ABSTRACT
Ribosomal RNA (rRNA) processing and maturation are fundamentally important for ribosome biogenesis, but the mechanisms in archaea, the third form of life, remains largely elusive. This study aimed to investigate the rRNA maturation process in Methanococcus maripaludis, a representative archaeon lacking known 3′-5′ exonucleases. Through cleavage site identification and enzymatic assays, the splicing endonuclease EndA was determined to process the bulge-helix-bulge (BHB) motifs in 16S and 23S rRNA precursors. After splicing, the circular processing intermediates were formed and this was confirmed by quantitative RT-PCR and Northern blot. Ribonuclease assay revealed a specific cleavage at a 10-nt A/U-rich motif at the mature 5′ end of pre-16S rRNA, which linearized circular pre-16S rRNA intermediate. Further 3′-RACE and ribonuclease assays determined that the endonuclease Nob1 cleaved the 3′ extension of pre-16S rRNA, and so generated the mature 3′ end. Circularized RT-PCR (cRT-PCR) and 5′-RACE identified two cleavage sites near helix 1 at the 5′ end of 23S rRNA, indicating that an RNA structure-based endonucleolytic processing linearized the circular pre-23S rRNA intermediate. In the maturation of pre-5S rRNA, multiple endonucleolytic processing sites were determined at the 10-nt A/U-rich motif in the leader and trailer sequence. This study demonstrates that endonucleolytic processing, particularly at the 10-nt A/U-rich motifs play an essential role in the pre-rRNA maturation of M. maripaludis, indicating diverse pathways of rRNA maturation in archaeal species.
Introduction
The ribosomal RNA (rRNA) genes in all the three domains of life are commonly organized as operons and are co-transcribed as polycistronic precursors. Therefore, subsequent processing is necessary to generate the mature 16S, 23S and 5S rRNAs in bacteria and archaea, as well as 18S, 28S, 5.8S rRNAs in eukaryotes [Citation1–4]. Pre-rRNA maturation is a crucial process in ribosome biogenesis for ensuring assembly of mature rRNA species and specific sets of ribosomal proteins so functional ribosomes [Citation5–7]. Incorrect or disrupted rRNA maturation can lead to defective ribosomes, and so to impair growth and even viability of organisms [Citation5,Citation8]. Extensive researches have been conducted on the processing pathways and the ribonucleases being involved in the rRNA maturation processes in several bacteria, eukaryotes and a few archaeal species. In bacteria such as Escherichia coli and Bacillus subtilis, endonuclease RNase III recognizes double-stranded RNA structures formed via base pairing between 5′ leader and 3′ trailer sequences of the 16S and 23S rRNA precursors, and facilitates an efficient separation of the rRNA species [Citation9,Citation10]. Similar double-stranded structures flanking the pre-18S and pre-25/28S rRNAs have been observed in eukaryotes, providing important structural determinants for the initial processing steps of rRNA maturation [Citation11]. In archaea, the 5′ and 3′ flanking sequences of 16S and/or 23S rRNA can form double-stranded RNA structures as well. These structures frequently include a bulge-helix-bulge (BHB) motif, which is prevalent in archaeal phyla like the TACK superphylum and Euryarchaeota except in Thermoplasmata class [Citation12,Citation13]. It determined that the splicing endonuclease EndA and RNA ligase RtcB are involved in the step-wise endonucleolytic processing at the BHB motif and the subsequent circularization of 16S and/or 23S rRNA [Citation14–16], a distinctive processing mechanism of archaea different from that of the bacterial and eukaryotic rRNA processing. The circular intermediates need to undergo additional endonucleolytic cleavages to be linearized for further maturation. However, the archaeal endonucleases responsible for linearizing the circular pre-rRNA intermediates are unknown. Moreover, the complete pre-rRNA maturation pathways in most archaeal species remain elusive, although several processing steps, such as cleavage of BHB motif, have been elucidated in some halophilic and thermophilic euryarchaea (e.g. Halobacterium, Thermoplasma, Archaeoglobus) and crenarchaea (e.g. Saccharolobus, Sulfolobus), and recently in a methanogenic archaeon Methanolobus psychrophilus [Citation14,Citation16–21]. A more recent study reported nanopore-based RNA sequencing to detect the rRNA maturation process in three archaea, Haloferax volcanii and Pyrococcus furiosus from Euryarchaeota and Sulfolobus acidocaldarius from Crenarchaeota [Citation22]. This not only deciphers the varied pre-rRNA maturation pathways but also exposes the conserved and different maturation steps occurring in archaea.
In recent years, several archaeal endonucleases have been implicated in the pre-rRNA maturation. RNase P and RNase Z specifically remove the 5′ leader and 3′ trailer sequences of tRNA precursors (pre-tRNAs), respectively [Citation23–25]. The tRNA and rRNA genes are frequently organized as a same operon, thus processing of pre-tRNAs also releases the adjacent rRNAs. The splicing endonuclease EndA has been identified to cleave at the BHB motif, a motif is also found in the intron-containing tRNA, rRNA and even some mRNA molecules in H. volcanii [Citation26,Citation27]. More recently, the β-CASP family protein aCPSF1 specifically cleaving at the uridine-rich RNA 3′-end has been identified as a general transcriptional termination factor in archaea [Citation28–30]. However, whether aCPSF1 functions in rRNA processing is yet to be determined. The PIN domain protein Nob1 has been reported to yield the mature 3′ end of 16S rRNA in Pyrococcus horikoshii and to linearize the circular pre-16S rRNA of M. psychrophilus in vitro [Citation16,Citation31]. Nevertheless, the exact targets of Nob1 in vivo have not been fully elucidated.
Following the endonucleolytic cleavages that release the pre-16S, pre-23S, and pre-5S rRNAs intermediates from the polycistronic precursor, exonucleolytic digestion by either 5′-3′ or 3′-5′ exonucleases is required to trim the excess 5′ leader and 3′ trailer sequences. 3′-5′ exonucleolytic and 5′-3′ exonucleolytic processing have been identified in Gram-negative bacteria such as enterobacteria and Gram-positive bacteria like Firmicutes, respectively [Citation32–34]. In archaea, a series of endo- and exonucleolytic events are found in the maturation of pre-16S, pre-23S, and pre-5S rRNA intermediates as well. Such as 5′-3′ and 3′-5′ exonucleolytic activities have been proposed to be responsible for trimming the 5′ and 3′ extensions of linearized pre-16S and pre-23S rRNA intermediates, as well as processing the pre-5S rRNA extensions at two ends in a methanogenic archaeon M. psychrophilus [Citation16]. However, the corresponding exonucleases involved in have not been demonstrated [Citation35].
This work investigated the pre-rRNA processing pathway in Methanococcus maripaludis, an archaeon characterized by the absence of the well-known 3′-5′ exonuclease homologs [Citation36]. A combination of processing sites mapping, ribonuclease assays, and molecular experiments revealed the comprehensive pathway of pre-rRNA processing, highlighting the critical role of endonucleolytic processing.
Results
Mapping the pre-rRNA processing sites in M. maripaludis
M. maripaludis encodes three rRNA gene operons, rrnA–C, as well as a separate 5S rRNA gene (). The rrnA and rrnB operons consist of 16S, 23S and 5S rRNA genes, while the rrnC operon include additional genes encoding tRNAAla2 and RNase P RNA (rnpB). Moreover, a fourth 5S rRNA gene is located within an operon comprising seven tRNA genes (). To identify the transcription start sites (TSSs) of the rrn operons, we analysed the genome-wide TSS map of M. maripaludis obtained by differential RNA-seq (dRNA-seq) [Citation37]. Interestingly, an identical 149 nucleotide sequence within the 5′ external transcribed spacers (ETSs) was found in the three rrn operons (Fig. S1). Additionally, stem structures containing BHB motifs by base pairing of the 5′ leader and 3′ trailer sequences were present in pre-16S and 23S rRNAs (), but differently an extra nucleotide incorporated in the pre-16S rRNA BHB stem.
