ABSTRACT
The long noncoding RNA (lncR) ANRIL in the human genome is an established genetic risk factor for atherosclerosis, periodontitis, diabetes, and cancer. However, the regulatory role of lncR-ANRIL in bone and adipose tissue metabolism remains unclear. To elucidate the function of lncRNA ANRIL in a mouse model, we investigated its ortholog, AK148321 (referred to as lncR-APDC), located on chr4 of the mouse genome, which is hypothesized to have similar biological functions to ANRIL. We initially revealed that lncR-APDC in mouse bone marrow cells (BMSCs) and lncR-ANRIL in human osteoblasts (hFOBs) are both increased during early osteogenesis. Subsequently, we examined the osteogenesis, adipogenesis, osteoclastogenesis function with lncR-APDC deletion/overexpression cell models. In vivo, we compared the phenotypic differences in bone and adipose tissue between APDC-KO and wild-type mice. Our findings demonstrated that lncR-APDC deficiency impaired osteogenesis while promoting adipogenesis and osteoclastogenesis. Conversely, the overexpression of lncR-APDC stimulated osteogenesis, but impaired adipogenesis and osteoclastogenesis. Furthermore, KDM6B was downregulated with lncR-APDC deficiency and upregulated with overexpression. Through binding-site analysis, we identified miR-99a as a potential target of lncR-APDC. The results suggest that lncR-APDC exerts its osteogenic function via miR-99a/KDM6B/Hox pathways. Additionally, osteoclasto-osteogenic imbalance was mediated by lncR-APDC through MAPK/p38 and TLR4/MyD88 activation. These findings highlight the pivotal role of lncR-APDC as a key regulator in bone and fat tissue metabolism. It shows potential therapeutic for addressing imbalances in osteogenesis, adipogenesis, and osteoclastogenesis.
Introduction
Long non-coding RNAs (lncRNAs), which consist of over 200 nucleotides in length, exhibit unique expression patterns of biological functions in genome organization and life processes [Citation1–4]. Emerging evidence suggest that lncRNAs regulate specific gene expression by competing with miRNA for the same binding site on mRNA, thus eliminating miRNA-induced repression and stabilizing the corresponding mRNA [Citation5,Citation6]. In humans, lncRNA-ANRIL, also recognized as CDKN2BAS, is a genetic risk factor for atherosclerosis, coronary artery disease (CAD), myocardial infarction (MI), periodontitis (PD), diabetes and cancer [Citation7–19]. Previous studies have demonstrated that ANRIL promotes angiogenesis in cerebral infarctions associated with diabetes mellitus through VEGF upregulation via activation of the NF-κB signalling pathway [Citation20]. It is still unclear how regulating ANRIL results in cellular or physiological processes associated with bone and adipose tissue metabolism. No ANRIL exons identified in mice, there exists limited studies exploring the fundamental biological disease and potential therapeutic mechanisms attributed to ANRIL via rodent models [Citation21].
To further gain insight, we studied the mouse lncRNA-ANRIL ortholog, AK148321 (a 70 kb mouse interval on chromosome 4), which has 50% base pair similarity human to mouse [Citation22,Citation23]. AK148321 inferred to be a genetic risk factor for atherosclerosis, as well PD, diabetes, and cancer, was coined lncR-APDC (atherosclerosis, periodontitis, diabetes, and cancer). Just like ANRIL, the precise regulatory role of lncR-APDC remains an enigma. Evidently, ANRIL is closely related to inflammatory milieu and metabolic disorders [Citation24–27]. For bone, the inflammatory milieu can cause disturbances in osteoclastic-osteogenic equilibrium, which may be related to the TLR4-mediated signalling pathway [Citation28,Citation29]. Also, our preceding research has illuminated lysine (K)-specific demethylase 6B (KDM6B), a target of miR-99a, is distinctively regulated during osteogenic differentiation [Citation30]. Based on these foundational findings, we sought to further understand the mechanism of lncR-APDC regulation of bone and adipose tissue metabolic disoders with inflammatory environment.
Our objective is to enhance our comprehension of the mechanistic regulatory role of lncR-APDC in bone and adipose tissue metabolism. AK148321 was confirmed to be severely reduced in these chr4 Δ70kb/70kb mice [Citation23]. Previously, chr4 Δ70kb/70kb mice have been utilized as a model to investigate regulatory features of AK148321 during development and in vascular diseases [Citation31]. Chr4 Δ70kb/70kb mice have also proven to be effectively utilized to investigate the functions of ANRIL. Within our laboratory, using the chr4 Δ70kb/70kb mouse strain, we designed an APDC-knockout (APDC-KO) mouse. In this paper, we identify the phenotypic variations in bone and adipose tissue between APDC-KO and wild type mice. With deletion/overexpression of lncR-APDC, osteogenesis, adipogenesis, osteoclastogenesis, molecular signalling pathway and mechanism of osteogenic function were explored. The gathered data strongly propose that lncR-APDC acts for an important regulatory element in bone and fat tissue metabolism that has therapeutic possibility for treating bone disorders of which metabolism is disturbed.
