomic location of xrRNAs between these viral species within the same family poses an interesting case for the evolutionary origin and function of these motifs. It can be assumed that xrRNAWCC stalls scanning ribosomes like xrRNAC does, which implies either a regulatory function on translation, or that internal translation initiation is utilized. Indeed, internal ribosome entry site (IRES)-activity has been demonstrated for two species of Chrysoviridae [Citation53], but it is yet unclear whether such IRES structures are present in Alphachrysoviruses.
Research on xrRNAs over the past decade has started to indicate their variety and broad distribution. However, these findings have also made clear how little is understood about what functions these structures may employ within the large variety of viruses that harbour them, and to what extent these structures are utilized. Here, we introduced a novel variation on the coremin motif xrRNA, which involves a more canonical pseudoknot interaction. In addition, a new potential function that may be conferred by xrRNAs is identified by showing how xrRNAC and xrRNAWCC – and to a lesser extent xrRNAZIKV and xrRNAC carrying a 6-nt lp1 – all have the ability of stimulating frameshifting in vitro. As such, this study again expanded the potential scope and spread of xrRNAs throughout the virome, and perhaps beyond.
Supplemental Material
Download MS Word (530.7 KB)Disclosure statement
No potential conflict of interest was reported by the authors.
Data availability statement
The authors confirm that the data supporting the findings of this study are available within the article and its supplementary materials.
Supplementary material
Supplemental data for this article can be accessed online at https://doi.org/10.1080/15476286.2023.2205224.
Additional information
Funding
References
- Staple DW, Butcher SE. Pseudoknots: RNA structures with diverse functions. PLoS Biol. 2005;3:956–959.
- Brierley I, Pennell S, Gilbert RJC. Viral RNA pseudoknots: versatile motifs in gene expression and replication. Nat Rev Microbiol. 2007;5:598–610. 58. doi: 10.1038/nrmicro1704
- Pleij CWA, Rietveld K, Bosch L. A new principle of RNA folding based on pseudoknotting. Nucleic Acids Res. 1985;13:1717–1731.
- Puglisi JD, Wyatt JR, Tinoco I. Conformation of an RNA pseudoknot. J Mol Biol. 1990;214:437–453.
- Giedroc DP, Theimer CA, Nixon PL. Structure, stability and function of RNA pseudoknots involved in stimulating ribosomal frameshifting. J Mol Biol. 2000;298:167–185.
- Poole TL, Wang C, Popp RA, et al. Pestivirus translation initiation occurs by internal ribosome entry. Virology. 1995;206:750–754.
- Dreher TW, Miller WA. Translational control in positive strand RNA plant viruses. Virology. 2006;344:185–197.
- Jang SK. Internal initiation: IRES elements of picornaviruses and hepatitis c virus. Virus Res. 2006;119:2–15.
- Olsthoorn RCL, Mertens S, Brederode FT, et al. A conformational switch at the 3′ end of a plant virus RNA regulates viral replication. Embo J. 1999;18:4856–4864.
- Ray D, Na H, White KA. Structural properties of a multifunctional T-shaped RNA domain that mediate efficient tomato bushy stunt virus RNA replication. J Virol. 2004;78:10490–10500.
- Brierley I. Ribosomal frameshifting on viral RNAs. J Gen Virol. 1995;76:1885–1892.
- Giedroc DP, Cornish PV. Frameshifting RNA pseudoknots: structure and mechanism. Virus Res. 2009;139:193–208.
- Pijlman GP, Funk A, Kondratieva N, et al. A highly structured, nuclease-resistant, noncoding RNA produced by flaviviruses is required for pathogenicity. Cell Host Microbe. 2008;4:579–591.
- Iwakawa H, Mizumoto H, Nagano H, et al. A viral noncoding RNA generated by cis-element-mediated protection against 5’->3’ RNA decay represses both cap-independent and cap-dependent translation. J Virol. 2008;82:10162–10174.