Figure 1. The rrn operons and processing sites of pre-rRNA in M. maripaludis. (a) The organization of rrn operons encoded in M. maripaludis. Three rrn operons (rrnA, rrnB and rrnC) comprise 16S, 23S and 5S rRNAs, and a separate 5S rRNA gene is embedded in a tRNA operon. (b) Processing sites identified through dRNA-seq and Term-seq. The mature 5′ and 3′ ends of specific rRNAs are indicated by cyan and purple letters, respectively. Processing sites cleaved by putative ribonucleases are indicated by arrows of different colours. Processing sites with a featured 10-nt A/U-rich motif are marked by magenta arrows. (c) A set of processing sites with a unique 10-nt A/U-rich motif found in pre-rRNA. The motif was generated based on the alignment of the nine mapped cleavage sites at the indicated position, such as site ①: 16S 5′ −145, which indicates the position 145 nt upstream the mature 5′ end of 16S rRNA body, and site ③: 16S 5′ +1, indicating the mature 5′ end of 16S rRNA. (d) The cleavage of the BHB motif of 16S and 23S rRNA by EndA. The schematic represents the structure of RNA substrates and expected cleavage products (left panel), as detected by the denaturing urea-PAGE (right panel).
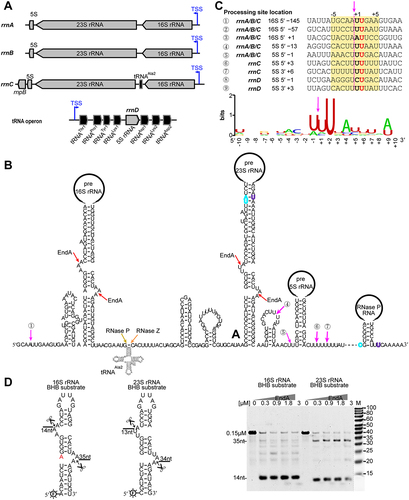
The processing sites within rRNA operons were further identified using the genome-wide mapping data of dRNA-seq and Term-seq in M. maripaludis [Citation28,Citation37], as depicted in . RNase P and RNase Z were proposed to cleave at the 5′ and 3′ end of tRNAAla to separate from the rrnC precursor [Citation23,Citation25], while the splicing endonuclease EndA could cleave at the BHB motif. Abundant processing sites at a consensus sequence ‘HDNNW↓HUDHH’ was identified in the pre-rRNAs of M. maripaludis (), by three sites within the 5′ ETS of 16S rRNA (sites ① ② ③) and another six found within the 5′ leader (sites ④ ⑤ ⑧) and 3′ trailer (sites ⑥ ⑦ ⑨) of 5S rRNA. A similar 10-nt motif sequence of ‘UNMND↓NUNAY’ was prevalently present around the processing sites of mRNAs and rRNAs in M. psychrophilus [Citation16,Citation38]. The processing sites within rrnA and rrnB operons are identical to that of rrnC operon (data not shown).
Circular processing intermediates of the pre-16S and pre-23S rRNA
Referenced to that the splicing endonuclease EndA specifically cleaves at BHB motif in the pre-16S and pre-23S rRNAs [Citation13,Citation16], we investigated the cleavage activity of M. maripaludis EndA (MmpEndA). Small RNAs containing the identified BHB motifs and partial processing stem sequences of M. maripaludis pre-16S and pre-23S rRNA were synthesized. The addition of purified recombinant MmpEndA from E. coli resulted in the generation of 14 nt and 35 nt, and 13 nt and 34 nt fragments from the synthesized 16S BHB and 23S BHB substrates, respectively (). This confirms the splicing endonuclease activity of the M. maripaludis EndA and its role to separate pre-16S and pre-23S rRNAs from the polycistronic transcript.
Following EndA cleavage at BHB motifs and subsequent ligation of the cleaved products by RNA ligase RtcB, circular rRNA molecules could be formed (Fig. S2). To detect the circularized intermediates of pre-16S and pre-23S rRNAs, RT-PCR was performed using divergent primers in RNA ligation omitted reactions (, left panel). Sequencing of the PCR products confirmed the self-ligation by the downstream 3′- and upstream 5′-splice sites of the BHB motifs (, right panel). Northern blot specifically probing the self-ligated sites of pre-16S and 23S rRNAs further validated the presence of circular pre-16S and pre-23S processing intermediates (). Quantitative RT-PCR assay determined that the circular processing intermediates account for 4.1% and 1.8% of the total 16S and 23S rRNAs, respectively, during the early-exponential growth phase; however, these proportions declined as the growth phase advanced (). These indicate that circularization of pre-16S and pre-23S rRNA occurs in the active cells of M. maripaludis.
Figure 2. Determination of the presence and percentages of circular pre-rRNA intermediates. (a) Validation of circular pre-rRNA intermediates through RT-PCR. The PCR primers are shown in the schematic of Fig. S2. The PCR products (red arrows) were amplified from cDNA templates that are reversed transcribed with primers RT from total RNA with (+) or without (−) T4 RNA ligase pretreatment. The presence of circular intermediates is confirmed by the amplification of PCR products. DNA sequencing showed the junction of 3′ splice site and 5′ splice site. (b) Circular pre-rRNA intermediates detected by Northern blot. Probes used for hybridizing the circular and mature rRNAs are indicated in Fig. S2. (c) qRT-PCR determined the percentages of circular pre-rRNA intermediates in the total RNA pool at the early, middle, late and stationary cultures. Three independent measurements were averaged, and standard deviations are shown.
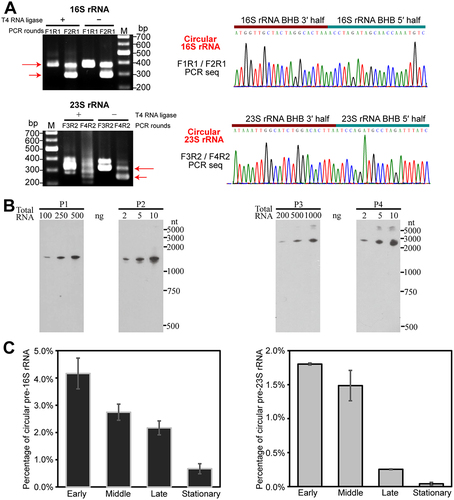
Endonucleolytic processing at a 10-nt A/U-rich motif at the 5′ end could linearize the circular pre-16S rRNA
To investigate the linearization pathway of the circular pre-16S rRNA in M. maripaludis, we extensively analysed the potential processing within the 120-nt spacer region. Primer extension was performed to determine the 5′ end processing sites of 16S rRNA, which validated the TSS of the rrn operon and the mature 5′ end (+1 nt) of 16S rRNA (Fig. S3) as determined by dRNA-seq. Next, 3′-RACE assay identified two processing sites at 58-nt and 1-nt upstream of the mature 5′ end of 16S rRNA, respectively (), and the flanking sequences contain a A/U-rich motif at single-stranded RNA regions (). This suggests that these endonucleolytic cleavages linearize the circular pre-16S rRNA, but the endonucleases are unknown. To test the cleavage occurring at the 10-nt A/U-rich motif, we synthesized a stem-loop RNA with a 16-nt loop containing the mature 5′ end sequence of 16S rRNA (−8 to + 8 nt) and a 9-GC stem structure (). Addition of cell-free extract (CFE) of M. maripaludis to the synthesized RNA substrate, expected cleaved product of 17-nt length was observed, and its production and substrate elimination were proportional to added CFE amount. This indicates an unknown endonuclease cleaves at the 5′ end 10-nt A/U-rich motif to linearize the circular intermediate of pre-16S rRNA. Furthermore, 5′-RACE captured the mature 5′ end (+1 site) of 16S rRNA (), but not the − 57 site. Improved capturing efficiency at site + 1 indicates that site + 1 could be the linearization site of the circular intermediate, while site − 57 could function as the processing site shortening the 3′ extension of pre-16S rRNA.
