ABSTRACT
Study of the timing and location for mRNA translation across model systems has begun to shed light on molecular events fundamental to such processes as intercellular communication, morphogenesis, and body pattern formation. In D. melanogaster, the posterior mRNA determinant, oskar, is transcribed maternally but translated only when properly localized at the oocyte’s posterior cortex. Two effector proteins, Bruno1 and Cup, mediate steps of oskar mRNA regulation. The current model in the field identifies Bruno1 as necessary for Cup’s recruitment to oskar mRNA and indispensable for oskar’s translational repression. We now report that this Bruno1-Cup interaction leads to precise oskar mRNA regulation during early oogenesis and, importantly, the two proteins mutually influence each other’s mRNA expression and protein distribution in the egg chamber. We show that these factors stably associate with oskar mRNA in vivo. Cup associates with oskar mRNA without Bruno1, while surprisingly Bruno1’s stable association with oskar mRNA depends on Cup. We demonstrate that the essential factor for oskar mRNA repression in early oogenesis is Cup, not Bruno1. Furthermore, we find that Cup is a key P-body component that maintains functional P-body morphology during oogenesis and is necessary for oskar mRNA’s association with P-bodies. Therefore, Cup drives the translational repression and stability of oskar mRNA. These experimental results point to a regulatory feedback loop between Bruno 1 and Cup in early oogenesis that appears crucial for oskar mRNA to reach the posterior pole and its expression in the egg chamber for accurate embryo development.
Introduction
Post-transcriptional gene regulation is important for complex processes, such as cell motility, synaptic plasticity, and organism development. This regulation is primarily dictated by the dynamic associations between messenger RNA (mRNA) and proteins (mRNP) throughout the mRNA’s life. In the Drosophila melanogaster egg chamber, oskar (osk) is critical for establishing the developmental patterning along with the future germline [Citation1]. A myriad of proteins forms a large mRNP involved in the stability, transport, localization, translational repression, and translational activation of osk mRNA. Two of these proteins, Cup and Bruno 1 (Bru1), have been proven necessary for precise osk mRNA regulation [Citation2–5]. However, the dynamics of their spatial and temporal interactions have remained elusive.
Bru1 is a member of the CELF superfamily (CUGBP and Elav-like family) which directly binds osk mRNA’s 3’ UTR at two regions: a combined A|B site and a C site, where multiple Bru1 response elements (BREs) are located [Citation3,Citation6]. Repression of osk mRNA translation requires both regions, and the importance of BREs was demonstrated by several studies carried out during mid-to-late oogenesis [Citation3,Citation7]. Interestingly, the C site is also involved in the translational activation of osk mRNA at the posterior of the oocyte [Citation7], yet whether this activation is dependent on osk’s localization is not fully understood. Bru1 has two RNA-recognition domains, RRM 1 + 2, and an extended RRM 3 that are important for binding osk mRNA and other maternal mRNAs in vitro [Citation8]. Bru1 expression must be maintained at a critical threshold, as either loss-of- function or overexpression of Bru1 led to similar defects, both being detrimental for egg chamber development [Citation6,Citation9]. Bru1 can dimerize through its N-terminal domain and without this domain, osk mRNA granules do not form, and osk mRNA is not translated [Citation10,Citation11]. Interestingly, this is also one of the domains through which Bru1 directly binds to Cup with very high affinity [Citation2]. Altogether, these observations raise the question of a possible additional mechanism of osk mRNA translational regulation where Cup binding to Bru1’s N-terminal domain leads to osk mRNA translational repression, but the absence of Cup binding to Bru1 leads to osk mRNA translation.
Cup is a canonical eIF4E binding protein (4E-BP) that blocks translation initiation of mRNAs [Citation4,Citation5,Citation12]. Cup also interacts with the CCR4-Not complex to allow for the deadenylation of mRNAs while simultaneously blocking decapping enzymes to maintain a translationally silent and stable mRNA [Citation13]. For osk mRNA, the current model suggests that Bru1 recruits Cup to the transcript, forming a Bru1-Cup-eIF4E translational repression complex [Citation4,Citation5]. Cup has also been identified as a processing body (P-body) component due to its association with two core P-body proteins, Me31B and Trailer Hitch (Tral) [Citation4,Citation14]. P-bodies are membraneless organelles that form via liquid–liquid phase separation. They are composed of proteins that contain intrinsically disordered regions (IDR), mRNAs, and mRNA-binding factors (reviewed in [Citation15]). Formation of P-body condensates is not fully understood, but their integrity and the physical state they adopt are regulated by structurally distinct proteins, such as Me31B and Tral [Citation16]. Though previously thought to be sites of mRNA turnover, recent research demonstrates that P-bodies are also storage hubs for translationally silenced mRNAs (reviewed in [Citation17]) which get released into the cytoplasm upon developmental cues or as a result of disrupted condensate organization [Citation16]. osk mRNA is one of the several post-transcriptionally regulated mRNAs stored in P-bodies [Citation18,Citation19], but the importance of this association for osk’s stability and translational repression has yet to be addressed during early oogenesis.
Here, we bring new insights into the regulation of osk mRNA translational repression. We show that both Bru1 and Cup associate with osk mRNP in the nurse cells and travel together into the oocyte. We demonstrate that Cup is able to join osk mRNP and actually repress its translation in the absence of Bru1, and surprisingly, Cup is necessary to stabilize the interaction between osk mRNA and Bru1. Interestingly, we also reveal a strict codependence between Bru1 and Cup stability and distribution in the egg chamber. Furthermore, we studied the role of Bru1 and Cup in osk mRNA recruitment into P-bodies during early oogenesis. Our investigation on the connection between Cup and osk mRNA translational repression identified Cup as a crucial cellular component that regulates Me31B-condensates physical state, which in turn, possibly ensures proper P-body function.
Results
Bru1 and Cup maintain a stable association with osk mRNA in the egg chamber
The Drosophila melanogaster egg chamber is an excellent system to study important details of the spatio-temporal events that occur during post-transcriptional regulation. Drosophila oogenesis spans from the germarium through 14 stages of egg-chamber development that here we referred to as early (1–4), mid (5–9) and late (10–14) (). The egg chamber consists of 16 germline cells, including a single oocyte and 15 nurse cells surrounded by somatic follicle cells (reviewed in [Citation20]). Nurse cells supply organelles, factors, and key transcripts to the transcriptionally repressed oocyte. osk mRNA moves as a translationally silenced mRNP from the nurse cells into the oocyte, where it eventually anchors tightly at the posterior cortex ( green) [Citation21]. Translation of osk mRNA occurs only at the posterior pole ( red). Premature or ectopic expression of Osk is lethal to the embryo [Citation22,Citation23]. Moreover, osk mRNA itself is crucial for progression through oogenesis, with its absence leading to arrest in egg chamber development during mid-oogenesis [Citation24].
Figure 1. Bru1 and Cup maintain a stable association with osk mRNA in the nurse cells and oocyte throughout oogenesis. (A) Drosophila melanogaster ovariole depicting developmental stages from germarium to stage 10. osk mRNA (green), Osk protein (red), F-actin (magenta) and membranes/WGA (white). (B, C) Co-visualization of osk mRNA and Bru1-GFP in stage 8 nurse cells, detected with osk-specific molecular beacons (B) or smFISH probes (C). (B) Colocalization analysis in live egg chambers at the 10 min time point of an 18 min XYZCt series taken every 30 sec. Circles indicate osk mRNA (red) and colocalization of osk mRNA with Bru1-GFP (yellow). Tracked colocalized spots shown as a 3D representation. (C) Images of a fixed egg chamber acquired at 100 × . Images are deconvolved XY max-intensity Z-projections of 7 (live) and 16 (fixed) optical slices (0.3 µm each). (D, E) Co-visualization of osk mRNA and Cup-YFP in a nurse cell, detected with osk-specific molecular beacons (D) or smFISH probes (E). (D) Colocalization analysis in live stage 9 egg chamber at the 7 min time point of a 20.5 min XYZCt series taken every 30 sec. Circles indicate osk mRNA (red) and colocalization of osk mRNA with Cup-YFP (yellow). Tracked colocalized spots are shown as a 3D representation. (E) Images of a fixed stage 8 egg chamber acquired at 100 × . Images are deconvolved XY max-intensity Z-projections of 12 (live) and 6 (fixed) optical slices (0.5 and 0.3 µm each), respectively. Scale bars, 10 μm.