Materials and methods
APDC-KO mouse model and genotyping
The mouse model in this study sourced from the Mutant Mouse Resource & Research Center (MMRRC) is supported from the National Institutes of Health. To creat this model, lncRNA AK148321 was deleted in W4/129S6 ES cells through Cre/lox P recombination. The severely reduced expression of AK148321 was confirmed in these chr4 Δ70kb/70kb mice [Citation23]. APDC-KO mice enrolled in this study underwent confirmational genotyping. To further confirm the APDC-null genotypes of this strain, tail DNA samples were extracted by DNeasy Blood & Tissue Kits, and genomic DNA genotyping analysis (QIAGEN, MD, USA) was performed with standard gDNA qPCR (primers found in Table S1) and gel electrophoresis was performed. All animals with the same genotype and same gender were randomized to the groups.
Plasmid transfection
In order to achieve elevated expression levels of lncR-APDC in bone marrow stromal cells (BMSCs), osteoblasts (OBs), and bone marrow macrophages (BMMs), a plasmid vector was constructed with a lncR-APDC insert. The integrity of the vector was confirmed by digestion with KpnI-NotI, sequenced to confirm the correct DNA orientation and sequence (State Key Laboratory of Oral Disease, Sichuan University, Chengdu, China). To complete transfection, BMSCs, OBs and BMMs were transfected with the plasmid vector using lipofectamine 3000 reagents (Thermo Fisher Scientific, MA, USA). After transfected for 24 h, harvested cells were analysed to verify overexpression of lncR-APDC.
Cell culture and cytological staining
BMSCs, OBs and BMMs were isolated from 4 to 6 weeks old C57BL/6J, APDC-KO, and wild type (WT) mice. Isolated cells or transfected cells were plated in 10 cm dishes and maintained in αMEM supplemented with 10% FBS and 1% penicillin/streptomycin. Osteogenic differentiation of BMSCs/OBs was carried out using αMEM medium (A1049001, Glo®, MT, USA) containing 50 μg/ml ascorbic acid (Sigma-Aldrich, MO, USA), 10 mM b-glycerophosphate (Sigma-Aldrich) and 100 nM dexamethasone (Sigma-Aldrich). To promote adipogenic differentiation in BMSCs, treatment with dexamethasone (1 μM), 3-isobutyl-1-methylxanthine (IBMX) (0.5 mM), and insulin (0.5 mM) for 3 days was followed by subsequent insulin (0.5 mM) treatment. Osteoclastogenic differentiation of BMMs was processed with receptor activator of nuclear factor kappa B ligand (RANKL) (50 ng/ml) and macrophage-colony stimulating factor (M-CSF)-1 (10 ng/ml) following MSCF-1 pretreatment for 3 days. The human foetal osteoblasts (hFOBs) (Gibco™ CRL-11372™, USA) were cultured in DMEM with 10% FBS and 1% penicillin/streptomycin with the osteogenesis-inducing medium.
Osteogenic, adipogenic and osteoclastogenic differentiation were analysed via cytological staining (Alizarin red staining for osteogenesis (Sigma-Aldrich), Oil red O staining for adipogenesis (Sigma-Aldrich), trap staining for osteoclastogenesis (Sigma-Aldrich)).
RNA extraction and quantitative real-time polymerase chain reaction (qRT-PCR) [Citation32]
Total RNA of aforementioned cells was extracted with Quick-RNA Miniprep Kit (ZYMO Research, CA, USA). Total RNA from white adipose tissue (WAT) or bone tissues of WT/APDC-KO mice was extracted through using TRIzol reagent (Thermo Fisher Scientific, USA) with protocol as lysing samples, separating phases and isolating RNA. Isolated RNA was reverse-transcribed with the PrimeScript™ RT Reagent Kit with gDNA Eraser (Takara, Japan). Utilizng the SYBR-Green 2X Master Mix (Thermo Fisher Scientific, USA) for qRT-PCR.
Total miRNAs of aforementioned cells were extracted via the miRNeasy Mini Kit (QIAGEN, MD, USA), and reverse-transcribed by the miRCURY LNA RT Kit (QIAGEN, MD, USA). The expression of miR-99a-5p was treated by miRCURY LNA SYBR Green PCR Kit (QIAGEN, MD, USA) for analysing.
The specific primers are listed in Table S3.