Figure 3. Linearization of circular pre-16S rRNA at the mature 5′ end. (a) 3′-RACE determined the 3′ end of linearized pre-16S rRNA intermediates. The schematic shows the circular pre-16S rRNA intermediate with two endonucleolytic processing sites indicated by magenta arrows (left panel). DNA sequencing results of 3′-RACE show the detected 3′ ends of pre-16S rRNA after linearization (right panel). (b) In vitro cleavage of the 10-nt A/U-rich motif at the mature 5′ end of 16S rRNA using cell-free extract (CFE) of M. maripaludis. The RNA substrate of SL35 comprising a 16-nt loop embedded with the 16S rRNA 5′-end sequence (flanking site ③) and a 9-GC base pairs stem, was used to assay the activity of the proposed endonuclease. RNA ladders with indicated length are shown on the left. The expected RNA product (17 nt in length) is indicated by the magenta arrow in the right panel. (c) The mature 5′ end of 16S rRNA determined by 5′-RACE. The upper panel illustrates the 5′-RACE procedure, and two rounds of PCR products (cyan arrows) were analysed on agarose gel (upper panel) and by DNA sequencing (lower panel).
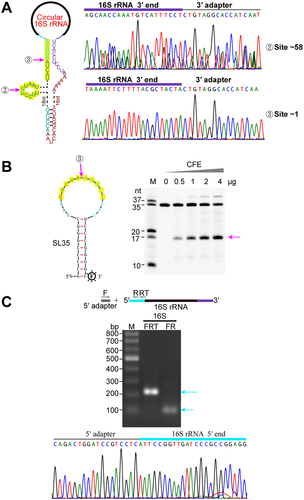
Nob1 cleavage is involved in the 3′ end maturation of 16S rRNA in M. maripaludis
Given linearization occurring at mature 5′ end of pre-16S rRNA, the 3′ end processing was also determined. Our previous Term-seq data revealed the mature 3′ end of the M. maripaludis 16S rRNA to be ‘UCACCUCC’ (), which lacks the last 3′ uridine in most other archaeal 16S rRNA [Citation16]. To validate this finding, a 3′-RACE assay was conducted as part of this study (). However, it is previously determined that the endonuclease Nob1 generates the mature 3′ end (5′-UCACCUCCU-3′) of the 16S rRNA in M. psychrophilus and P. horikoshii [Citation16,Citation31]. To investigate whether the M. maripaludis Nob1 (MmpNob1) is involved in the 3′ end maturation of 16S rRNA, an 80-nt RNA encompassing 54-nt upstream sequence of the 16S rRNA 3′ end and 26-nt downstream spacer was synthesized and used as a substrate. With addition of purified recombinant MmpNob1, two expected RNA fragments of 54-nt and 26-nt were produced (). Sequencing of the 3′-RACE products confirmed the presence of last uridine at the 3′ end of the 54-nt fragment (Fig. S4). Furthermore, two 37-nt stem-loop RNAs with a 9-GC base pairs and an 18-nt 3′-end sequence of 16S rRNA (Mmp-SL37 and Mpy-SL37) were synthesized to mimic the circular intermediate. MmpNob1 and MpyNob1 from M. psychrophilus were each tested and both produced a 16-nt fragment with the last uridine present as UCACCUCCU (), confirming that MmpNob1 is also the enzyme to process the 16S rRNA 3′ end, albeit with lower activity compared to its ortholog MpyNob1 in vitro. Therefore, we propose an additional cleavage to remove the last uridine for final 3′ end maturation of 16S rRNA after MmpNob1′s processing.
Figure 4. Endonuclease Nob1 cleaved at the 3′ end of 16S rRNA. (a) The mature 3′ end of 16S rRNA determined by 3′-RACE. The secondary structure and sequence of the 3′ end of 16S rRNA are depicted, and the endonucleolytic processing site of Nob1 is indicated by the purple arrow. The 3′-RACE products are indicated by arrows next to the agarose gel, and DNA sequencing shows the 3′ end of 16S rRNA. (b – c) In vitro enzymatic assay of Nob1 using the 80-nt linear (L80) RNA substrate (b) as well as 3′-FAM-labelled 37-nt circular-like stem-loop (SL37) substrate (c). L80 consists of 54 nt upstream and 26 nt downstream of the mature 3′ end of 16S rRNA, while SL37 comprises a stem with 9-GC base pairs and an 18-nt loop that embeds with 16S rRNA 3′-end cleavage sites of M. psychrophilus (Mpy-SL37) and M. maripaludis (Mmp-SL37), respectively. The 3′ end of the cleaved upstream fragment in (b) was determined by 3′-RACE shown in Fig. S4. Nob1 and the RNA substrate containing the 3′ terminal region of 16S rRNA of M. psychrophilus were used as a positive control in (c).
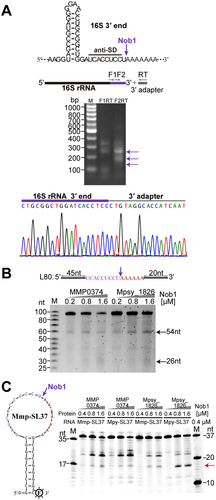
An RNA structure-dependent endonucleolytic processing at the mature 5′ end linearizes circular pre-23S rRNA
To determine the processing site of the circular pre-23S rRNA intermediate in M. maripaludis, the mature 5′ and 3′ ends of 23S rRNA were first investigated. Circularized RT-PCR (cRT-PCR) using intramolecular ligation by T4 RNA ligase was performed to identify the 5′ and 3′ ends of 23S rRNA. Two sites, 23S-M1 and 23S-M2, located in a predicted helix 1 were identified as the main 5′ ends of 23S rRNA (cyan arrows indicated in ). The abundances of these two sites appeared at a ratio of approximately 5:1 based on TA clone numbers. This was further verified by 5′-RACE, which detected an approximate ratio of 10:1 for site 23S-M1 to site 23S-M2 (), indicating that processing at site 23S-M1 primarily contributes to the 5′ end maturation of 23S rRNA. Both cRT-PCR and 3′-RACE assay determined the same mature 3′ end (23S-M3) in helix 1 of 23S rRNA (purple arrow in ). Noticeably, no other processing sites in the 3′ extension of M. maripaludis pre-23S rRNA were detected by 3′-RACE or previous Term-seq. Similarly, apart from sites 23S-M1 and 23S-M2, no other processing sites upstream of the mature 5′ end of 23S rRNA were detected by cRT-PCR or 5′-RACE. Based on these findings, we proposed that a single endonucleolytic cleavage at the 5′ end linearizes the circular pre-23S rRNA intermediate, resulting in the generation of a 38-nt 3′ extension. Notably, 3′-RACE did not capture any further processed products from the 38-nt 3′ extension in the M. maripaludis pre-23S rRNA, unlike in M. psychrophilus where 3′-5′ exonucleolytic products were found from a 48-nt 3′ extension [Citation16]. This difference could be attributed to the absence of known 3′-5′ exonuclease homologs in M. maripaludis [Citation36].