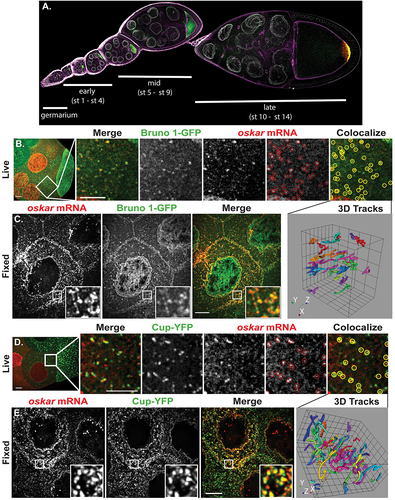
Bru1’s direct binding to osk mRNA has only been demonstrated via biochemical assays [Citation3,Citation7,Citation8,Citation10,Citation25]. We carried out live and fixed-cell experiments to elucidate the spatio-temporal requirements for Bru1 recruitment to osk mRNA, using osk-specific molecular beacons and smFISH probes, respectively. To define the dynamics of osk mRNA and Bru1-GFP association in live egg chambers, we chose to use the previously published, endogenously tagged, homozygous lethal, Bru1-GFP fly line [Citation11,Citation26]. We also confirmed via immunofluorescence (IF) studies that an anti-Bru1 antibody signal is colocalized with the GFP signal (Fig. S1A), indicating that the localization of Bru1-GFP reports on that of endogenous Bru1. We analysed osk mRNA colocalization with Bru1-GFP in both the nurse cells’ cytoplasm and the ooplasm at mid stages after nurse cell microinjections with five different osk-specific molecular beacons () [Citation27,Citation28]. Using the object-based image analysis software Icy [Citation29], we found that 57 ± 3% (n = 5) of osk-containing particles associated with Bru1-GFP in the nurse cells, and 43 ± 4% (n = 6) in the oocyte (, S1B). Tracking analyses revealed that colocalized particles travelled together for an average of 8.5 ± 1 min with an average total displacement of 37 ± 5 µm (n = 11), indicating a stable association between osk mRNA and Bru1-GFP (3D tracks in , S1B; Movies S1 and S2). At high magnification, large mRNP particles were observed in fixed egg chambers, indicating the association of osk mRNA and Bru1 as multiple copies in both the nurse cell cytoplasm and the oocyte, which showed colocalization at 45 ± 1% (n = 14) similar to our live-cell analysis (, S1B). A caveat of object-based analysis of large mRNP particles in ICY is that it can lead to an underestimation of the degree of colocalization [Citation29]. Nevertheless, together these two approaches demonstrate a stable association of osk mRNP with Bru1 in the nurse cells and the oocyte.
In contrast to Bru1, Cup does not contain known RNA-binding domains, nor is there any evidence for direct binding of Cup to osk mRNA. Instead, binding between Cup and osk mRNP was proposed to occur through association with other proteins, including Bru1 [Citation4,Citation5]. However, the precise details of spatial and temporal recruitment of Cup to osk mRNP are unresolved. Previous IF experiments indicated that Cup localizes at the germline cell periphery [Citation30]. However, endogenously tagged Cup-YFP [Citation31] demonstrated the presence of Cup throughout the nurse cells and oocyte cytoplasm in various size condensates, indicating the limitation of antibody accessibility. Colocalization of osk mRNA with Cup-YFP in the cytoplasm of live nurse cells averaged 59 ± 2% (n = 5) and in the ooplasm 73 ± 3% (n = 5) (, S1C). These stable complexes travelled over 50 ± 6 µm (average displacement) for an average time period of 7 ± 1 min (3D tracks in , S1C; Movies S3, S4). At higher magnification, osk mRNA colocalized with Cup-YFP (57 ± 1% n = 20) and formed large puncta in the nurse cells’ cytoplasm and ooplasm during mid-oogenesis in fixed egg chambers (, S1C). Together, the image analysis results for live and fixed egg chambers demonstrate that Cup is a core member of the osk mRNP complex within the nurse cells’ cytoplasm and ooplasm prior to osk’s anchoring at the posterior cortex.
In addition, we analysed the association of Bru1 with Cup and consequently between osk mRNA/Bru1/Cup. Colocali-zation analyses of Bru1 with Cup resulted in an average 55 ± 7% (n = 10) in stage 4/5 egg chambers. Under stringent selection of the ICY software, and only assessing particles with both Bru1 and Cup, we found that osk mRNA colocalizes with this complex at an average of 42 ± 3% (n = 10), underscoring that both proteins are part of osk mRNA during oogenesis. We also carried out fluorescence intensity-line profiling to further demonstrate the high degree of colocalization among these three factors in the nurse cells (Fig. S1D).
Bru1 affects Cup protein and osk mRNA expression levels, but not their colocalization
It was previously suggested that Bru1 is necessary for recruiting Cup to the osk mRNP [Citation4,Citation5]. To test this hypothesis, we utilized Gal4 drivers under the control of different promoters to identify the most efficient and controlled timing of bru1 knockdown. In strong Bru1 mutants, it has been shown that Bru1 is crucial for the development of the germarium [Citation32]. Therefore, it was necessary to choose Gal4 drivers that initiate the knockdown of Bru1 after the germarium stage. The otu-Gal4 driver expresses very low Gal4 levels in the germarium, and development of bru1RNAi egg chambers arrests around stage 4/5. This driver circumvents the limitations of strong Bru1 mutants, making it ideal for studying Bru1’s role during early oogenesis, after the egg chambers progress through the germarium (Fig. S2A). The efficiency of bru1 knockdown was confirmed via IF, where a strong Bru1 signal was detected in the germarium (Fig. S2A arrow), with only background-level signal detected in egg chambers starting at stage 1/2 (Fig. S2A). We confirmed the knockdown efficiency of two bru1RNAi lines and used these interchangeably throughout our study.
To assess whether Cup can be associated with osk mRNP in the absence of Bru1, we generated fly lines expressing Cup-YFP in an otu-Gal4>bru1RNAi background. For our analysis, we selected egg chambers where Bru1 levels were severely reduced; with such strong depletion of Bru1, a change in the osk mRNA/Cup colocalization would be expected if Bru1 were crucial for Cup recruitment to osk mRNA. On the contrary, we found that the osk mRNA/Cup colocalization percentage was 35 ± 1% in wild type (wt) () and remained unchanged in egg chambers with bru1RNAi (37 ± 2%) () background, indicating that, during early oogenesis, Cup can be recruited to osk mRNP via proteins other than Bru1. Using osk smFISH probes in Bru1-depleted egg chambers, we found that the osk mRNA signal was decreased as compared to wt ( vs A) We confirmed our visual observations with RT-qPCR analysis. For preparation of whole ovary lysates, we chose a stronger homogeneously expressed Gal4 driver, maternal tub-alpha (V37). This driver allows for germarium development, as it initiates the knockdown at stage 2, and presents full developmental arrest by stage 3/4. We found that in bru1RNAi egg chambers, osk mRNA level was reduced to 18 ± 7% of the level detected in wt, indicating that Bru1 is actually necessary for maintaining normal osk mRNA levels ().
Figure 2. Reduced Bru1 levels affect Cup and osk mRNA expression levels, but not their colocalization. (A, B) osk mRNA and Bru1 co-visualized via smFISH and if in stage 2 and 3 egg chambers expressing Cup-YFP in wt (A) or bru1RNAi (B) backgrounds. Red arrowhead = oocyte. Images are deconvolved XY max-intensity Z-projections of 17 optical slices (0.3 µm each). (C) Colocalization percentage of osk mRNA with Cup-YFP in wt and bru1RNAi egg chambers (wt n = 9, bru1RNAi n = 11, ns = not significant). (D) RT-Qpcr quantification of endogenous osk mRNA in the indicated backgrounds normalized to rp49 mRNA (mean ± SEM; ****p < 0.0001; mCherryRNAi n = 4, bru1RNAi n = 4). (E-G) Chain of egg chambers expressing Cup-YFP and various levels of bru1 knockdown analysed via IF, with the starred egg chamber showing lower knockdown efficiency and increased Bru1 and Cup-YFP signal (E). Fluorescence intensity analysis of a stage 5 egg chamber expressing Cup-YFP and different levels of bru1RNAi in neighbouring nurse cells as detected with if (F). White arrow outside the box of the Merge image shows the direction of intensity analysis (G). Images are XY max-intensity Z-projections of 26 (E: 40×) and 18 deconvolved (F: 100×) optical slices (0.3 µm each). (H) Immunoblot against GFP and α-Tubulin using lysates prepared from egg chambers expressing Cup-YFP in the indicated backgrounds. Scale bars, 10 μm.
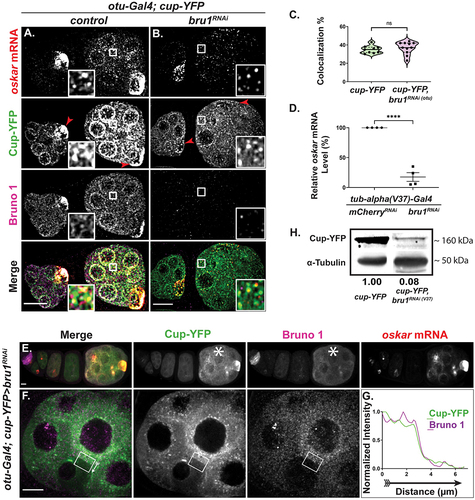
Though it was previously reported that osk mRNA stability was not mediated by Bru1 through the BRE sites [Citation24], we speculated that in BRE mutants, Bru1 is still recruited to the transcript via Cup, thus maintaining osk mRNA stability. Since independent binding of Cup to osk mRNA has not yet been demonstrated, it prompted us to search for factors that would recruit Cup to osk mRNP. Cup also interacts with eIF4E and Barentsz (Btz), two known components of osk mRNP [Citation5]. Systematic knockdowns of paired factors demonstrated that when pairing bru1RNAi with either btzRNAi or eIF4ERNAi, egg chambers arrest at a stage similar to bru1 knockdown alone (Fig. S2B vs 2A) without any impact on osk mRNA/Cup-YFP colocalization observed in wt egg chambers [35 ± 1% (wt n = 9) vs 36 ± 2% (bru1RNAi: btzRNAi n = 6) vs 30 ± 2% (bru1RNAi: eIF4ERNAi n = 5)]. Simultaneous knockdown of btz and eIF4E arrested egg chamber development mid-oogenesis and had no effect on osk mRNA/Cup-YFP colocalization [56 ± 1% (wt n = 10) vs 59 ± 1% (btzRNAi: eIF4ERNAi n = 7)] (Fig. S2B). These results suggest Cup’s recruitment to osk mRNP may be redundant or yet involve other protein factors. An intriguing speculation is that the IDRs of Cup may actually facilitate direct binding to osk mRNA, as IDR domains are known to bind mRNAs in other species [Citation33]. This speculation was supported by results of a recent study that suggest that Cup can directly bind to mRNAs without any other factors [Citation34].