Western-blot analysis [Citation33]
Proteins of from the previously mentioned cells or BMMs lysed through RIPA lysis buffer (Thermo Fisher Scientific, USA) were isolated by Sodium Dodecyl Sulfate polyacrylamide gel electrophoresis (SDS-PAGE) (Sigma-Aldrich), which were transferred to Polyvinylidene fluoride (PVDF) membranes. The membranes blocked with 5% skim milk for 2 h at room temperature, were incubated with primary antibodies overnight at 4°C. And then the membranes were washed with Tris-buffered saline with Tween (TBST) buffer. Secondary antibody was utilized to incubate the membranes at room temperature for 1 h. The results were visualized by Pierce ECL Western Blotting Substrate kit for enhanced chemiluminescence (ECL). Antibodies are listed in Table S3.
Luciferase assay
Luciferase reporter plasmids of wild type and mutant lncR-APDC were constructed by GENECFPS, China, Jiangsu. Site mutations in lncR-APDC were confirmed by using MicroInspector online software (http://bioinfo.uni-plovdiv.bg/microinspector) (). Both fragments were further confirmed by digestion of the plasmids with EcoRI-XhoI. All genetic material was sequenced to confirm the correct DNA orientation and sequence. BMSCs co-transfected with the pmirGLO Vector constructs, miRNA oligos used lipofectamine 3000 reagents (Thermo Fisher Scientific, MA, USA) for 24 h treatment. Following the transfection, harvested cells were analysed for luciferase activity with the Dual-Glo® Luciferase Assay System (Promega). For each transfection, luciferase activity was averaged from five individual replicates.
Histomorphometric and immunohistochemical staining of bone and adipose tissues [Citation33]
For histological examination, tissue samples (7 samples for each group) were fixed in 10% formalin. Hard tissues were decalcified in 10% ethylenediaminetetraacetic acid (pH 7.0) for 2–3 weeks followed with dehydration in ethanol and embedded in paraffin wax. Sections were cut and mounted on glass slides with a thickness of 5 μm. Three random slides from each sample were stained with haematoxylin and eosin. Immunohistochemical staining (IHC) was performed to identify osteocalcin (OCN) (primary antibody: ab93876, Abcam, 1:250) expression using a Histostain SP kit (Life Technologies). H&E and IHC staining were photographed with an OLYMPUS B×53 microscope. To prevent the introduction of examiner bias, every slide was coded.
Statistical analysis
All results were expressed as means ± SD of three or more independent experiments. We used one-way analysis of variance and multivariate analysis of variance with Tukey post hoc test to test statistics significance via the software GraphPad Prism 9.1.2.
Results
lncR-ANRIL and lncR-APDC upregulation during osteogenesis
Enhanced ANRIL expression was evident in hFOBs during osteogenesis (). We harvested mBMSCs and mBMMs from C57/BL6 mice. The mBMSCs were induced to osteogenic differentiation and adipogenic differentiation. mBMMS were induced to osteoclastogenesis. mRNA expression of lncR-APDC and representative gene markers of osteogenesis, adipogenesis and osteoclastogenesis were analysed via qPCR. We found that the expression of lncR-APDC in BMSCs increased significantly during the early stages of osteogenesis, contrastingly decreasing during adipogenesis and osteoclastogenesis ( and Supplementary Figure S2). For the first time, we can predict that lncR-APDC has an impact on regulating stem cell and osteoclast differentiation, particularly on the processes involved in bone remodelling.
Role of lncR-APDC in regulating stem cell differentiation and osteoclast differentiation
Deletion of lncR-APDC impaired osteogenesis while promoting adipogenesis and osteoclastogenesis
To investigate the role of lncR-APDC in BMSCs, OBs and BMMs, APDC-KO mice obtained from MMRRC were bred as described previously [Citation12].
Genotyping results demonstrated successful deletion of lncR-APDC in the KO mice (). Mice were categorized into two distinct groups: APDC-KO and WT. Excessive proliferation of BMSCs in the APDC-KO group was overserved (S ). Isolated BMSCs were cultured with osteogenesis- or adipogenesis-inducing media. In vitro studies included qPCR, Alizarin Red staining (ARS) and oil red O staining. Osteogenic, adipogenic and osteoclastogenic marker gene ALP, Osx, Runx2, Ocn, Bmp-2, C/EBPα, Ucp-1, Zic-1, Mmp-9 and cathepsin K (CatK) (primers found in Table S2) expression were compared between the WT and APDC-KO groups. Reduced osteogenesis and increased adipogenesis of BMSCs were demonstrated in the APDC-KO group by qPCR and staining analysis. The heightened UCP1 expression in the APDC-KO group demonstrates enhanced energy expenditure and mitochondrial activity of adipocytes and precursor adipocytes. A reduction in osteogenesis was observed in the APDC-KO group. Deletion of lncR-APDC enhanced osteoclastogenesis of BMMs as the expression of CatK was upregulated and TRAP-positive multinucleated cells (MNCs) were increased ().