Figure 5. Linearization of circular pre-23S rRNA at the mature 5′ end. (a) Circularized RT-PCR (cRT-PCR) detected the 5′ and 3′ termini of 23S rRNA. The schematic on the left shows the circular pre-23S rRNA carrying the complete 38-nt spacer sequence. Thick black lines show mature rRNA bodies and the spacer sequences at the 5′ and 3′ ends in the circular region are indicated in dark cyan and red colours respectively. The mature 5′ and 3′ ends of 23S rRNA body are shown in cyan and purple colours, respectively. cRT-PCR was performed as described in Fig. 2. DNA sequencing of the reaction with T4 RNA ligase pretreatment (+) revealed the junction of mature 3′ end and 5′ end of 23S rRNA. Two mature 5′ ends were identified. (b – c) The 5′ and 3′ ends of 23S rRNA detected by 5′-RACE (b) and 3′-RACE (c) assays, respectively. Products from two rounds of PCR in RACE analysis were analysed on an agarose gel (upper panel) and by DNA sequencing (lower panel).
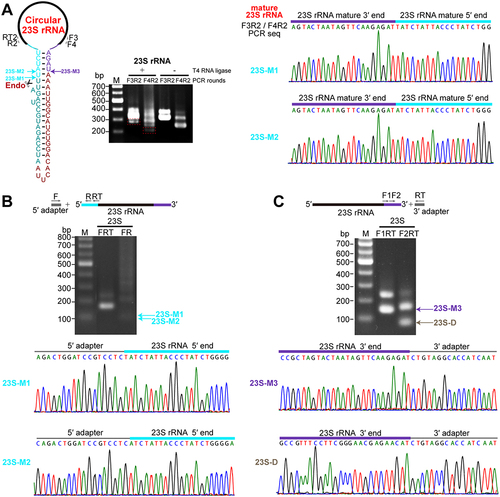
Endonucleolytic cleavages at both 5′ and 3′ ends contribute to the maturation of 5S rRNA
In M. maripaludis, the splicing cleavage at the pre-23S rRNA stem releases a downstream fragment containing pre-5S rRNA. Thereafter, the processing and maturation pathway of this cropped pre-5S rRNA was investigated. Primer extension revealed three sites near the 5′ end of 5S rRNA in the rrnC operon: one mature site (+1 nt) and two potential processing sites (−13 nt and − 1 nt, sites ④ and ⑤) (). This was further supported by 5′-RACE, which identified the mature 5′ end and a relatively abundant processing site (site ⑤) (). Meanwhile, the mature 3′ end of 5S rRNA was determined by 3′-RACE (). In addition, rnpB encoding RNase P RNA is located downstream of 5S rRNA gene in the rrnC operon, and these two components need to be separated. Two processing sites (sites ⑥ and ⑦ in ), located 3 or 6 nucleotides downstream of the mature 3′ end of 5S rRNA, were identified by dRNA-seq. These sites likely represent endonucleolytic processing events that separate 5S rRNA and RNase P RNA. Interestingly, motif search revealed that these four processing sites (sites ④–⑦ indicated in ) specially matched the aforementioned 10-nt A/U-rich motif (). This finding suggests the involvement of the unidentified endonuclease in the maturation of 5S rRNA, which undergoes a multi-step endonucleolytic processing at both ends. Moreover, we also identified the same 10-nt A/U-rich motif surrounding two processing sites in the fourth 5S rRNA located in the tRNA operon, with one site located 1 nt upstream of the mature 5′ end (site ⑧) and the other site (site ⑨) located 3 nt downstream the mature 3′ end (Fig. S5A, B). Therefore, the maturation of the M. maripaludis pre-5S rRNA may likely involve a multi-step endonucleolytic processing mechanism targeting the 10-nt A/U-rich motif. This represents a novel mode of 5S rRNA maturation pathway in archaea.
Figure 6. Maturation of 5S rRNA in M. maripaludis. (a) Primer extension identified the processing sites and the mature 5′ end of 5S rRNA, indicated by the coloured arrows corresponding to Fig. 1B. cDNA product was analysed in a denaturing polyacrylamide gel, in parallel with a sequencing ladder obtained with the same primer. (b – c) 5′-RACE and 3′-RACE assays were performed to determine the 5′ end and 3′ end of 5S rRNA, respectively. The upper schematics show 5′-RACE and 3′-RACE procedures. Two rounds of PCR products were analysed on agarose gel (upper panel) and subjected to DNA sequencing (lower panel).
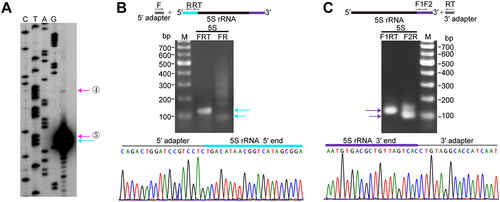
Discussion
Ribosomal RNA processing and maturation is an essential biological process for ribosome biogenesis [Citation5–7,Citation13]. However, compared to bacteria and eukaryotes, our knowledge of rRNA maturation in archaea is very limited, especially in the exponentially increasing number of archaeal species [Citation39]. This study focused on M. maripaludis, a representative archaeon lacking known 3′-5′ exonuclease homologs and comprehensively deciphered its rRNA processing and maturation pathway ().
Figure 7. The pre-rRNA maturation pathway in M. maripaludis. The complex rrnC operon, consisting of 16S-tRNAAla2-23S-5S-rnpB, is utilized as a representative example. The polycistronic RNA is transcribed from the transcription start site (TSS, indicated by a blue arrow). EndA cleaves at BHB motifs within the stem structure, resulting in the excision of pre-16S and pre-23S rRNAs and the formation of circular pre-16S and pre-23S rRNA intermediates. Endonucleases RNase P and RNase Z are responsible for the cleavage that releases tRNAAla2. Four endonucleolytic processing sites (sites ④ ⑤ ⑥ ⑦) flanking 5S rRNA separate both 5S and RNase P RNA from the polycistronic precursor. These four sites, characterized by a distinct 10-nt A/U-rich motif, are presumably cleaved by an unidentified endonuclease. The circular pre-16S and 23S rRNA intermediates undergo sequence-specific or structure-specific endonucleolytic cleavage at their respective mature 5′ ends. The 3′ extension of pre-16S rRNA is then trimmed stepwise through the endonucleolytic processing at site ② with similar 10-nt A/U-rich motif and 3′ processing by the endonuclease Nob1, leading to the generation of the mature 3′ end. The cropped 5′ ETS and ITS regions of different length are predicted to be eliminated by exonucleolytic degradation by potential 5′-3′ exonucleases (shown as pacman). Homologous 5′-3′ exonucleases are found as aRNase J (MMP0044) or aCPSF2 (MMP0431) encoded in M. maripaludis. The processing sites are indicated by the coloured arrows, similarly as shown in Fig. 1B. RNA structures of each molecule are schematically shown as aforementioned.