- Funk A, Truong K, Nagasaki T, et al. RNA structures required for production of subgenomic flavivirus RNA. J Virol. 2010;84:11407–11417.
- Silva PAGC, Pereira CF, Dalebout TJ, et al. An RNA pseudoknot is required for production of yellow fever virus subgenomic RNA by the host nuclease XRN1. J Virol. 2010;84:11395–11406.
- Peltier C, Klein E, Hleibieh K, et al. Beet necrotic yellow vein virus subgenomic RNA3 is a cleavage product leading to stable non-coding RNA required for long-distance movement. J Gen Virol. 2012;93:1093–1102.
- Dilweg IW, Bouabda A, Dalebout T, et al. Xrn1-resistant RNA structures are well-conserved within the genus flavivirus. RNA Biol. 2021;18:709–717.
- Dilweg IW, Savina A, Köthe S, et al. All genera of Flaviviridae host a conserved Xrn1-resistant RNA motif. RNA Biol. 2021;18:2321–2329.
- Moon SL, Blackinton JG, Anderson JR, et al. XRN1 stalling in the 5’ UTR of hepatitis C virus and bovine viral diarrhea virus is associated with dysregulated host mRNA stability. PLOS Pathog. 2015;11:e1004708.
- Steckelberg A, Vicens Q, Kieft JS. Exoribonuclease-resistant RNAs exist within both coding and noncoding subgenomic RNAs. MBio. 2018;9:1–12.
- Schnettler E, Sterken MG, Leung JY, et al. Noncoding flavivirus RNA displays RNA interference suppressor activity in insect and Mammalian cells. J Virol. 2012;86:13486–13500.
- Moon SL, Dodd BJT, Brackney DE, et al. Flavivirus sfRNA suppresses antiviral RNA interference in cultured cells and mosquitoes and directly interacts with the RNAi machinery. Virology. 2015;485:322–329.
- Göertz GP, Fros JJ, Miesen P, et al. Noncoding subgenomic flavivirus RNA is processed by the mosquito RNA interference machinery and determines West Nile virus transmission by Culex pipiens mosquitoes. J Virol. 2016;90:10145–10159.
- Flobinus A, Hleibieh K, Klein E, et al. A viral noncoding RNA complements a weakened viral RNA silencing suppressor and promotes efficient systemic host infection. Viruses. 2016;8:272.
- Chapman EG, Costantino DA, Rabe JL, et al. The structural basis of pathogenic subgenomic flavivirus RNA (sfRNA) production. Science. 2014;344:307–310.
- Akiyama BM, Laurence HM, Massey AR, et al. Zika virus produces noncoding RNAs using a multi-pseudoknot structure that confounds a cellular exonuclease. Science. 2016;354:1148–1152.
- Steckelberg A, Akiyama BM, Costantino DA, et al. A folded viral noncoding RNA blocks host cell exoribonucleases through a conformationally dynamic RNA structure. PNAS. 2018;115:6404–6409.
- Jones RA, Steckelberg A, Szucs MJ, et al. Different tertiary interactions create the same important 3-D features in a divergent flavivirus xrRNA. RNA. 2020;27:54–65.
- Zhao M, Woodside MT. Mechanical strength of RNA knot in Zika virus protects against cellular defenses. Nat Chem Biol. 2021;17:975–981. 179. doi: 10.1038/s41589-021-00829-z
- Dilweg IW, Gultyaev AP, Olsthoorn RC. Structural features of an Xrn1-resistant plant virus RNA. RNA Biol. 2019;16:838–845.
- Su L, Chen L, Egli M, et al. Minor groove RNA triplex in the crystal structure of a ribosomal frameshifting viral pseudoknot. Nat Struct Biol. 1999;6:285–292.
- Pallan PS, Marshall WS, Harp J, et al. Crystal structure of a luteoviral RNA pseudoknot and model for a minimal ribosomal frameshifting motif. Biochemistry. 2005;44:11315–11322.