Figure 2. a. PCR of mouse tail DNA showing the genotyping of the APDC knockout: homozygotes (-/-), heterozygotes (±) and wild type (+/+). b. PCR verifying the lncR-APDC overexpression plasmid constructed successfully. c. The differentiation mRNA expression, ARS, oil red O staining and trap staining results were presented to describe the effects in osteogenesis, adipogenesis and osteoclastogenesis which was related to deletion of lncR-APDC. d. The differentiation mRNA expression, ARS, oil red O staining and trap staining results were presented to describe the effects in osteogenesis, adipogenesis and osteoclastogenesis which was related to overexpression of lncR-APDC. (All results were normalized using control group and statistically analysed via GraphPad prism 9.1.2.).
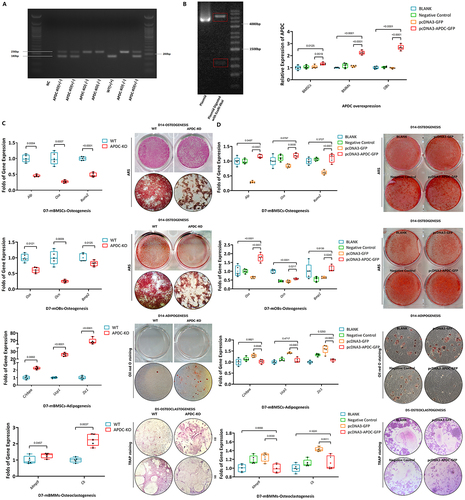
Overexpression of lncR-APDC promoted osteogenesis while inhibiting adipogenesis and osteoclastogenesis
Overexpression of lncR-APDC obtained by plasmid transfection constructed via pcDNA3.1 cloning vector (GENECFPS, Jiangsu, China), was verified by DNA electrophoresis and qPCR in BMSCs, OBs and BMMs (). The same comparisons as above were made between the BLANK, negative control, pcDNA3-GFP and pcDNA3-APDC-GFP groups. In vitro, the upregulation of osteogenesis of BMSCs and OBs was observed with overexpression of lncR-APDC compared with the control group. Adipogenesis was impaired in BMSCs with the overexpression of lncR-APDC. Overexpression of lncR-APDC effectively inhibited osteoclastogenesis of BMMs. ()
Impaired bone formation, altered expression of adiponectin & receptors in APDC-KO mice
With OCN IHC staining, a notable reduced Ocn expression was observed in metaphyseal trabecular bone in the APDC-KO group of 8-week-old mice (), which was similar to the results of 4-week-old (Supplementary Figure S3). Depending the reduced OCN expression within metaphyseal trabecular bone in the APDC-KO group, the reduced osteogenesis could be corroborated, especially in endochondral osteogenesis. mRNA expression of osteogenetic markers in bone tissues of APDC-KO and WT mice was analysed by qPCR (). Phenotypic differences include downregulated osteogenetic markers (Alp, Osx, Runx2, and Bsp1) and reduced bone mineralization in the APDC-KO group. Upregulation of RANKL also occurred in the APDC-KO group, indicating the activated osteoclasogenesis or reduced bone modelling. These results suggest that deletion of lncR-APDC leads to overall decreased bone formation. The mRNA expression of adiponectin receptors 1/2 (AdipoR1/R2) and genes associated with adiponectin (Apn) in the bone of both APDC-KO and WT mice are presented in . Increased Pparγ, Appl1 and Appl2 appeared in the bones of the APDC-KO group compared to WT group. The expression of AdipoR1 was downregulated in the APDC-KO group, while the expression of AdipoR2 was upregulated. Based on the evidences aforementioned, adipose metabolism was promoted, even the inflammatory state of bone tissue.
Figure 3. a. The histological HE-stained and OCN IHC staining femurs of 8-week-group were presented under different magnifications. b. The mRNA expression of bone tissue from WT/APDC-KO mice were analysed via RT-RCR. c. Expression of TLR4 and MyD88 in BMMs from WT/APDC-KO mice. d. Expression of OPG, TLR4 and MyD88 in osteogenesis-induced BMSCs from WT/APDC-KO mice. e. Expression of p38, p-p38 and TLR4 in osteoclastogenesis-induced BMMs from WT/APDC-KO mice. (All results were normalized using WT group and statistically analysed via GraphPad prism 9.1.2.).
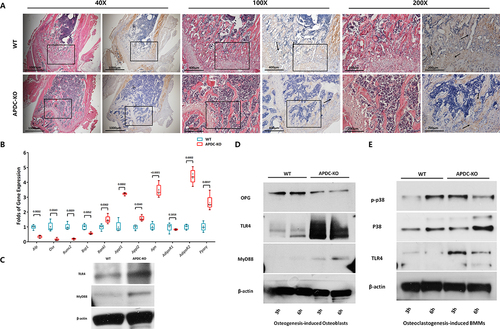
No evident variations in bone mass and microstructure of bone trabecula appeared around distal metaphysis in femurs with the HE staining, which was similar to the results of 8-week-old. While the reduced Ocn expression was observed in metaphyseal trabecular bone in APDC-KO group of 4-week-old with OCN IHC staining.