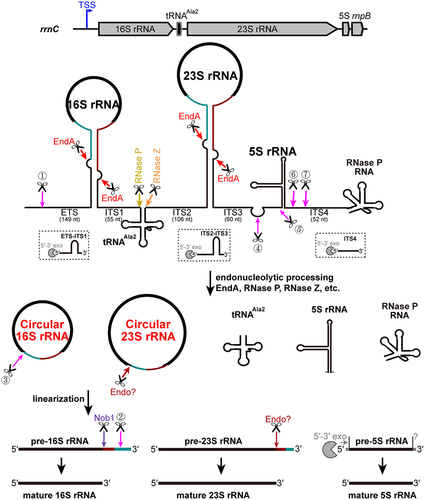
The initiation of pre-16S and pre-23S rRNA maturation involves the cleavages at the BHB motifs by the splicing endonuclease EndA, which is located in the helices formed by base pairing of the 5′ leader and 3′ trailer of 16S and 23S rRNAs, respectively. Subsequently, the RNA ligase RtcB catalyzes the coupled ligation of the EndA cleaved fragments, resulting in the formation of circular rRNA intermediates. In contrast to the Nob1-dependent 3′-end linearization of the circular pre-16S rRNA in M. psychrophilus, the linearization of the circular pre-16S rRNA intermediate and the generation of the mature 5′ end of the 16S rRNA occur simultaneously in M. maripaludis through endonucleolytic activity targeting a 10-nt A/U-rich motif near the 5′ terminus. The resulting 3′ extension of pre-16S rRNA is subsequently removed by cleavage at another site with 10-nt A/U-rich motif and at the 3′ end by Nob1. Furthermore, four processing sites with the same 10-nt A/U-rich motif were identified in the 5′ leader and 3′ trailer regions of the pre-5S rRNA. Additionally, the circular pre-23S rRNA is linearized probably by a structure-dependent endonucleolytic cleavage at the mature 5′ end. The processing of tRNAAla, encoded in 16S-23S internal transcribed spacer (ITS) region, involves cleavage by RNase P at the 5′ end and RNase Z at the 3′ end. Therefore, the endonucleolytic processing plays a crucial role in rRNA maturation in M. maripaludis. In addition to endonucleolytic activities, exonucleolytic activities also contribute to pre-rRNA processing in M. maripaludis. For example, the extra nucleotide remaining upstream of the mature 5′ end of 5S rRNA after endonucleolytic processing at site ⑤ needs to be trimmed by potential 5′-3′ exoribonucleases, such as archaeal β-CASP ribonucleases aRNase J and aCPSF2 [Citation12,Citation40], with homologs being identified in M. maripaludis. Exonucleolytic digestion may also be involved in the elimination of the 5′ ETS and ITS of pre-rRNA. The comprehensive mapping of the rRNA processing and maturation pathway in M. maripaludis sheds key insights into this model methanogen and reveals several novel strategies and processing modes for rRNA maturation. The formation of circular pre-rRNA intermediates, as well as the mechanisms of linearization and processing may have broader implications and be applicable to other archaeal species.
Formation of the circular pre-16S and pre-23S rRNA intermediates detected in M. maripaludis provides additional evidence for that the circularization and linearization is a relatively conserved mechanism of pre-rRNA maturation in archaea, which is also supported by the prevalence of rRNA BHB motifs and the presence of the conserved splicing endonuclease EndA among various archaeal phyla [Citation13,Citation16,Citation41]. Similar circular pre-16S and pre-23S rRNA intermediates were also recently reported in M. psychrophilus [Citation16] and in H. volcanii, P. furiosus and S. acidocaldarius detected by nanopore-based RNA sequencing [Citation22]. Knockdown of endA has been shown to result in a decrease in circularized intermediates, emphasizing the essential role of EndA in rRNA processing in vivo [Citation27]. Moreover, the pre-rRNA intermediates observed in S. acidocaldarius including ligation at the BHB motif of the upstream region of the 16S leader and downstream region of the 16S trailer and continues to the downstream 23S rRNA suggests that the 16S rRNA BHB-processing could occur prior to 23S rRNA maturation [Citation22]. However, the subsequent linearization of the circular intermediates, which is an essential prerequisite for further maturation of rRNAs, has rarely evaluated in archaeal species. Recent study has determined that in M. psychrophilus, Nob1 mediates the linearization of pre-16S rRNA at the mature 3′ end and an unknown endonuclease is responsible for linearization of pre-23S rRNA at the mature 5′ end [Citation16]. However, distinct from that, endonucleolytic processing at the mature 5′ end of 16S rRNA by an unidentified endonuclease targeting a 10 nt A/U-rich motif could linearize the circular pre-16S rRNA intermediate in M. maripaludis (). This provides one another strategy for linearizing circular pre-16S rRNA intermediates in archaea. Through multiple sequence alignment, a large abundance of archaeal species was found to possess the similar 10-nt A/U-rich motif with conserved U+2 at the 16S rRNA 5′ end (Fig. S6). Supportively, nanopore-based RNA sequencing detected the same intermediate result from linearization of the circular pre-16S rRNA at the mature 16S rRNA 5′ end in H. volcanii with relatively abundance [Citation22]. Thus, the linearization pathway of circular pre-16S rRNA found in M. maripaludis might be widely employed by other archaeal species.
Interestingly, the mature 3′ end of 16S rRNA in M. maripaludis was found to be UCACCUCC (), lacking the last uridine base of the 16S rRNA 3′ end (UCACCUCCU) produced by the conserved endonuclease Nob1 in other archaea such as M. psychrophilus and P. horikoshii [Citation16,Citation31]. In vitro enzymatic reaction confirmed that the Nob1 homolog in M. maripaludis is capable of cleaving at UCACCUCCU as well ( and S4). Therefore, it appears that after Nob1 cleavage in M. maripaludis, the last uridine should be further removed. Similar heterogeneity at 3′ termini of the 16S rRNA has also been observed in other archaeal species like Methanococcus vannielii and Halobacterium salinarum (formerly known as H. halobium) [Citation42]. It is generally believed that the prokaryotic Shine-Dalgarno (SD) sequence (typically GGAGG) pairs with the anti-SD sequence (CCUCC) encoded in the 3′ end of 16S rRNA [Citation43]. However, recent genome-wide TSS identification in several archaeal species have revealed that the SD sequence is GGUGA and the complementary sequence is UCACC [Citation44–48]. This suggests that lack of the last uridine in 16S rRNA 3′ end, as observed in Methanococcus, would not impair the interaction between the SD and anti-SD sequence. Multiple sequence alignment of 3′ tail of 16S rRNA also revealed that the last two nucleotides (CU) in 16S rRNA 3′ terminus are not conserved among archaeal species (Fig. S6), indicating heterogeneous 16S rRNA 3′ termini and the consequently diversified usage of anti-SD among archaea.
Regarding linearization of circular pre-23S rRNA in M. maripaludis, it occurs similarly at the mature 5′ end, but likely through a structure-specific endonuclease rather than a sequence-specific one. The 5′ and 3′ end of mature 23S was found to form a 4-bp stem of helix 1 with or without a 1 residue overhang at the 5′ end in M. maripaludis (). This feature may be relatively conserved among different archaeal species [Citation49]. Similarly, the mature 5′ and 3′ end of 5S rRNA form an 11-bp stable stem with a 1 residue overhang at the 3′ end. Additionally, the RNase P RNA encoded in rrnC operon exhibits a 6-bp stem structure with overhangs at both ends (). This suggests that the terminal stem structure around the mature ends of 23S rRNA, 5S rRNA and RNase P RNA may serve as a molecular ruler or roadblock to guide the exo- and endonucleolytic processing, similar to how exonuclease-mediated processing stops at RNA hairpin structures [Citation50]. Additionally, another site located 88 nt upstream of the mature 3′ end of 23S rRNA was identified in the 3′-RACE analysis (), matching the 10-nt A/U-rich motif. Structural mapping revealed that this site is within helix 100 of 23S rRNA body (Fig. S7), suggesting that the unidentified endonuclease targeting the 10-nt A/U-rich motif may be also involved in 23S rRNA decay or turnover. Thus, endonucleolytic cleavage could play pleiotropic roles in maturation and degradation of pre-rRNA in M. maripaludis.