Surprisingly, we also found that Cup-YFP expression was decreased in bru1RNAi egg chambers and the YFP signal was no longer concentrated in the oocyte ( vs 2B, arrowheads). This was unexpected, since there were no previous implications for Bru1 in the regulation of Cup expression. To confirm Bru1’s possible role in cup gene expression regulation, we exploited the heterogenous levels of otu-Gal4 expression across egg chambers, where a few egg chambers ‘escaped’ and developed further () while in some instances neighbouring nurse cells presented varying degrees of the knockdown, thus creating mosaics (). Convincingly, Cup expression levels are directly correlated with those of Bru1. In egg chambers where Gal4 expression led to a weaker bru1 knockdown and persisting Bru1 signal, Cup-YFP levels were also higher (, asterisk). Furthermore, in neighbouring mosaic nurse cells with different bru1 knockdown efficiencies, Bru1 and Cup signal intensities remained directly correlated (), as did Bru1 and osk mRNA signals (Figs S2C, S2D). Western blot analysis confirmed a severe decrease of Cup protein to 8% when Bru1 levels were reduced with the homogeneous expressing Gal4 driver (Gal4V37) (). Despite both Cup and osk mRNA levels drastically decreasing, their remaining levels maintain the same rate of colocalization in bru1 knockdown egg chambers as compared to wt (). Taken together, we unveiled Bru1’s importance for both osk mRNA and Cup stability during early-mid oogenesis.
Cup regulates Bru1 protein expression, distribution, and its association with osk mRNP
Bolstered by our findings that Bru1 is not necessary for Cup’s recruitment to osk mRNA during early oogenesis, we sought to investigate whether Cup mediates the effects of Bru1 on osk mRNA regulation. Cup has been previously shown to interact with numerous proteins and post-transcriptionally regulate multiple mRNAs including osk mRNA [Citation4,Citation35–38].
We evaluated the knockdown of cup using the otu-Gal4 driver and found that Cup expression is highly reduced in egg chambers beginning at stage 1/2 (Fig. S3A arrow indicates signal in germarium). cupRNAi egg chambers phenocopy strong cup mutants [Citation30], developing with almost wild-type morphology until stage 5, and rarely progressing past stage 7. In cupRNAi egg chambers, osk mRNA no longer accumulates into large foci, appearing smaller in size in the nurse cell cytoplasm, especially during mid oogenesis ( vs 3B, 3C vs 3D). We also observed that osk mRNA levels were decreased in cup knockdowns (reduced to 22 ± 6% of wt) (Fig. S3B) just as previous work showed in cup mutant egg chambers [Citation39].
Figure 3. Bru1 protein expression, distribution, and association with osk mRNP are regulated by Cup. (A-D) osk mRNA and Cup detected via smFISH and if in early and mid-stage egg chambers expressing Bru1-GFP in wt or cupRNAi. Early oogenesis, wt (A) and cupRNAi (B). Mid oogenesis, wt (C) and cupRNAi (D). Green arrows highlight the nuclear aggregation of Bru1-GFP in cupRNAi egg chambers. Red arrowheads = oocyte. Images are deconvolved XY max-intensity Z-projections of 19 (wt) and 16 (cupRNAi) optical slices (0.3 µm each) during early, and 22 (wt) and 16 (cupRNAi) during mid stage egg chambers. (E) Colocalization percentage of osk mRNA with Bru1-GFP in wt and cupRNAi backgrounds during early oogenesis (**p < 0.0021, ****p < 0.0001; wt vs cupRNAi: n = 5 and n = 8). (F) Colocalization percentage of osk mRNA with Bru1-GFP in wt and cupRNAi backgrounds during mid oogenesis (**p < 0.0021, ****p < 0.0001; wt vs cupRNAi: n = 6 and n = 8). (G) Immunoblot against Bru1 and α-Tubulin using lysates prepared from egg chambers in indicated backgrounds. (H, I) Fluorescence intensity analysis of a stage 6 egg chamber expressing Bru1-GFP and different levels of cupRNAi knockdown in neighbouring nurse cells, detected with IF. Green arrows highlight nuclear aggregation of Bru1-GFP (G). White arrow outside the box of the Merge image shows the direction of intensity analysis (H). Images are deconvolved XY max-intensity Z-projections 16 optical slices (0.3 µm each). (J) RT-Qpcr quantification of endogenous cup mRNA and bru1 mRNA in the indicated backgrounds normalized to rp49 mRNA (mean ± SEM; ****p < 0.0001; mCherryRNAi n = 5, bru1RNAi n = 5 mCherryRNAi n = 4, cupRNAi n = 4). Scale bars, 10 μm.
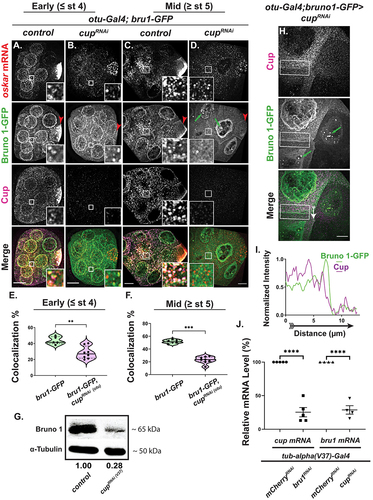
Until stage 3 of oogenesis, Bru1-GFP signal became slightly more diffused in the nurse cells, but its intensity remained comparable to wt ( vs 3A), however it no longer concentrated in the oocyte ( vs 3A, 3D vs 3C, red arrowheads). Beginning around stage 4 and persisting during mid-oogenesis, Bru1-GFP expression became highly diffused and no longer formed large puncta in the nurse cells’ cytoplasm ( vs 3C). Intriguingly, Bru1-GFP formed extremely large and highly concentrated puncta in the nurse cell nuclei that did not include osk mRNA (, S3C, green arrows). We confirmed the nuclear and cytoplasmic localization of endogenous Bru1 in cupRNAi background via IF in order to ensure that the endogenous protein displays the same phenotype as the GFP-tagged protein (, S3D, S4A). When the full volume of the nurse cell nuclei was visualized, the large nuclear aggregation phenotype was highly penetrant with either Bru1 antibody (, green arrows) or Bru1-GFP (, S3C, green arrows).
Figure 4. Cup directs translational silencing, while Bru1 stimulates translational activation of osk mRNA during early stages of oogenesis. (A-D) Cup and Bru1 visualized via if in egg chambers expressing Osk-GFP in the indicated RNAi backgrounds, wt (A), bru1RNAi (B), cupRNAi (C), and bru1RNAi;cupRNAi (D). Green arrows highlight nuclear aggregation of Bru1; some of the nuclei were outlined for clarity. Images are deconvolved XY max-intensity Z-projections of 29 (wt), 30 (cupRNAi), 27 (bru1RNAi) and 34 (bru1RNAi;cupRNAi) optical slices (0.3 µm each). (E) Inset from (C) showing premature Osk-GFP and Bru1 colocalization. (F) Nurse cells expressing Osk-GFP with different levels of cupRNAi knockdown in neighbouring nurse cells delineated by a dashed line, detected with IF. Green arrows highlight nuclear aggregation of Bru1. Image is XY max-intensity Z-projection of 34 optical slices (0.3 µm each). Scale bars, 10 μm.
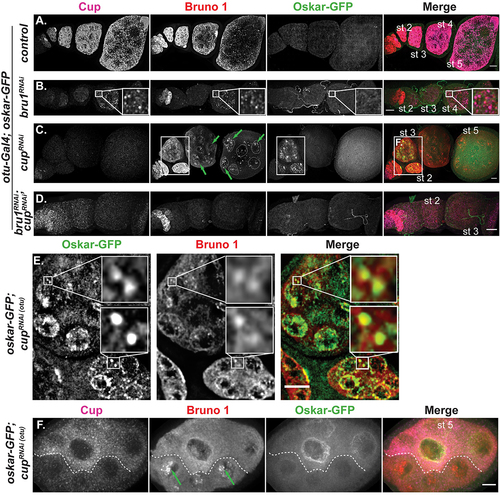
Since Bru1’s level and cytoplasmic localization were altered in cupRNAi egg chambers, we analysed the colocalization of osk mRNA with Bru1-GFP. In early stages, osk mRNA colocalization with Bru1-GFP only moderately decreased (44 ± 2% vs 28 ± 3%) (); however, in stage 5 or older egg chambers, the colocalization was strongly reduced (52 ± 1% vs 23 ± 2%) (). When comparing these results with our live cell imaging data, we observed similar distribution patterns (Movie S5). Taken together, these data indicate that Cup plays a crucial role in the proper formation of osk mRNP, and in the proper localization of Bru1.