To investigate signalling pathways involved with lncR-APDC in bone marrow (BM), markers of different signalling pathways were analysed by western blot. It is noteworthy that lncR-APDC could be related to inflammatory states and metabolic disorders, which could cause osteoclastic-osteogenic imbalance [Citation24–27]. According to this, activated TLR4/MyD88 signalling in BM is observed in lncR-APDC KO mice (). Different signal pathways were investigated in osteogenesis-induced BMSCs and osteoclastogenesis-induced BMMs. A time-dependent increase in the expression of TLR4 and MyD88 was observed in BMSCs of APDC-KO group compared to WT group (). This implies the involvement of lncR-APDC in modulating these signalling molecules, potentially affecting the inflammatory response. Phosphorylation of p38 appeared earlier in BMMS from APDC-KO group compared to WT group (), which indicates that lncR-APDC might participate in the activation of the mitogen-activated protein kinase (MAPK) signal pathway through p-p38 in osteoclastogenesis.
We observed that APDC-KO mice were generally bigger in size and heavier in weight compared to WT mice (). This led us to further examine the WAT distribution and the histomorphology in these mice. Our investigation revealed that adipocytes in WAT in the inguinal region showed increased size in lncR-APDC KO mice (). We also focused on the changes of gene expression of WAT in 8-week-old male APDC-KO mice. With qPCR analysis, deletion of lncR-APDC enhanced adipogenic markers (C/EBPα, Ucp1, and Pparγ) in WAT, while molecules related to Apn were upregulated as well, such as Appl1/2 (). However, the upregulation of AdipoR1/R2 expression was not obvious, and there was no statistical significance. These results consistent with in vitro findings demonstrate that lncR-APDC suppresses adipogenesis and obesity.
Figure 4. a. The body type and weight of male mice was shown. B. The histological HE-stained wat from inguinal region was presented under different magnifications. c. The mRNA expression of WAT from WT/APDC-KO mice were analysed via RT-RCR. (All results were normalized using WT group and statistically analysed via GraphPad prism 9.1.2.).
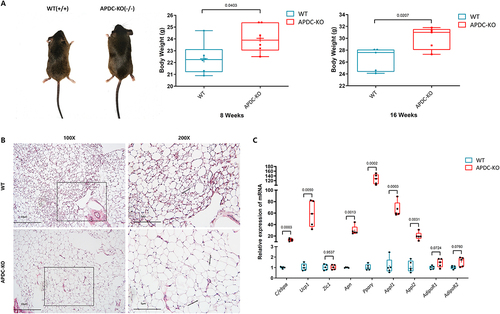
lncR-APDC affects osteogenesis through miR-99a/KDM6B
Our results revealed that, KDM6B was downregulated with the absence of lncR-APDC from BM cells. On the contrary, with overexpression of lncR-APDC, KDM6B was upregulated (). In our previous research, we discovered that KDM6B is a target of miR-99a, and is also specifically regulated by miR-99a during osteogenic differentiation [Citation30]. We theorized that miR-99a might be a potential binding site of lncR-APDC. While miR-99a was upregulated with the absence of lncR-APDC, downregulated miR-99a was observed with overexpression of lncR-APDC (), which suggests an intricate interplay between lncR-APDC, miR-99a, and KDM6B, pointing towards their potential involvement in osteogenic differentiation processes.
Figure 5. A. KDM6B expression in WT/APDC-KO mice analysed via RT-RCR. B. Analysed KDM6B expression in BMSCs with overexpression of lncR-APDC via RT-RCR. C. The miR99a expression in WT/APDC-KO mice. D. Analysed miR99a expression in BMSCs with overexpression of lncR-APDC via RT-RCR. E. The prediction of binding site between lncR-APDC and miR-99a using MicroInspector online software (http://bioinfo.uni-plovdiv.bg/microinspector). F. Wild-type and mutant lncR-APDC inserted into a luciferase vector, were transfected into BMSCs, which were cotransfected with either control or miR-99a mimic. miR-99a effectively suppressed luciferase reporter activity. G. lncR-APDC could regulate osteogenesis by targeting miR99a, which can regulate osteogenic differentiation through KDM6. (All results were normalized using negative control group and statistically analyzed via GraphPad prism 9.1.2.).