One additional interesting finding in this study is the abundance of processing sites with featured 10-nt A/U-rich motif flanking the 5S rRNA in M. maripaludis. This suggests that the maturation of pre-5S rRNA in this organism primarily depends on multi-step endonucleolytic cleavages. This distinct maturation pathway differs from the known pathways reported for 5S rRNA maturation in archaea [Citation51]. There are three maturation pathways of 5S rRNA reported in archaea [Citation16]. One of them involves a combination of endo- and exonucleolytic activities and has been described in M. psychrophilus, where the endonucleolytic activity depends on the unidentified endonuclease acting on the same 10-nt A/U-rich motif upstream of 5S rRNA [Citation16]. In M. maripaludis, cleavage at the 10-nt motif in pre-5S rRNA not only generates a 5′ end with only one extra residue to be removed for final maturation but also releases the downstream RNase P RNA ( and S5). Multiple sequence alignment suggests that the endonucleolytic processing at the 10-nt A/U-rich motif in the 5′ leader of 5S rRNA could be prevalent in methanogenic archaea (Fig. S8). This endonucleolytic processing could accelerate the subsequent exonucleolytic digestion and thus increase the maturation rate of 5S rRNA.
In conclusion, this study provides a comprehensive understanding of the complete processing and maturation pathway of pre-rRNA in M. maripaludis. It reveals that endonucleolytic processing, particularly mediated by the unidentified endonuclease targeting the 10-nt A/U-rich motif, plays a crucial role in the linearization of circular processing intermediates and the maturation of 5S rRNA. This finding is consistent with the absence of 3′-5′ exonuclease in M. maripaludis and suggests that this pathway may be utilized in other archaeal organisms that lack 3′-5′ exonucleases.
Materials and methods
Strain culture and RNA isolation
M. maripaludis strain S0001 was cultured in formate medium (McF) reduced with cysteine hydrochloride at 37°C as described previously [Citation52,Citation53]. The growth of the strain was monitored by measuring the optical densities at 600 nm (OD600). Cells from the exponential or other phases throughout the growth period were collected by centrifugation at 10, 000 rpm for 5 min at 4°C. RNA isolation was performed using the standard TRIzol (Ambion) protocol as previously described [Citation28]. Briefly, TRIzol (1 mL at room temperature) was add to the cell pellets and mixed thoroughly. After the addition of 200 μL of chloroform, the samples were mixed by inversion and then incubated at room temperature. Subsequently, the samples were centrifuged at 4°C for 10 min at maximal speed. The upper phase containing the RNA was transferred into a new tube, and RNA precipitation was achieved by adding isopropanol. The RNA pellet was washed twice with 75% ethanol, and then resuspended in RNase-free water. The RNA concentration was quantified using a NanoDrop (Thermo Fisher Scientific).
Processing site mapping and verification
To identify the processing sites, circularized RT-PCR (cRT-PCR) was performed to simultaneously determine the 5′ and 3′ termini of RNA, following a similar protocol as previously described [Citation54]. A negative control without T4 RNA ligase was included. Primer extension and 5′-RACE was used to detect the 5′ ends of RNA, while 3′-RACE was used to detect the 3′ ends [Citation16,Citation38,Citation55]. Primers for these analyses were designed using Primer Premier and listed in Table S1. In the RACE analysis, a 5′ RNA adapter (5′-CAGACUGGAUCCGUCGUC-3′, Integrated DNA Technologies) and a universal miRNA cloning linker (5′-rAppCTGTAGGCACCATCAAT-NH2-3′, New England Biolabs) was used for 5′-RACE and 3′-RACE, respectively. PCR products from cRT-PCR, 5′-RACE and 3′-RACE were purified and cloned into the pMD19-T vector (TaKaRa). At least 10 positive clones were sequenced for each analysis, and the processing or mature sites of the rRNA were identified based on the sequence information obtained. Additionally, transcriptomic data, including dRNA-seq and Term-seq [Citation28,Citation37], were utilized to identify or validate the 5′ ends and 3′ ends of RNA, respectively.
Bioinformatic analysis
BHB motifs of 16S and 23S rRNA were identified by performing sequence alignment using the 5′ leader and 3′ trailer sequence flanking the mature rRNA. A candidate BHB motif was defined as a 7-bp unpaired region between two aligned sequences. Multiple sequence alignments were conducted using DNAMAN software with default parameters. The 16S and 23S rRNA sequences of representative archaeal species were obtained from the NCBI or KEGG database [Citation56]. RNA secondary structures were predicted using the RNA Folding Form V2.3 in Mfold web server [Citation57] and displayed using the RnaViz program [Citation58] with manual curation. The motif of processing sites was searched by MEME program [Citation59], and the consensus sequence was generated by WebLogo 3 [Citation60].
Qualitative and quantitative analysis of circular intermediates
For the qualitative analysis of circular intermediates of 16S and 23S rRNA, Northern blot was performed following the previously described protocol [Citation16]. The quantitative analysis of circular intermediates was determined using quantitative reverse transcription PCR (qRT-PCR). 10 mL of M. maripaludis cultures were collected at different growth phases, and total RNA was extracted using phenol/chloroform treatment. The cDNA was synthesized by reverse transcription of 1 μg total RNA using the ReverTra Ace qPCR RT MasterMix with gDNA Remover (TOYOBO). Quantitative PCR was performed using THUNDERBIRD SYBR qPCR Mix (TOYOBO) on a Mastercycler ep realplex2 (Eppendorf). The expression levels were calculated using the absolute quantitative method.
Enzymatic assay
Protein expression, purification, and enzymatic assay of EndA and Nob1 were performed as previously described [Citation16], with the exception that the test temperature was shifted to 37°C to match the optimal growth temperature of M. maripaludis. The cleavage of the 10-nt motif RNA was determined using cell-free extract (CFE). The CFE was obtained by centrifuging 50 mL of cultures at 5,000×g for 10 min, followed by concentration in 1 mL of affinity buffer (20 mM Tris-HCl pH 8.0, 150 mM KCl, 1 mM DTT). The resuspended pellets were sonicated for 10 min at 400 W and kept on ice to prevent protein denaturation and degradation. The suspension was cleared by centrifugation at 16,000×g for 20 min at 4°C. Total protein concentration of the CFE was determined using the Pierce BCA Protein Assay Kit (Thermo Fisher Scientific) according to the manufacturer’s instructions. A 3′-FAM-labelled RNA substrate (2 pmol) was added to the enzymatic system. All reactions were analysed by running on a 20% denaturing PAGE and detected based on the fluorescence signal captured using Tanon-5200Multi chemiluminescent imaging system (Tanon Science & Technology). Probes and RNA substrates used in this study are listed in Table S2.
Author contributions
LQ, XD, and JL conceptualized and acquired funding. LQ, HL, JG performed the experiments. All authors interpreted the experimental data and assisted with the preparation of the manuscript. LQ, KD, XW, XD, and JL wrote the manuscript. All authors approved the final manuscript.