The diffused Bru1 expression phenotype raises the question of whether the decrease in Bru1-GFP signal intensity represents a decrease in total Bru1 protein or merely its redistribution in the egg chamber, as previously suggested [Citation39]. To help answer this question, we carried out Western blot analysis for endogenous Bru1 in cupRNAi, using the stronger/homogeneous Gal4 driver (Gal4V37), which led to an early developmental arrest (~stage 5/6) () and found a 72% decrease from wt. Our results contrast with previous Western blot analyses of mutant cup egg chambers where Bru1 levels remained unchanged. However, the Broyer lab did find a reduction in the Bru1 signal during early oocyte stages similar to our IF experimental results [Citation39]. Different experimental methodologies (e.g. the use of mutant cup egg chambers and different antibodies for Western blots) make a direct comparison between these results difficult. Importantly, however, we were able to confirm the same decrease in Bru1 levels in cup knockdown egg chambers using the otu-Gal4 driver and an anti-GFP antibody in our Western blot analysis. We also detected two Bru1-GFP bands, indicating a possible post-translational modification of Bru1, in agreement with a previously proposed hypothesis (Fig. S3D) [Citation6]. To further confirm that Bru1-GFP levels indeed decreased in cupRNAi egg chambers, we analysed Bru1-GFP levels in mosaic cupRNAi egg chambers and found that nurse cells with a lower intensity Cup signal also showed a decreased Bru1-GFP signal in the cytoplasm, with large, concentrated puncta visible in the nuclei (, green arrows, 3I). We found a similar reduction of endogenous Bru1 between mosaic nurse cells in cupRNAi background (Fig S3E, red arrow). Our results illuminate a new and critical role for Cup in maintaining normal Bru1 expression during early oogenesis. Furthermore, since the decrease of both osk mRNA and Bru1 is comparable (22% and 28% respectively) in cup knockdown egg chambers, we expected their colocalization to be maintained, but instead, we found a significant decrease in their colocalization indicating that Cup is important for their association.
We decided to further investigate whether Cup regulates Bru1 at the protein or mRNA level. Interestingly, we found that when Cup levels were reduced, bru1 mRNA levels were significantly decreased (29 ± 6%) (), bringing credence to our Western blot results (reduced to 28% of wt) as the levels at which they decreased are directly correlated. Moreover, we also demonstrated that Bru1 is necessary to maintain cup‘s normal mRNA levels (reduced to 25 ± 7% of wt) (). Remarkably, this indicates a surprisingly reciprocal regulation where Cup and Bru1 control each other’s expression at the mRNA level.
Cup directs translational silencing during early oogenesis, while Bru1 stimulates translational activation of osk mRNA
Cup’s role in the translational repression of osk mRNA has only been established in the oocyte during mid to late oogenesis [Citation4]. Premature Osk protein expression in bru1 mutants has never been detected, with Bru1’s role in translational repression only demonstrated using transgenes and ovary lysates [Citation3,Citation7,Citation8,Citation10,Citation25]. We aimed to clarify the roles of both factors in the translational regulation of osk mRNA during early stages of oogenesis by using egg chambers that express endogenously tagged Osk-GFP. We confirmed that Osk-GFP mimics the wild-type protein expression via an anti-Osk antibody (Figs S4A, S4B). Surprisingly, both Osk-GFP and the anti-Osk antibody enabled us to detect very low Osk levels during earlier stages, which were visualized as colocalized signals (Figs S4C, S4D). Since such a premature and ectopic Osk signal has never been reported, it is possible that it was interpreted to be non-specific due to the quality of the Osk antibodies, as well as the reduced resolution of the detection approaches. This presents a new idea of a possible ‘leaky’ control of osk mRNA translational repression. While the antibody is reliable for detecting Osk protein, it generates a strong background signal, especially during earlier stages of oogenesis where we focused our study, therefore we proceeded using the tagged protein for our analysis. Once again, we chose the otu-Gal4 driver to minimize the knockdown of Bru1 in the germarium, allowing us to only address the early stages of oogenesis.
To our surprise, premature Osk-GFP was not detected when Bru1 levels were decreased in early-stage egg chambers, an indication that osk mRNA translation remained repressed ( vs 4B). Cup expression was reduced in these egg chambers, but it nevertheless persisted and remained colocalized with osk mRNA even when Bru1 levels were no longer detectable as demonstrated above (see vs 2A, 2C). In egg chambers with less efficient bru1 knockdown, Cup and Bru1 were still colocalized ( inset). Altogether, this suggests that Cup is sufficient to maintain the translational repression of osk mRNA (). Confirming this finding, we could not detect premature Osk-GFP in a different bru1RNAi line, as well as in two bru1 mutant and deficiency fly lines (data not shown). Nonetheless, this does not exclude the possibility that Bru1 may be present below our detection limits and could facilitate sufficient translational repression.
By contrast, cup knockdown led to the accumulation of a strong Osk-GFP signal in the nurse cells, particularly within the nuclei and their periphery, as well as within the oocyte (, S4E, S4F). We validated the observed premature Osk-GFP expression by crossing a combination of four different cup mutant fly lines (i.e. cup1/15, Fig. S4G). In cupRNAi egg chambers, the Osk-GFP signal was detected as early as stage 1. The strongest Osk-GFP signal was surprisingly observed in stages 2–3, when Bru1 expression was still comparable to wt, confirming that Bru1 alone, without Cup, is not sufficient to maintain the translational repression of osk mRNA during these early stages (, S4E). Beginning at stage 5, Osk-GFP signal was drastically reduced in cupRNAi egg chambers, becoming undetectable by stage 7. Interestingly, these are the stages when Bru1-GFP aggregated in the nurse cell nuclei and no longer colocalized with osk mRNA in the cytoplasm (, arrows), implying that Bru1 plays a key role in activating osk mRNA translation.
To further test this idea, we postulated that if Bru1 plays a crucial role in activating osk mRNA translation, a double knockdown of cup and bru1 will significantly reduce any activation that Bru1 mediates in cupRNAi egg chambers. However, if Bru1 serves only to help repress translation, without a role in activating translation, the double knockdown would present premature Osk-GFP expression due to the simultaneously reduced Cup levels. Remarkably, we did not observe any premature Osk-GFP in these egg chambers (), even though osk mRNA was still detected, albeit at a significantly reduced level (Fig. S4E). To further confirm Bru1’s role in activating osk mRNA premature translation, we again exploited the differences in cupRNAi knockdown efficiency in neighbouring nurse cells. Bru1 formed large puncta in the nurse cell nuclei (, arrows) and Osk-GFP was undetectable when the levels of Cup and Bru1 signals were strongly reduced as compared to wt. However, we observed a strong premature Osk-GFP expression in nurse cells where both Cup and Bru1 were detectable but at very low levels (). This indicates that Cup must persist above a certain threshold level to maintain translational repression, and similarly there is a threshold level for Bru1 to facilitate translation. Additionally, Bru1 and Osk-GFP colocalized in the nurse cell cytoplasm in large puncta indicating possible translational hubs of osk mRNA via Bru1 (). We confirmed these results by detecting endogenous Osk in the presence or absence of the Osk-GFP (Figs S4H). While the Osk signal reported by the antibody was difficult to detect, we used an advanced image acquisition set up, optimized to resolve low signals.
Cup facilitates the association of osk mRNA with P-bodies and is necessary for proper P-body morphology
Knowing that osk mRNA localizes into P-bodies, where translationally repressed mRNAs are stored [Citation18,Citation40], we hypothesized that with reduced levels of Cup, osk mRNA would not associate with P-bodies, thus leading to osk’s premature and ectopic translation and degradation. To test this notion directly, we examined the recruitment of osk mRNA into P-bodies, using the P-body marker Me31B-GFP. First, we determined that Bru1, Cup, Me31B- GFP and osk mRNA all associate in the cytoplasm, forming large aggregates in wt egg chambers, indicating that they are components of the same condensate (Fig. S5A). As osk mRNA accumulates in a tight crescent at the oocyte’s posterior pole, it becomes poised for translation. During this time, osk mRNA signal highly exceeds the expression signal of Me31B and Cup, indicating a reorganization of the components of these condensates, with a likely dissociation of osk mRNA from P-bodies (Fig. S5B).
Next, we generated flies co-expressing the otu-Gal4 driver and endogenously tagged Me31B-GFP in combination with bru1RNAi or cupRNAi. In bru1RNAi egg chambers, we did not detect a change in the association of osk mRNA with Me31B-GFP, indicating that osk mRNA continues to localize into P-bodies, and it is translationally repressed when Bru1 levels were reduced (Figs S5C, S5D). However, in the cupRNAi background, though Me31B-GFP was reduced, a considerable amount of signal persisted throughout the egg chambers ( vs 5B, 5F vs 5 G). This was not surprising, as Cup has been shown to be partially important for maintaining Me31B levels [Citation39]. Me31B-GFP formed condensates in early stages of cupRNAi egg chambers, but the colocalization of osk mRNA with Me31B-GFP was decreased (49 ± 1% in wt to 43 ± 1% in cupRNAi) ( vs 5B, 5C), however the dissociation of osk mRNA from Me31B-GFP became more significant during mid-oogenesis (51 ± 1% in wt to 40 ± 1% in cupRNAi) ( vs 5 G, 5 H). The decreased percentage in their colocalization becomes even more significant when taking into account the already reduced levels of osk mRNA in cupRNAi egg chambers. If the association of osk mRNA with P-bodies were not mediated via Cup, these lower levels of osk mRNA would instead increase the percentage of colocalization with Me31B, as transcripts within P-bodies are protected from degradation. Our data indicates that Cup is necessary for osk mRNA’s association with P-bodies, implicating that when osk mRNA is not housed in P-bodies, translational repression and mRNA stability are compromised.