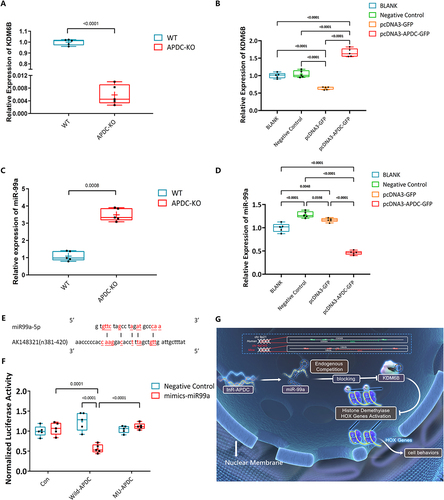
Using MicroInspector online software to predict the miRNA target site, lncR-APDC contains a single element complementary to miR-99a-5p (a putative target site located between n381 and n420 of lncR-APDC) (). This indicates that the functional inhibition of miR-99a may be induced by binding lncR-APDC, which was proved by luciferase assay followed. The mutant lncR-APDC (GENECFPS, Jiangsu, China) and wild type lncR-APDC inserted in Luciferase Vector were utilized in a luciferase assay to investigate whether miR-99a was a potential binding target of lnc-APDC. These vectors were transfected into BMSCs, while either control or miR-99a mimics co-transfected. The miR-99a mimics significantly suppressed luciferase activity, whereas mutated lncR-APDC effectively reversed this repression (), suggesting that miR-99a is a direct binding target of lnc-APDC ().
Discussion
ANRIL (CDKN2BAS) has been confirmed as a genetic risk factor for atherosclerosis, periodontitis, diabetes, and cancer, which represent complicated biological functions and mechanisms [Citation7–16,Citation20]. ANRIL knockout resulted in the repression of three genes ADIPOR1, VAMP3, and C11ORF10, associated with increased risk of CAD and PD [Citation34]. As konown, ANRIL regulates inflammatory responses by influencing glucose and fatty acid metabolism as well as the immune response [Citation13,Citation17,Citation34]. LncR-APDC, an ortholog of lncR-ANRIL, is hypothesized to have similar biological functions to ANRIL, including important regulatory mechanisms in bone and adipose tissue metabolism [Citation22,Citation23]. LncR-APDC and ANRIL exhibit the same promotion of osteogenesis in both mBMSCs and hFOBs. We report significant upregulation of lncR-APDC in the early stages of osteogenesis, with subsequent downregulation during adipogenesis and osteoclastogenesis. The chr4 Δ70kb/70kb mouse, in which lncR-APDC is severely reduced, has been utilized as a model for exploring phenotype, biological functions and mechanisms, studying the regulating features of lncR-APDC during the development and vascular diseases [Citation31]. With kinds of primary cells from APDC-KO mice, decreased presence of lncR-APDC leads to diminished osteogenesis by BMSCs and OBs and enhanced adipogenesis of BMSCs and osteoclastogenesis of BMMs. On the contrary, overexpression of lncR-APDC inhibits the adipogenic and osteoclastogenic lineages. Our findings with APDC-KO mice suggest strong actions of lncR-APDC on osteogenesis, adipogenesis, and osteoclastogenesis.
In the absence of lncR-APDC, osteogenic markers are remarkably downregulated, and significant inhibition of osteogenesis was observed in bone tissue of femurs. Except that, the more obvious OCN downregulation was especially observed in alveolar bone in APDC-KO group (S ). These results demonstrate the role of lncR-APDC in bone metabolism. With our displayed results, lncR-APDC is involved in the activation of MAPK signal pathways through p-p38 during osteoclastogenesis. As previously reported, MAPK/p-38 and nuclear factor kappa B (NFκB) are involved downstream of MCSF and RANKL inducing osteoclast differentiation and survival via cAMP response-element binding protein (CREB) phosphorylation [Citation35–37]. Furthermore, decreased presence of lncR-APDC activates TLR4/MyD88 signalling of osteoclasto-osteogenic balance, especially during the initial stage of osteoclastic activation. Osteoclasto-osteogenic imbalance caused by inflammatory state or metabolic disorders is involved with the high expression of TLR4. Notably, inhibition of TLR4/MyD88/NFκB-related signalings can inhibit osteoclastic activation and osteogenic destruction through different pathways [Citation28,Citation29,Citation38,Citation39]. These indicate that osteoclasto-osteogenic imbalance can be mediated with lncR-APDC by activated MAPK/p38 and TLR4/MyD88.
Based on prior research, the regulatory mechanism categories of lncRNAs can be divided into interfering with the translation of an encoded gene, binding to functional proteins or chromosomes, facilitating post-transcriptional modification or precursor substance of small molecule RNA [Citation2–4], especially functioning as a competing endogenous RNA (ceRNA), competitively binding to the corresponding miRNA response elements (MRE) [Citation5,Citation6,Citation40]. With the lncR-APDC deficiency, the downregulated KDM6B and upregulated miR-99a have been validated, while overexpression of lncR-APDC leads to the converse effect – downregulation of miR-99a and upregulation of KDM6B. The KDM6B gene has been confirmed as a target of miR-99a, which could affect the biological function of HOX genes, especially osteogenic differentiation of BMSCs [Citation30]. According to our previous research [Citation30], we supposed that miR-99a might be a potential binding site of lncR-APDC. Due to MicroInspector online software (http://bioinfo.uni-plovdiv.bg/microinspector), the miRNA target site was predicted within lncR-APDC, containing a single element complementary (a putative target site located between n381 and n420 of lncR-APDC) to miR-99a-5p. This indicates that the functional inhibition of miR-99a could be induced by binding lncR-APDC. Upon completion of a luciferase assay, miR-99a was identified as the direct binding target of lncR-APDC. Importantly, this investigation marks the first instance of identifying the influence of osteogenic function by miR-99a through regulating the KDM6B levels. We infer that bone metabolism regulation is regulated through the lncR-APDC/miR-99a/KDM6B/Hox/Runx2 pathway.