Supplemental Material
Download MS Word (3.1 MB)Acknowledgments
We thank Professor William B. Whitman at the University of Georgia providing strains and plasmids used in this work.
Disclosure statement
No potential conflict of interest was reported by the author(s).
Data availability statement
The original contributions presented in the study are included in the article/Supplementary material, further inquiries can be directed to the corresponding author(s).
Supplementary material
Supplemental data for this article can be accessed online at https://doi.org/10.1080/15476286.2023.2258035.
Additional information
Funding
References
- Hage AE, Tollervey D. A surfeit of factors: why is ribosome assembly so much more complicated in eukaryotes than bacteria? RNA Biol. 2004;1(1):10–15. doi: 10.4161/rna.1.1.932
- Woolford JL Jr., Baserga SJ. Ribosome biogenesis in the yeast Saccharomyces cerevisiae. Genetics. 2013;195(3):643–681. doi: 10.1534/genetics.113.153197
- Yip WS, Vincent NG, Baserga SJ. Ribonucleoproteins in archaeal pre-rRNA processing and modification. Archaea. 2013;2013:614735. doi: 10.1155/2013/614735
- Henras AK, Plisson-Chastang C, O’Donohue MF, et al. An overview of pre-ribosomal RNA processing in eukaryotes. Wiley Interdiscip Rev RNA. 2015;6(2):225–242. doi: 10.1002/wrna.1269
- Shajani Z, Sykes MT, Williamson JR. Assembly of bacterial ribosomes. Annu Rev Biochem. 2011;80(1):501–526. doi: 10.1146/annurev-biochem-062608-160432
- Peña C, Hurt E, Panse VG. Eukaryotic ribosome assembly, transport and quality control. Nat Struct Mol Biol. 2017;24(9):689–699. doi: 10.1038/nsmb.3454
- Baßler J, Hurt E. Eukaryotic ribosome assembly. Annu Rev Biochem. 2019;88(1):281–306. doi: 10.1146/annurev-biochem-013118-110817
- Williamson JR. Cellular physiology of bacterial ribosome assembly. FASEB J. 2010;24(S1):197.2. doi: 10.1096/fasebj.24.1_supplement.197.2
- Young RA, Steitz JA. Complementary sequences 1700 nucleotides apart form a ribonuclease III cleavage site in Escherichia coli ribosomal precursor RNA. Proc Natl Acad Sci, USA. 1978;75(8):3593–3597. doi: 10.1073/pnas.75.8.3593
- Deutscher MP. Maturation and degradation of ribosomal RNA in bacteria. Prog Mol Biol Transl Sci. 2009;85:369–391.
- Ferreira-Cerca S. Life and death of ribosomes in archaea. In: Clouet-d’Orval B, editor. RNA metabolism and gene expression in archaea. Cham: Springer International Publishing AG; 2017. p. 129–158. doi: 10.1007/978-3-319-65795-0_6
- Clouet-d’Orval B, Batista M, Bouvier M, et al. Insights into RNA-processing pathways and associated RNA-degrading enzymes in archaea. FEMS Microbiol Rev. 2018;42(5):579–613. doi: 10.1093/femsre/fuy016
- Londei P, Ferreira-Cerca S. Ribosome biogenesis in archaea. Front Microbiol. 2021;12:686977. doi: 10.3389/fmicb.2021.686977
- Tang TH, Rozhdestvensky TS, d’Orval BC, et al. RNomics in archaea reveals a further link between splicing of archaeal introns and rRNA processing. Nucleic Acids Res. 2002;30(4):921–930. doi: 10.1093/nar/30.4.921
- Danan M, Schwartz S, Edelheit S, et al. Transcriptome-wide discovery of circular RNAs in archaea. Nucleic Acids Res. 2012;40(7):3131–3142. doi: 10.1093/nar/gkr1009
- Qi L, Li J, Jia J, et al. Comprehensive analysis of the pre-ribosomal RNA maturation pathway in a methanoarchaeon exposes the conserved circularization and linearization mode in archaea. RNA Biol. 2020;17(10):1427–1441. doi: 10.1080/15476286.2020.1771946
- Chant J, Dennis P. Archaebacteria: transcription and processing of ribosomal RNA sequences in Halobacterium cutirubrum. EMBO J. 1986;5(5):1091–1097. doi: 10.1002/j.1460-2075.1986.tb04327.x
- Ree HK, Zimmermann RA. Organization and expression of the 16S, 23S and 5S ribosomal RNA genes from the archaebacterium Thermoplasma acidophilum. Nucleic Acids Res. 1990;18(15):4471–4478. doi: 10.1093/nar/18.15.4471
- Durovic P, Dennis PP. Separate pathways for excision and processing of 16S and 23S rRNA from the primary rRNA operon transcript from the hyperthermophilic archaebacterium Sulfolobus acidocaldarius: similarities to eukaryotic rRNA processing. Mol Microbiol. 1994;13(2):229–242. doi: 10.1111/j.1365-2958.1994.tb00418.x
- Dennis PP, Ziesche S, Mylvaganam S. Transcription analysis of two disparate rRNA operons in the halophilic archaeon Haloarcula marismortui. J Bacteriol. 1998;180(18):4804–4813. doi: 10.1128/JB.180.18.4804-4813.1998
- Ciammaruconi A, Londei P. In vitro processing of the 16S rRNA of the thermophilic archaeon Sulfolobus solfataricus. J Bacteriol. 2001;183(13):3866–3874. doi: 10.1128/JB.183.13.3866-3874.2001
- Grünberger F, Jüttner M, Knüppel R, et al. Nanopore-based RNA sequencing deciphers the formation, processing, and modification steps of rRNA intermediates in archaea. RNA. 2023;29(8):1255–1273. doi: 10.1261/rna.079636.123
- Redko Y, de la Sierra-Gallay IL, Condon C. When all’s zed and done: the structure and function of RNase Z in prokaryotes. Nat Rev Microbiol. 2007;5(4):278–286. doi: 10.1038/nrmicro1622
- Wang X, Gu X, Li J, et al. Characterization of the methanomicrobial archaeal RNase Zs for processing the CCA-containing tRNA precursors. Front Microbiol. 2020;11:1851. doi: 10.3389/fmicb.2020.01851
- Phan HD, Lai LB, Zahurancik WJ, et al. The many faces of RNA-based RNase P, an RNA-world relic. Trends Biochem Sci. 2021;46(12):976–991. doi: 10.1016/j.tibs.2021.07.005
- Hirata A. Recent insights into the structure, function, and evolution of the RNA-splicing endonucleases. Front Genet. 2019;10:103. doi: 10.3389/fgene.2019.00103
- Schwarz TS, Berkemer SJ, Bernhart SH, et al. Splicing endonuclease is an important player in rRNA and tRNA maturation in archaea. Front Microbiol. 2020;11:594838. doi: 10.3389/fmicb.2020.594838
- Yue L, Li J, Zhang B, et al. The conserved ribonuclease aCPSF1 triggers genome-wide transcription termination of archaea via a 3’-end cleavage mode. Nucleic Acids Res. 2020;48(17):9589–9605. doi: 10.1093/nar/gkaa702
- Li J, Zheng X, Li L, et al. The archaeal transcription termination factor aCPSF1 is a robust phylogenetic marker for archaeal taxonomy. Microbiol Spectr. 2021;9(3):e0153921. doi: 10.1128/spectrum.01539-21