Figure 5. Cup facilitates the recruitment of osk mRNA into P-bodies and regulates the condensates’ physical state. (A, B, F, G) Representative images of osk smFISH experiments expressing Me31B-GFP in wt and cupRNAi backgrounds in early stage (A, B) and mid stage (F, G) egg chambers. Images are deconvolved XY max-intensity Z-projections of early stage: 26 (wt), 25 (cupRNAi), 25 (bru1RNAi) and mid stage: 31 (wt), 25 (cupRNAi) egg chambers, optical slices (0.3 µm each). (C, H) Colocalization quantification of osk mRNA with Me31B-GFP was performed in wt and cupRNAi backgrounds in early stage (**p = 0.0076; wt vs cupRNAi: n = 9 and n = 6) (C) and mid stage (****p < 0.0001; wt vs cupRNAi: n = 8 and n = 6) (H) egg chambers. (D, I) Volume analysis of Me31B-GFP condensates was performed in wt and cupRNAi backgrounds in early stage (****p < 0.0001; wt vs cupRNAi: n = 13 and n = 13) (D) and mid stage (****p < 0.0001; wt vs cupRNAi: n = 14 and n = 14) (I) egg chambers. (E, J) Sphericity analysis of Me31B-GFP condensates was performed in wt and cupRNAi backgrounds in early stage (**p = 0.0052; wt vs cupRNAi: n = 14 and n = 14) (E) and mid stage (****p < 0.0001; wt vs cupRNAi: n = 14 and n = 14) (J) egg chambers. Scale bars, 10 μm.
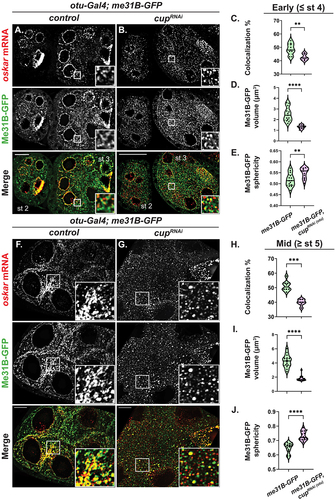
Previous work from multiple groups have shown that the physical properties of Me31B condensates correspond to the fate of mRNAs that are regulated in P-bodies in mature oocytes and in early embryos [Citation11,Citation16]. When P-bodies are in a dynamic state, characterized by a smaller and more spherical morphology, mRNAs are released from and/or fail to incorporate into P-bodies. Conversely, the more amorphous, arrested physical state, preceded by a short-lived liquid state, facilitates mRNA storage and the incorporation of mRNAs and proteins into P-bodies [Citation11,Citation16]. We wished to assess the role of Cup in modulating the physical state of P-bodies during oogenesis by analysing the size and sphericity of Me31B-GFP condensates. In cup knockdown, we found a significant decrease in P-body size (early: 2.51 ± 0.18 µm3 in wt to 1.32 ± 0.05 µm3 in cupRNAi; mid: 4.27 ± 0.24 µm3 in wt to 1.81 ± 0.10 µm3 in cupRNAi) (). More importantly, the P-body surface also changed significantly from an amorphous to a more spherical morphology (early: 0.52 ± 0.008 µm3 in wt to 0.55 ± 0.007 µm3 in cupRNAi; mid:0.65 ± 0.009 µm3 in wt to 0.73 ± 0.009 µm3 in cupRNAi) (). Taken together, these results indicate that Cup is important for modulating the physical state of P-bodies, which in turn facilitates proper storage of translationally repressed mRNAs.
Discussion
Our study demonstrates details of a complex feedback loop between two key maternal protein factors, Bru1 and Cup, in early oogenesis when location and timing of osk mRNA expression is critical for proper Drosophila melanogaster development. By employing cup knockdown flies, we showed that Bru1’s association with osk mRNA is compromised, an unexpected and important observation since an earlier study suggested that Bru1 binds without a binding partner at the BRE sites of osk 3’UTR [Citation8]. A more recent study, however, demonstrated that deletion of the Cup-binding (N-terminal) domain of Bru1 that left the RRM domains intact led to a largely diffuse Bru1 expression while reducing osk mRNP granule assembly [Citation11]. Extending the work on the interactions between Bru1 and Cup, we now suggest that both Bru1 binding and a Cup-stabilized interaction with osk mRNA contribute to the formation of a mature, translationally silent, osk mRNP. We speculate that for Bru1, acting through a binding partner may reflect a broad mechanism of action that remains to be elucidated, since repression by Bru1 of germ cell-less mRNA occurs in a BRE-independent manner [Citation41].
It was previously found that premature Osk expression occurred exclusively in the oocyte in egg chambers with deleted eIF4E-binding sites in Cup [Citation4]. We however observed robust premature Osk expression throughout early oogenesis (stage 2–4) in cup knockdown egg chambers, despite persistent Bru1 levels, precluding the notion that small amounts of Bru1 alone can maintain translational repression. Our observation indicates that Bru1 is insufficient for silencing osk mRNA translation, even though Bru1 and osk mRNA remained colocalized, albeit at a reduced level. In stark contrast, bru1 knockdown egg chambers did not express Osk prematurely. Cup levels decreased but remained sufficient to colocalize with osk mRNA and sustain its translationally repressed state, supporting a previous study where Cup was able to maintain translational repression in an RNA-binding protein-independent manner in the embryo [Citation14]. Overall, these results imply that the Bru1-Cup-eIF4E complex is the primary mechanism that represses osk’s translation after the mRNP is deposited into the oocyte, while Cup is the essential factor for osk’s translational repression in nurse cells.
Previous work indicated that Bru1 is only necessary for translation activation of osk mRNA at the posterior cortex of the oocyte [Citation2]. Our results from our mosaic egg-chamber analysis of double knockdown cupRNAibru1RNAi flies imply that Bru1’s translational role will occur throughout the egg chamber when Bru1 expression is at a threshold level and when Cup is not part of the osk mRNP. To explain these observations, we postulate that the N-terminal domain facilitates translation activation since Bose et al. did not detect Osk protein in N-terminal-deleted bru1 mutant egg chambers [Citation11]. As Cup protein normally binds Bru1 through this domain, future studies could explore whether other protein factors are also recruited to this domain to activate osk mRNA translation in the absence of Cup.
We can also report a previously undescribed phenotype in cupRNAi egg chambers: Bru1 forms large punctas in nurse-cell nuclei devoid of osk mRNA. We propose two possible mechanisms: (1) since Cup interacts with members of the nuclear pore complex [Citation42], Cup knockdowns affect export through the nuclear pore and generate increased Bru1 concentration within the nuclei which, in turn, leads to concentration-dependent aggregation, or (2) in the absence of Cup, Bru1 forms large aggregates from increased Bru1-Bru1 dimerization within the nucleus, an effect consistent with in vitro studies that reported Cup competition for Bru1 dimerization-binding sites [Citation2]. Therefore, it is possible that without Cup, Bru1 forms large aggregates due to increased Bru1-Bru1 association in the nucleus followed by a downstream alteration of its normal association with osk mRNP. The consequence of both mechanisms for nurse cell cytoplasm would be more widely diffused Bru1, locally decreased concentration, and diminished aggregation. We favour the second possibility since the N-terminal-deleted Bru1 protein mutants were also diffused in the cytoplasm [Citation11].
Our model that emerges from these data describes a reciprocal interaction between Cup and Bru1 to maintain functional levels and distribution in the egg chamber. Variations beyond threshold for this sensitive protein relationship is lethal for the developing fly, similar to other widespread auto- and/or cross-regulated protein combinations in cellular biology, for example, RNA-binding proteins such as ELAV in neurons and RBPS that regulate translation and RNA integrity [Citation43–45]. Deciphering how these CELF family member proteins regulate expression and function is crucial for the understanding of health and disease progression. Intriguingly, Cup’s role in Bru1’s expression extends beyond intracellular localization. We found not only that Cup regulates bru1 mRNA levels, but that Bru1 also regulates cup mRNA levels, likely through a feedback loop. We used the RBPmap web server to identify several potential candidates that could mediate mutual regulation [Citation45]. Analysis of bru1 mRNA shows that multiple proteins, including Bru1 itself, have putative-binding sites within the bru1 mRNA sequence, possibly for recruiting Cup. Consistent with our model of reciprocal interaction, we also found that cup mRNA contains numerous BRE sites, which have the potential to bind Bru1 directly. The association could result in the stabilization of cup mRNA, perhaps through Bru1 recruiting Cup itself. While this explanation is plausible, the Rangan group recently showed that Bru1 is necessary for translational repression of pgc mRNA [Citation46]. PGC directs global transcriptional silencing by inhibiting RNA polymerase II activity. Conceivably, the decreased levels of cup and osk mRNAs may be due to this more indirect action of Bru1. These two possible mechanisms of maintaining mRNA expression levels via Bru1 are not mutually exclusive and require further exploration.