Adipokines, the cell-signalling molecules secreted by adipose tissue, play functional roles in the crosstalk between bone and adipose tissue. In this study, we determined the enhancement of adipogenesis in the lncR-APDC deficiency mouse model. The gene expression of adiponectin (APN), AdipoRs, and APPLs was upregulated in WAT of APDC-KO mice, which indicates that the local adipogenesis and function of APN were enhanced. For the first time, we demonstrated the positive correlation between lncR-APDC and APN/APN receptors. APN is a major adipokine with bone anabolic actions [Citation41–43]. This correlation has vast potential for pharmacological treatment of bone metabolism diseases, especially diabetes. The research reported that adipogenesis could be inhibited by paracrine-secreted APN, while endocrine-secreted APN could decrease the levels of circulating bone-activating hormones [Citation44]. Increased APN expression in lncR-APDC deficiency might lead to the increased biological function of endocrine-secreted APN, thereby enhancing adipogenesis and diminishing osteogenesis in BM. It’s important to acknowledge, however, there are still controversial opinions with function of APN to regulate bone metabolism. The role of APN from different sources needs to be further explored in our lncR-APDC deficiency and overexpression models in the future.
Recent studies suggested that excessive accumulation of marrow adipocytes, caused by BMSCs, plays a key role in ageing-related bone loss [Citation45,Citation46]. In this study, the gain- and loss- of lncRNA-APDC models demonstrate that lncRNA-APDC modulates both the bone remodelling and the metabolic process of adipocytes. These indicate that lncRNA-APDC may be involved in the transdifferentiation of osteoblasts and adipocytes, and has great potential preventive and/or therapeutic effects on bone and fat metabolic diseases, especially ageing-related bone loss.
Conclusion
lncR-APDC represents an pivotal regulatory element in bone and adipose tissue metabolism. The deletion of lncR-APDC impaired osteogenesis, while promoting adipogenesis and osteoclastogenesis. Conversely, the overexpression of lncR-APDC stimulates osteogenesis but inhibits adipogenesis and osteoclastogenesis. LncR-APDC, targeting miR-99a, triggers an enhancement of osteogenesis through miR-99a/KDM6B/Hox/Runx2 pathways. It also can inhibit osteoclastogenesis through MAPK/p-38 and TLR4/MyD88 signalling pathways, while regulating adipogenesis via APN-related pathways. These findings highlight the pivotal role of lncR-APDC as a key regulator in bone and fat tissue metabolism. It shows potential therapeutic for addressing inbalances in osteogenesis, adipogenesis, and osteoclastogenesis.
Contributions
Jake Jinkun Chen, En Luo, Yao Liu and Xiaofang Zhu designed the study. Yao Liu, Xiaofang Zhu, Elissa K. Zboinski, Wei Qiu, Junxiang Lian, Shibo Liu conducted the study and collected data. Yao Liu, Xiaofang Zhu and Qisheng Tu analysed the data. Jake Jinkun Chen, Yao Liu, and Qisheng Tu, interpretated the data. Jake Jinkun Chen Yao Liu and Xiaofang Zhu wrote the manuscript. Thomas E. Van Dyke and Hans E. Johansson reviewed and revised the manuscript.
Ethics approval statement
All the in vivo work in this study followed guidelines established by Tufts University and was approved by the Institutional Animal Care and Use Committee (IACUC) and the National Institutes of Health (NIH). (IACUC approval #B2020–02)
Supplemental Material
Download MS Word (1.2 MB)Supplemental Material
Download MS Word (1.2 MB)Disclosure statement
No potential conflict of interest was reported by the author(s).
Data availability statement
All data that support the findings of this study are available from the corresponding authors upon reasonable request.
Supplementary material
Supplemental data for this article can be accessed online at https://doi.org/10.1080/15476286.2023.2268489.