- Plant EP, Jacobs KLM, Harger JW, et al. The 9-Å solution: how mRNA pseudoknots promote efficient programmed −1 ribosomal frameshifting. RNA. 2003;9:168–174.
- Chernyakov I, Whipple JM, Kotelawala L, et al. Degradation of several hypomodified mature tRNA species in Saccharomyces cerevisiae is mediated by Met22 and the 5-3 exonucleases Rat1 and Xrn1. Genes Dev. 2008;22:1369–1380.
- Jinek M, Coyle SM, Doudna JA. Coupled 5’ nucleotide recognition and processivity in Xrn1-mediated mRNA decay. Mol Cell. 2011;41:600–608.
- Nagarajan VK, Jones CI, Newbury SF, et al. XRN 5′→3′ exoribonucleases: structure, mechanisms and functions. Biochim Biophys Acta - Genet Regul Mech. 2013;1829:590–603.
- Michiels PJ, Versleijen AA, Verlaan PW, et al. Solution structure of the pseudoknot of SRV-1 RNA, involved in ribosomal frameshifting. J Mol Biol. 2001;310:1109–1123.
- Brierley I, Jenner AJ, Inglis SC. Mutational analysis of the “slippery-sequence” component of a coronavirus ribosomal frameshifting signal. J Mol Biol. 1992;227:463–479.
- Dixon AS, Schwinn MK, Hall MP, et al. NanoLuc complementation reporter optimized for accurate measurement of protein interactions in cells. ACS Chem Biol. 2016;11:400–408.
- Milligan JF, Groebe DR, Witherell GW, et al. Oligoribonucleotide synthesis using T7 RNA polymerase and synthetic DNA templates. Nucleic Acids Res. 1987;15:8783–8798.
- Chamorro M, Parkin N, Varmus HE. An RNA pseudoknot and an optimal heptameric shift site are required for highly efficient ribosomal frameshifting on a retroviral messenger RNA. PNAS. 1992;89:713–717.
- Brierley I, Rolley NJ, Jenner AJ, et al. Mutational analysis of the RNA pseudoknot component of a coronavirus ribosomal frameshifting signal. J Mol Biol. 1991;220:889–902.
- Yu CH PhD thesis. Leiden University; 2011.
- Kim YG, Su L, Maas S, et al. Specific mutations in a viral RNA pseudoknot drastically change ribosomal frameshifting efficiency. PNAS. 1999;96:14234–14239.
- Ten Dam EB Verlaan PWG, Pleij CWA, et al. Analysis of the role of the pseudoknot component in the SRV-1 gag-pro ribosomal frameshift signal: loop lengths and stability of the stem regions. RNA. 1995;1:146–154.
- Baeyens KJ, De Bondt HL, Pardi A, et al. A curved RNA helix incorporating an internal loop with G·A and A·A non-Watson-Crick base pairing. PNAS. 1996;93:12851–12855.
- Klein DJ, Schmeing TM, Moore PB, et al. The kink-turn: a new RNA secondary structure motif. Embo J. 2001;20:4214–4221.
- Chen G, Znosko BM, Kennedy SD, et al. Solution structure of an RNA internal loop with three consecutive sheared GA pairs. Biochemistry. 2005;44:2845–2856.
- Olson WK, Li S, Kaukonen T, et al. Effects of noncanonical base pairing on RNA folding: structural context and spatial arrangements of G·A pairs. Biochemistry. 2019;58:2474–2487.
- Shi M, Lin XD, Tian JH, et al. Redefining the invertebrate RNA virosphere. Nat. 5407634. 2016;540:539–543.
- Eddy SR RNABOB: a program to search for RNA secondary structure motifs in sequence databases. http://selab.janelia.org/software.html
- Chiba S, Jamal A, Suzuki N. First evidence for internal ribosomal entry sites in diverse fungal virus genomes. MBio. 2018;9: e02350-17.