Another intriguing question remaining is whether this mRNA feedback loop takes place in P-bodies. Recent work showed that the physical state of P-bodies, as well as their integrity, determines recruitment and release of mRNAs into and from P-bodies [Citation16]. Cup can interact with multiple key P-body factors such as Me31B and Tral. The absence of Cup during early oogenesis reduces the rate of association of osk mRNA with Me31B, and alters P-body morphology, as we demonstrated a shift into smaller sizes and more spherical shapes of P-bodies. We observed the highest premature expression of Osk protein during these early stages, leading us to propose future studies to determine whether bru1 and cup are stabilized in P-bodies as is the osk transcript.
The physical state of P-bodies in cup knockdowns continues to change as oogenesis progresses. P-bodies continue to shrink in volume and become more spherical. In this state, the majority of the osk mRNA is not associated with P-bodies. This smaller and more spherical morphology of Me31B condensates is reminiscent of P-body characteristics in the early embryo, a time when the mRNAs are released for translation. Other work has shown that Cup levels decrease drastically during early embryogenesis and lead to a change in Me31B’s role in the regulation of mRNAs. In effect, mRNA association with Me31B switches from mRNA storage to mRNA degradation during this developmental stage [Citation14]. Our findings confirm that osk mRNA is unstable as its association with P-bodies becomes compromised, which suggests that Cup is involved in the formation of a more amorphous, arrested state of P-bodies. With the loss of Cup, Me31B condensates become more spherical, resulting in osk mRNA degradation. However, we cannot rule out the possibility that as Cup is depleted, Me31B levels decrease to such an extent that it can no longer form proper condensates.
Our results, therefore, show that Cup is crucial for ensuring proper P-body morphology and, at the same time, shed new light on mechanisms of maternal mRNA storage, opening the possibility that the amorphous P-body state facilitates osk mRNA storage. Further investigation can help clarify whether the premature osk mRNA translation is due to the inability of mRNA to be recruited into P-bodies or due to its premature release from P-bodies, two non-mutually exclusive possibilities. The model we are advancing here has Bru1 and Cup serving reciprocal roles, like ‘yin and yang’ partners, that direct each other’s correct expression by maintaining sensitive protein threshold levels and promoting each other’s wild-type localization (). The Bru1-Cup complex is necessary to preserve a stable and translationally silent osk mRNA. Additionally, the protein complex plays a crucial function in the association of the osk mRNP with P-bodies, which results in osk’s translational repression.
Figure 6. Cup models P-body physical states and governs osk mRNA regulation during Drosophila melanogaster oogenesis. An intertwined mechanism of post-transcriptional gene regulation during oogenesis, in which a feedback mechanism between Bru1 and Cup maintains a sensitive threshold of each other’s gene expression and distribution. Bru1 and Cup form a complex that associates with osk mRNA in the cytoplasm. Cup then links osk mRNA to P-bodies, where it is maintained as a stable and translationally silent transcript. Upon loss of Cup, the physical state of P-bodies changes, osk mRNP no longer associates with P-bodies that leads to osk mRNA translation and ultimate degradation. Translation is facilitated by Bru1 that may occur by recruiting another Bru1-binding factor.
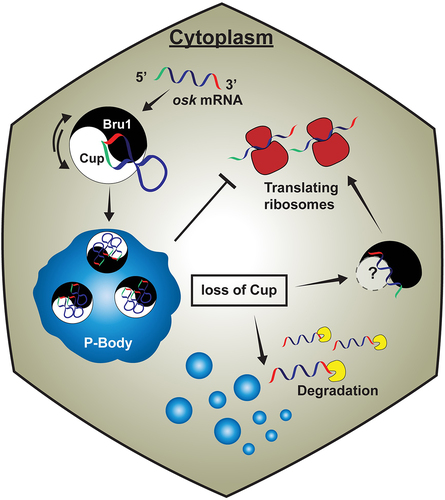
Such mechanisms of mRNA regulation are conserved beyond Drosophila oogenesis, for example in neuronal development, where both mRNA binding proteins and mRNAs stored in phase-separated condensates are necessary for proper memory formation ([Citation47], reviewed in [Citation48]).
Materials and methods
Fly husbandry
All fruit fly stocks were maintained on standard cornmeal agar food at 25°C. Prior to dissection, female flies were fed yeast paste in grape vials for 2–5 days. Fly stocks were obtained from Bloomington Drosophila Stock Center: Gal4 inducible TRiP lines: mCherry (BL #35785), cup (BL #35406), bru1 (BL #35394 and BL #54812), btz (BL #58353), eIF4E (BL #34096). Mutant Lines: cup15 (BL #29718) cup1 (BL #4978). Maternal Gal4 driver lines: BL #7063 (tub-alpha(V37)-Gal4) and BL #58424 (otu). Fluorescently tagged: Bru1-GFP (BL #60144) and Me31B-GFP (BL #51530). We acquired Cup-YFP (DGRC 115–161) from the Kyoto Drosophila `Stock Center. CRISPR Oskar-GFP fly line was a generous gift from Dr G. Gonsalvez (Augusta University). Experiments were carried out with the otu-Gal4 driver, unless otherwise indicated.
Single-molecule RNA FISH (smFISH) and combined smFISH-Immunofluorescence (IF)
smFISH and smFISH-IF were performed as previously described [Citation49]. Briefly, ovaries were dissected in Robb’s medium [Citation50], fixed in 4% PFA in 1X oocyte buffer for 10 min. After fixation, egg chambers were pre-hybridized in wash buffer (2X SSC, 10% formamide), incubated with the osk-CY5 probes (Supplemental_Methods_S1) overnight at 37°C, followed by washing with wash buffer and mounting with ProLongTM Diamond Antifade Mountant (Life Technologies) on a glass slide using a #1.5 cover glass. Combined smFISH-IF was carried out with the following modification: after fixation, the egg chambers were permeabilized for 2 h in 1% Triton X-100 in 2X SSC with 1% BSA followed by incubation with osk-CY5 probes for 4 h at 37°C, egg chambers were then incubated with primary antibodies overnight at room temperature in 0.3% Triton X-100, 0.2% BSA, 2X SSC. After three washes with 0.05% Triton X-100 in 2X SSC, the egg chambers were incubated with fluorescently labelled secondary antibodies [Alexa Fluor Plus 555, Alexa Fluor 405 (1:1,000; Life Technologies)] for 2 h at room temperature in 0.3% Triton X-100, 0.2% BSA, 2X SSC, and washed and mounted as previously described (Bayer et al., 2015). Nuclear membrane was detected with Wheat germ agglutinin (WGA) CF405S (1:100; Biotium) and actin was detected with Phalloidin DyLight554 (1:200; ThermoFisher). Primary antibodies used were rat anti-Cup (1:1,000) (kind gift from Dr Allan Spradling, Carnegie Institution for Science), mouse anti-Cup (1:1,000) (kind gift from Dr Akira Nakamura, Institute of Molecular Embryology and Genetics, Kumamoto University), rabbit anti-Bru1 (1:3,000) (kind gift from Dr Mary Lilly, National Institutes of Health National Institutes of Child Health & Development) rabbit anti-Osk (1:1000) (kind gift from Dr Paul Lasko, McGill University) and rabbit anti-GFP (1:1,000) (Millipore).
Live imaging
Whole ovaries were dissected from well fed females expressing either Bru1-GFP or Cup-YFP in a wild type or knocked down (RNAi) background, and individual egg chambers were separated in Halocarbon oil 700 (Sigma-Aldrich) on a #1.5 cover glass. Egg chambers were microinjected with a solution containing a cocktail of five osk-specific molecular beacons at a concentration of 200 ng/µl of each probe. Molecular beacons were designed, synthesized, labelled, and purified as we previously described (Supplemental_Methods_S2) [Citation27,Citation28]. Imaging was initiated within seconds after microinjection, and data acquisition was performed at room temperature on a LeicaDMI-4000B inverted microscope (Leica Microsystems, Buffalo Groove, IL) mounted on a TMC isolation platform (Technical Manufacturing Corporation, Peabody, MA), with a Yokogawa CSU10 spinning disc head and Hamamatsu C9100–13 ImagEM EMCCD camera. The microscopy set up includes diode lasers [491, 561, and 638 nm (Spectra Services, Ontario, NY)], and an Eppendorf Patchman- Femtojet microinjector (Eppendorf, Hauppauge, NY). The images were acquired as 16-bit data files, with 40×/1.25, 63×/1.4 or 100×/1.42 oil objectives (0.385, 0.24 and 0.143 µm/pixel, respectively), using Volocity acquisition software (Quorum Technologies). The second microscope set up was composed of a Yokogawa CSU22 spinning disc head and Hamamatsu ORCA-ER CCD camera, mounted on an inverted Nikon TE2000-U microscope with an 60×/1.49 oil objective. It was equipped with five Obis solid state lasers (405, 488, 514, 561, 640 nm) controlled by an Obis Scientific Remote. Acquisition software: Nikon NIS – Elements v 4.6. The third microscope set up was Leica TCS SP8 WLL. It was equipped with a White Light Laser (470-670 nm), a solid-state laser (405 nm), an acousto-optical tunable filter and an 63×/1.4 oil objective. Acquisition software: Leica LAS-X. Optical Z-stacks with a slice thickness ranging from 0.3 to 0.5 µm were acquired using a manual XY-stage with piezo-Z (PerkinElmer), or automated XYZ-piezo stage, respectively. For tracking analysis, 11 to 17 Z-slices of 0.3 to 0.5 µm were acquired every 30 s for at least 10 min, using the ‘High-quality’ camera mode.