- Li J, Yue L, Li Z, et al. aCPSF1 cooperates with terminator U-tract to dictate archaeal transcription termination efficacy. Elife. 2021;10:e70464. doi: 10.7554/eLife.70464
- Veith T, Martin R, Wurm JP, et al. Structural and functional analysis of the archaeal endoribonuclease Nob1. Nucleic Acids Res. 2012;40(7):3259–3274. doi: 10.1093/nar/gkr1186
- Mackie GA. RNase E: at the interface of bacterial RNA processing and decay. Nat Rev Microbiol. 2013;11(1):45–57. doi: 10.1038/nrmicro2930
- Baumgardt K, Gilet L, Figaro S, et al. The essential nature of YqfG, a YbeY homologue required for 3′ maturation of Bacillus subtilis 16S ribosomal RNA is suppressed by deletion of RNase R. Nucleic Acids Res. 2018;46(16):8605–8615. doi: 10.1093/nar/gky488
- Mohanty BK, Kushner SR, Storz G, et al. Enzymes involved in posttranscriptional RNA metabolism in Gram-negative bacteria. Microbiol Spectr. 2018;6(2):RWR-0011–2017. doi: 10.1128/microbiolspec.RWR-0011-2017
- Clouet-d’Orval B, Phung DK, Langendijk-Genevaux PS, et al. Universal RNA-degrading enzymes in archaea: prevalence, activities and functions of β-CASP ribonucleases. Biochimie. 2015;118:278–285. doi: 10.1016/j.biochi.2015.05.021
- Phung DK, Rinaldi D, Langendijk-Genevaux PS, et al. Archaeal β-CASP ribonucleases of the aCPSF1 family are orthologs of the eukaryal CPSF-73 factor. Nucleic Acids Res. 2013;41(2):1091–1103. doi: 10.1093/nar/gks1237
- Li J, Zhang B, Zhou L, et al. The archaeal RNA chaperone TRAM0076 shapes the transcriptome and optimizes the growth of Methanococcus maripaludis. PLoS Genet. 2019;15(8):e1008328. doi: 10.1371/journal.pgen.1008328
- Qi L, Yue L, Feng D, et al. Genome-wide mRNA processing in methanogenic archaea reveals post-transcriptional regulation of ribosomal protein synthesis. Nucleic Acids Res. 2017;45(12):7285–7298. doi: 10.1093/nar/gkx454
- Ferreira-Cerca S. The dark side of the ribosome life cycle. RNA Biol. 2022;19(1):1045–1049. doi: 10.1080/15476286.2022.2121421
- Zheng X, Feng N, Li D, et al. New molecular insights into an archaeal RNase J reveal a conserved processive exoribonucleolysis mechanism of the RNase J family. Mol Microbiol. 2017;106(3):351–366. doi: 10.1111/mmi.13769
- Jüttner M, Weiß M, Ostheimer N, et al. A versatile cis-acting element reporter system to study the function, maturation and stability of ribosomal RNA mutants in archaea. Nucleic Acids Res. 2020;48(4):2073–2090. doi: 10.1093/nar/gkz1156
- Jarsch M, Böck A. DNA sequence of the 16S rRNA/23S rRNA intercistronic spacer of two rDNA operons of the archaebacterium Methanococcus vannielii. Nucleic Acids Res. 1983;11(21):7537–7544. doi: 10.1093/nar/11.21.7537
- Nakagawa S, Niimura Y, Miura K, et al. Dynamic evolution of translation initiation mechanisms in prokaryotes. Proc Natl Acad Sci, USA. 2010;107(14):6382–6387. doi: 10.1073/pnas.1002036107
- Jäger D, Förstner KU, Sharma CM, et al. Primary transcriptome map of the hyperthermophilic archaeon Thermococcus kodakarensis. BMC Genomics. 2014;15(1):684. doi: 10.1186/1471-2164-15-684
- Li J, Qi L, Guo Y, et al. Global mapping transcriptional start sites revealed both transcriptional and post-transcriptional regulation of cold adaptation in the methanogenic archaeon Methanolobus psychrophilus. Sci Rep. 2015;5(1):9209. doi: 10.1038/srep09209
- Cho S, Kim MS, Jeong Y, et al. Genome-wide primary transcriptome analysis of H2-producing archaeon Thermococcus onnurineus NA1. Sci Rep. 2017;7(1):43044. doi: 10.1038/srep43044
- Smollett K, Blombach F, Reichelt R, et al. A global analysis of transcription reveals two modes of Spt4/5 recruitment to archaeal RNA polymerase. Nat Microbiol. 2017;2(5):17021. doi: 10.1038/nmicrobiol.2017.21
- Schmitt E, Coureux PD, Kazan R, et al. Recent advances in archaeal translation initiation. Front Microbiol. 2020;11:584152. doi: 10.3389/fmicb.2020.584152
- Leffers H, Kjems J, Ostergaard L, et al. Evolutionary relationships amongst archaebacteria. A comparative study of 23 S ribosomal RNAs of a sulphur-dependent extreme thermophile, an extreme halophile and a thermophilic methanogen. J Mol Biol. 1987;195(1):43–61. doi: 10.1016/0022-2836(87)90326-3
- Lécrivain AL, Le Rhun A, Renault TT, et al. In vivo 3’-to-5’ exoribonuclease targetomes of Streptococcus pyogenes. Proc Natl Acad Sci, USA. 2018;115(46):11814–11819. doi: 10.1073/pnas.1809663115
- Hölzle A, Fischer S, Heyer R, et al. Maturation of the 5S rRNA 5′ end is catalyzed in vitro by the endoribonuclease tRNase Z in the archaeon H. volcanii. RNA. 2008;14(5):928–937. doi: 10.1261/rna.933208
- Long F, Wang L, Lupa B, et al. A flexible system for cultivation of Methanococcus and other formate-utilizing methanogens. Archaea. 2017;2017:7046026. doi: 10.1155/2017/7046026
- Li J, Zhang L, Xu Q, et al. CRISPR-Cas9 toolkit for genome editing in an autotrophic CO2-fixing methanogenic archaeon. Microbiol Spectr. 2022;10(4):e0116522. doi: 10.1128/spectrum.01165-22
- Slomovic S, Schuster G. Circularized RT-PCR (cRT-PCR): analysis of the 5′ ends, 3′ ends, and poly(A) tails of RNA. Methods Enzymol. 2013;530:227–251.
- Zhang J, Olsen GJ. Messenger RNA processing in Methanocaldococcus (Methanococcus) jannaschii. RNA. 2009;15(10):1909–1916. doi: 10.1261/rna.1715209
- Kanehisa M, Furumichi M, Tanabe M, et al. KEGG: new perspectives on genomes, pathways, diseases and drugs. Nucleic Acids Res. 2017;45(D1):D353–D361. doi: 10.1093/nar/gkw1092
- Zuker M. Mfold web server for nucleic acid folding and hybridization prediction. Nucleic Acids Res. 2003;31(13):3406–3415. doi: 10.1093/nar/gkg595
- De Rijk P, Wuyts J, De Wachter R. RnaViz 2: an improved representation of RNA secondary structure. Bioinformatics. 2003;19(2):299–300. doi: 10.1093/bioinformatics/19.2.299
- Bailey TL, Johnson J, Grant CE, et al. The MEME suite. Nucleic Acids Res. 2015;43(W1):W39–W49. doi: 10.1093/nar/gkv416
- Crooks GE, Hon G, Chandonia JM, et al. WebLogo: a sequence logo generator. Genome Res. 2004;14(6):1188–1190. doi: 10.1101/gr.849004