Image processing, particle detection and tracking
Identical image acquisition, processing parameters and same stage egg chambers were compared with wt. Images were deconvolved using AutoQuant X (Media Cybernetics) with adaptive PSF (Blind) using the default recommended expert settings (10 iterations, 10 interval and 20 noise level). Images were processed with ImageJ and Icy softwares [Citation29,Citation51]. Particle detection, colocalization and tracking analyses for live egg chamber experiments were performed with Icy, using the ‘Spot detection’, ‘Colocalization’’ and ‘Spot Tracking’ blocks. For live cell images acquired with the 63× objective, deconvolution and background subtraction were performed, and then spots were detected using the following parameters: scale 2 and 70–110 sensitivity; object-based bright spot detection and size filtering of minimum 4 pixels. Colocalization was determined as a distance of 4 pixels or less from the middle of the 2 particles. Colocalization of osk mRNA with either Bru1-GFP or Cup-YFP was measured using an Icy protocol with “Spot detection and Colocalizer’’. Only colocalized particles were used to perform tracking analysis. Tracking parameters were estimated for diffusive and directed motion. Fixed images acquired with the 40× objective were not deconvolved. For fixed images acquired with the 63× or 100× objectives, colocalization analysis was carried out after deconvolution without any further processing using Icy, at scale 1 and sensitivity 40 (osk mRNA), 60 (Cup-YFP), 80 (Bru1-GFP), 70 (Me31B-GFP), with minimum size filtering of 3 pixels. All image insets are 2× magnification of the corresponding region indicated with a white rectangle using the Zoom in Images and Stacks macro by Gilles Carpentier available in ImageJ. Pairwise stitching was used in a subset of images in ImageJ [Citation52]. Imaris 9.7 image analysis software surface detection module was used to detect Me31B-GFP signal to calculate the volume and the sphericity of each object detected. Thresholding and sensitivity were carefully determined for each image, objects smaller than 0.15 µm3 for early and 0.2 µm3 for mid oogenesis were removed from the analysis. Statistical analysis was carried out using Prism 8 software (GraphPad) using Mann-Whitney test, aka Wilcoxon rank sum test, to calculate the p value, except where otherwise indicated. On the colocalization and mRNA quantification graphs, each data point is represented along with mean ± SEM. Normalized fluorescence intensity calculation for line profiles: (i – imin)/(imax- imin). Line profiles were performed with a linewidth of 8 µm, 26 µm, 10 µm, and 8 µm in , S1D and S2C, respectively.
Western blotting analysis
Ovaries from young females fed on yeast paste for 3–5 days were dissected in Robb’s medium. The samples were lysed with CytobusterTM Protein Extraction Reagent (Millipore) supplemented with HALT protease inhibitor cocktail. Antibodies used were anti-GFP (1:5,000) (Millipore), anti-Bru1 (1:5,000) (kind gift from Dr Mary Lilly, National Institutes of Health National Institutes of Child Health & Development) and anti-α Tubulin 12G10 (1:15,000) (DSHB Hybridoma Product 12G10 anti-alpha-tubulin, the monoclonal antibody developed by Frankel, J./Nelsen, E.M. was obtained from the Developmental Studies Hybridoma Bank, created by the NICHD of the NIH and maintained at The University of Iowa, Department of Biology, Iowa City, IA 52,242). HRP-conjugated secondary antibodies were used at 1:5,000 anti-rabbit (Cell Signaling) or anti-mouse (Fisher Scientific). Protein band quantification analysis was performed by using ImageJ [Citation51].
RNA isolation and RT-Qpcr
Whole ovaries were dissected in Robb’s medium, washed with 1X PBS and kept on ice. As control for the RNAi machinery, we drove RNAi for mCherry, a non-endogenous gene in the fly genome. Total RNA was isolated using TRIzol (ThermoFisher) according to the manufacturer’s instructions. RT reactions using 2.25 µg total RNA, Superscript III (Life Technologies) and dT18 oligomers were used to synthesize cDNA. No RT enzyme reactions were used as negative controls. Primers were designed using DRSC FlyPrimerBank for Drosophila (Primer sequences: Supplemental_Methods_S3). Primers were acquired from Integrated DNA Technologies. qPCR was performed on white 384-well plates (E&K Scientific, Santa Clara, CA) using Roche Lightcycler 480 (Roche Molecular Systems, Inc.). Each reaction contained 1 µL of cDNA from the RT reaction, 2 µL of primer solution containing 10 µM forward and reverse primers, 5 µL SYBR Green I Master mix (5 mL: Roche Diagnostics, Indianapolis, IN) and 2 µL of distilled and deionized H2O. Plates were spun down to eliminate air bubbles. Reactions were carried out as follows: 95°C denaturation for 5 min, followed by 37 cycles of 95°C for 20 sec, 58°C for 15 sec. Statistical analyses were carried out using Prism 8 software using unpaired t test to calculate the p value.
Supplemental Material
Download Zip (7.7 MB)Acknowledgments
We thank Dr A. Spradling (Carnegie Institution for Science), Dr A. Nakamura (Riken Center for Developmental Biology), Dr P. Lasko (McGill University) and Dr M. Lilly (NIH) for their kind gifts of antibodies. We extend a special thanks to Dr G.B. Gonsalvez (Augusta University) for allowing us to use, and be first to publish, the CRISPR osk-GFP fly strain made in his laboratory. We thank the BDSC Indiana and Kyoto DGRC for providing the fly stocks for our study, and the TRiP at Harvard Medical School (NIH/NIGMS R01-GM084947) for providing the transgenic RNAi fly stocks. We thank E. Bagaeva for assistance with fly husbandry and maintenance of stocks, and Dr S.A.E. Marras (Public Health Research Institute Center, Rutgers University) for the synthesis, labeling and purification of osk molecular beacons, and labeling of the osk smFISH probes. We thank the Bioimaging Facility at Hunter College for access to the Leica TCS SP8, Nikon spinning disc microscope, Autoquant and Imaris 9.7 software used for image analysis. We thank Dr P. Feinstein for allowing us to use the Roche Lightcycler instrument. We are grateful to Dr J.M. McLaughlin and O.S. Omar for their critical comments and especially to Dr R. Persell for his editorial advice on the manuscript.
Disclosure statement
No potential conflict of interest was reported by the author(s).
Data availability statement
The authors confirm that the data supporting the findings of this study are available within the article and its supplementary material.
Supplementary material
Supplemental data for this article can be accessed online at https://doi.org/10.1080/15476286.2023.2242650
Additional information
Funding
References
- Lehmann R, Nusslein-Volhard C. Abdominal segmentation, pole cell formation, and embryonic polarity require the localized activity of oskar, a maternal gene in Drosophila. Cell. 1986;47(1):141–152. doi: 10.1016/0092-8674(86)90375-2
- Kim G, Pai C-I, Sato K, et al. Region-specific activation of oskar mRNA translation by inhibition of Bruno-mediated repression. PLoS Genet. 2015;11(2):e1004992. doi: 10.1371/journal.pgen.1004992
- Kim-Ha J, Kerr K, Macdonald PM. Translational regulation of oskar mRNA by Bruno, an ovarian RNA-binding protein, is essential. Cell. 1995;81(3):403–412. doi: 10.1016/0092-8674(95)90393-3
- Nakamura A, Sato K, Hanyu-Nakamura K. Drosophila cup is an eIF4E binding protein that associates with Bruno and regulates oskar mRNA translation in oogenesis. Dev Cell. 2004;6(1):69–78. doi: 10.1016/S1534-5807(03)00400-3
- Wilhelm JE, Hilton M, Amos Q, et al. Cup is an eIF4E binding protein required for both the translational repression of oskar and the recruitment of Barentsz. J Cell Bio. 2003;163(6):1197–1204. doi: 10.1083/jcb.200309088
- Snee M, Benz D, Jen J, et al. Two distinct domains of Bruno bind specifically to the oskar mRNA. RNA Biol. 2008;5(1):1–9. doi: 10.4161/rna.5.1.5735
- Reveal B, Yan N, Snee MJ, et al. Bres mediate both repression and activation of oskar mRNA translation and act in trans. Dev Cell. 2010;18(3):496–502. doi: 10.1016/j.devcel.2009.12.021
- Reveal B, Garcia C, Ellington A, et al. Multiple RNA binding domains of Bruno confer recognition of diverse binding sites for translational repression. RNA Biol. 2011;8(6):1047–1060. doi: 10.4161/rna.8.6.17542
- Snee MJ, Harrison D, Yan N, et al . A late phase of Oskar accumulation is crucial for posterior patterning of the Drosophila embryo, and is blocked by ectopic expression of Bruno. Differentiation. Res In Bio Divers. 2007;75(3):246–255. doi: 10.1111/j.1432-0436.2006.00136.x
- Chekulaeva M, Hentze MW, Ephrussi A. Bruno acts as a dual repressor of oskar translation, promoting mRNA oligomerization and formation of silencing particles. Cell. 2006;124(3):521–533. doi: 10.1016/j.cell.2006.01.031
- Bose M, Lampe M, Mahamid J, et al. Liquid-to-solid phase transition of oskar ribonucleoprotein granules is essential for their function in Drosophila embryonic development. Cell. 2022;185(8):1308–1324.e23. doi: 10.1016/j.cell.2022.02.022
- Kinkelin K, Veith K, Grünwald M, et al. Crystal structure of a minimal eIf4E-Cup complex reveals a general mechanism of eIF4E regulation in translational repression. RNA. 2012;18(9):1624–1634. doi: 10.1261/rna.033639.112
- Igreja C, Izaurralde E. CUP promotes deadenylation and inhibits decapping of mRNA targets. Genes Dev. 2011;25(18):1955–1967. doi: 10.1101/gad.17136311
- Wang M, Ly M, Lugowski A, et al. ME31B globally represses maternal mRNAs by two distinct mechanisms during the Drosophila maternal-to-zygotic transition. Elife. 2017;6. doi: 10.7554/eLife.27891
- Standart N, Weil D. P-Bodies: Cytosolic Droplets for Coordinated mRNA Storage. Trends Genet. 2018;34(8):612–626. doi: 10.1016/j.tig.2018.05.005
- Sankaranarayanan M, Emenecker RJ, Wilby EL, et al. Adaptable P body physical states differentially regulate bicoid mRNA storage during early Drosophila development. Dev Cell. 2021;56(20):2886–2901.e6. doi: 10.1016/j.devcel.2021.09.021
- Luo Y, Na Z, Slavoff SA. P-Bodies: composition, properties, and functions. Biochemistry. 2018;57(17):2424–2431. doi: 10.1021/acs.biochem.7b01162
- Nakamura A, Amikura R, Hanyu K, et al . Me31b silences translation of oocyte-localizing RNAs through the formation of cytoplasmic RNP complex during Drosophila oogenesis. Development. 2001;128(17):3233–3242. doi: 10.1242/dev.128.17.3233
- Weil TT, Parton RM, Herpers B, et al. Drosophila patterning is established by differential association of mRNAs with P bodies. Nat Cell Biol. 2012;14(12):1305–1313. doi: 10.1038/ncb2627
- McLaughlin JM, Bratu DP. Drosophila melanogaster Oogenesis: An Overview. Methods Mol Biol. 2015;1328:1–20 doi:10.1007/978-1-4939-2851-4_1
- Ephrussi A, Dickinson LK, Lehmann R. Oskar organizes the germ plasm and directs localization of the posterior determinant nanos. Cell. 1991;66(1):37–50. doi: 10.1016/0092-8674(91)90137-N
- Ephrussi A, Lehmann R. Induction of germ cell formation by oskar. Nature. 1992;358(6385):387–392. doi: 10.1038/358387a0
- Kim-Ha J, Smith JL, Macdonald PM. oskar mRNA is localized to the posterior pole of the Drosophila oocyte. Cell. 1991;66(1):23–35. doi:10.1016/0092-8674(91)90136-m
- Kanke M, Jambor H, Reich J, et al. Oskar RNA plays multiple noncoding roles to support oogenesis and maintain integrity of the germline/soma distinction. RNA. 2015;21(6):1096–1109. doi: 10.1261/rna.048298.114
- Castagnetti S, Hentze MW, Ephrussi A, et al. Control of oskar mRNA translation by Bruno in a novel cell-free system from Drosophila ovaries. Development. 2000;127(5):1063–1068. doi: 10.1242/dev.127.5.1063
- Nagarkar-Jaiswal S, DeLuca SZ Lee PT, et al. A genetic toolkit for tagging intronic MiMIC containing genes. Elife. 2015;4. doi: 10.7554/eLife.08469
- Bratu DP, Cha B-J, Mhlanga MM, et al. Visualizing the distribution and transport of mRNAs in living cells. Proc Natl Acad Sci U S A. 2003;100(23):13308–13313. doi: 10.1073/pnas.2233244100
- Bayer LV, Omar OS, Bratu DP, et al. PinMol: Python application for designing molecular beacons for live cell imaging of endogenous mRNAs. RNA. 2019;25(3):305–318. doi: 10.1261/rna.069542.118
- de Chaumont F, Dallongeville S, Chenouard N, et al. Icy: an open bioimage informatics platform for extended reproducible research. Nat Methods. 2012;9(7):690–696. doi: 10.1038/nmeth.2075
- Keyes LN, Spradling AC. The Drosophila gene fs(2)cup interacts with otu to define a cytoplasmic pathway required for the structure and function of germ-line chromosomes. Development. 1997;124(7):1419–1431. doi: 10.1242/dev.124.7.1419
- Lowe N, Rees JS, Roote J, et al. Analysis of the expression patterns, subcellular localisations and interaction partners of Drosophila proteins using a pigP protein trap library. Development. 2014;141(20):3994–4005. doi: 10.1242/dev.111054
- Webster PJ, Liang L, Berg CA, et al. Translational repressor bruno plays multiple roles in development and is widely conserved. Genes Dev. 1997;11(19):2510–2521. doi: 10.1101/gad.11.19.2510
- Smith J, Calidas D, Schmidt H, et al. Spatial patterning of P granules by RNA-induced phase separation of the intrinsically-disordered protein MEG-3. Elife. 2016;5. doi: 10.7554/eLife.21337
- Pekovic F, Rammelt C, KubíkováJ, et al . RNA binding proteins Smaug and Cup induce CCR4-NOT-dependent deadenylation of the nanos mRNA in a reconstituted system. Nucleic acids research. 2023;51(8):3950–70. doi:10.1093/nar/gkad159
- Clouse KN, Ferguson SB, Schupbach T. Squid, cup, and PABP55B function together to regulate gurken translation in Drosophila. Dev Biol. 2008;313(2):713–724. doi: 10.1016/j.ydbio.2007.11.008
- Piccioni F, Zappavigna V, Verrotti AC. Translational regulation during oogenesis and early development: the cap-poly(A) tail relationship. C R Biol. 2005;328(10–11):863–881. doi: 10.1016/j.crvi.2005.05.006
- Piccioni F, Ottone C, Brescia P, et al. The translational repressor cup associates with the adaptor protein miranda and the mRNA carrier Staufen at multiple time-points during Drosophila oogenesis. Gene. 2009;428(1–2):47–52. doi: 10.1016/j.gene.2008.09.019
- Verrotti AC, Wharton RP. Nanos interacts with cup in the female germline of Drosophila. Development. 2000;127(23):5225–5232. doi: 10.1242/dev.127.23.5225
- Broyer RM, Monfort E, Wilhelm JE. Cup regulates oskar mRNA stability during oogenesis. Dev Biol. 2017;421(1):77–85. doi: 10.1016/j.ydbio.2016.06.040
- Fan S-J, Marchand V, Ephrussi A, et al. Drosophila Ge-1 promotes P body formation and oskar mRNA localization. PLoS One. 2011;6(5):e20612–e20612. doi: 10.1371/journal.pone.0020612
- Moore J, Han H, Lasko P. Bruno negatively regulates germ cell-less expression in a BRE-independent manner. Mech Dev. 2009;126(7):503–516. doi: 10.1016/j.mod.2009.04.002
- Grimaldi MR, Cozzolino L, Malva C, et al. Nup154 genetically interacts with cup and plays a cell-type-specific function during Drosophila melanogaster egg-chamber development. Genetics. 2007;175(4):1751–1759. doi: 10.1534/genetics.106.062844
- Borgeson CD, Samson ML. Shared RNA-binding sites for interacting members of the Drosophila ELAV family of neuronal proteins. Nucleic Acids Res. 2005;33(19):6372–6383. doi: 10.1093/nar/gki942
- Pullmann R, Kim HH, Abdelmohsen K, et al. Analysis of turnover and translation regulatory RNA-binding protein expression through binding to cognate mRNAs. Mol Cell Biol. 2007;27(18):6265–6278. doi: 10.1128/MCB.00500-07
- Samson ML. Evidence for 3’ untranslated region-dependent autoregulation of the Drosophila gene encoding the neuronal nuclear RNA-binding protein ELAV. Genetics. 1998;150(2):723–733. doi: 10.1093/genetics/150.2.723
- Flora P, Wong-Deyrup SW, Martin ET, et al. Sequential Regulation of Maternal mRNAs through a Conserved cis-Acting Element in Their 3’ UTRs. Cell Rep. 2018;25(13):3828–3843.e9. doi: 10.1016/j.celrep.2018.12.007
- Tsang B, Arsenault, J, Vernon RM, et al. Phosphoregulated FMRP phase separation models activity-dependent translation through bidirectional control of mRNA granule formation. Proc Natl Acad Sci USA. 2019;116(10):4218–4227. doi: 10.1073/pnas.1814385116
- Arkov AL. Looking at the pretty “phase” of membraneless organelles: a view from Drosophila glia. Front Cell Dev Biol. 2022;10:801953. doi: 10.3389/fcell.2022.801953
- Bayer LV, Batish M, Formel SK, et al. Single-molecule RNA in situ hybridization (smFISH) and Immunofluorescence (IF) in the Drosophila Egg Chamber. Methods Mol Biol. 2015;1328:125–136.
- Recipe, modified Robb’s medium. Cold Spring Harb Protoc. 2011;2011(8):12483. doi: 10.1101/pdb.rec12483
- Schindelin J, Arganda-Carreras I, Frise E, et al. Fiji: an open-source platform for biological-image analysis. Nat Methods. 2012;9(7):676–682. doi: 10.1038/nmeth.2019
- Preibisch S, Saalfeld S, Tomancak P. Globally optimal stitching of tiled 3D microscopic image acquisitions. Bioinformatics. 2009;25(11):1463–1465. doi: 10.1093/bioinformatics/btp184