Additional information
Funding
References
- Guttman M, Amit I, Garber M, et al. Chromatin signature reveals over a thousand highly conserved large non-coding RNAs in mammals. Nature. 2009;458(7235):223–227. doi: 10.1038/nature07672
- Geisler S, Coller J. RNA in unexpected places: long non-coding RNA functions in diverse cellular contexts. Nat Rev Mol Cell Biol. 2013;14(11):699–712. doi: 10.1038/nrm3679
- Kung JT, Colognori D, Lee JT. Long noncoding RNAs: past, present, and future. Genetics. 2013;193(3):651–669. doi: 10.1534/genetics.112.146704
- Ponting CP, Oliver PL, Reik W. Evolution and functions of long noncoding RNAs. Cell. 2009;136(4):629–641. doi: 10.1016/j.cell.2009.02.006
- Salmena L, Poliseno L, Tay Y, et al. A ceRNA hypothesis: the Rosetta Stone of a hidden RNA language? Cell. 2011;146(3):353–358. doi: 10.1016/j.cell.2011.07.014
- Tay Y, Kats L, Salmena L, et al. Coding-independent regulation of the tumor suppressor PTEN by competing endogenous mRnas. Cell. 2011;147(2):344–357. doi: 10.1016/j.cell.2011.09.029
- Helgadottir A, Thorleifsson G, Manolescu A, et al. A common variant on chromosome 9p21 affects the risk of myocardial infarction. Science. 2007;316(5830):1491–1493. doi: 10.1126/science.1142842
- McPherson R, Pertsemlidis A, Kavaslar N, et al. A common allele on chromosome 9 associated with coronary heart disease. Science. 2007;316(5830):1488–1491. doi: 10.1126/science.1142447
- Helgadottir A, Thorleifsson G, Magnusson KP, et al. The same sequence variant on 9p21 associates with myocardial infarction, abdominal aortic aneurysm and intracranial aneurysm. Nat Genet. 2008;40(2):217–224. doi: 10.1038/ng.72
- Schunkert H, Götz A, Braund P, et al. Repeated replication and a prospective meta-analysis of the association between chromosome 9p21.3 and coronary artery disease. Circulation. 2008;117(13):1675–1684. doi: 10.1161/CIRCULATIONAHA.107.730614
- Holdt LM, Beutner F, Scholz M, et al. ANRIL expression is associated with atherosclerosis risk at chromosome 9p21. Arterioscler Thromb Vasc Biol. 2010;30(3):620–627. doi: 10.1161/ATVBAHA.109.196832
- Harismendy O, Notani D, Song X, et al. 9p21 DNA variants associated with coronary artery disease impair interferon-γ signalling response. Nature. 2011;470(7333):264–268. doi: 10.1038/nature09753
- Schaefer AS, Richter GM, Dommisch H, et al. CDKN2BAS is associated with periodontitis in different European populations and is activated by bacterial infection. J Med Genet. 2011;48(1):38–47. doi: 10.1136/jmg.2010.078998
- Congrains A, Kamide K, Oguro R, et al. Genetic variants at the 9p21 locus contribute to atherosclerosis through modulation of ANRIL and CDKN2A/B. Atherosclerosis. 2012;220(2):449–455. doi: 10.1016/j.atherosclerosis.2011.11.017
- Deloukas P, Kanoni S, Willenborg C, et al. Large-scale association analysis identifies new risk loci for coronary artery disease. Nat Genet. 2013;45(1):25–33. doi: 10.1038/ng.2480
- Hannou SA, Wouters K, Paumelle R, et al. Functional genomics of the CDKN2A/B locus in cardiovascular and metabolic disease: what have we learned from GWASs? Trends in endocrinology and metabolism. TEM. 2015;26(4):176–184. doi: 10.1016/j.tem.2015.01.008
- Aarabi G, Zeller T, Heydecke G, et al. Roles of the Chr.9p21.3 ANRIL locus in regulating inflammation and implications for anti-inflammatory drug target identification. Front Cardiovasc Med. 2018;5:47. doi: 10.3389/fcvm.2018.00047
- Cho H, Li Y, Archacki S, et al. Splice variants of lncRNA RNA ANRIL exert opposing effects on endothelial cell activities associated with coronary artery disease. RNA Biol. 2020;17(10):1391–1401. doi: 10.1080/15476286.2020.1771519
- Chen S, Zhong H, Wang Y, et al. The clinical significance of long non-coding RNA ANRIL level in diabetic retinopathy. Acta Diabetol. 2020;57(4):409–418. doi: 10.1007/s00592-019-01442-2
- Zhang B, Wang D, Ji TF, et al. Overexpression of lncRNA ANRIL up-regulates VEGF expression and promotes angiogenesis of diabetes mellitus combined with cerebral infarction by activating NF-κB signaling pathway in a rat model. Oncotarget. 2017;8(10):17347–17359. doi: 10.18632/oncotarget.14468
- Kong Y, Hsieh CH, Alonso LC. ANRIL: a lncRNA at the CDKN2A/B locus with Roles in cancer and metabolic disease. Front Endocrinol. 2018;9:405. doi: 10.3389/fendo.2018.00405
- Karolchik D, Kuhn RM, Baertsch R, et al. The UCSC genome browser database: 2008 update. Nucleic Acids Res. 2008;36(Database):D773–9. doi: 10.1093/nar/gkm966
- Visel A, Zhu Y, May D, et al. Targeted deletion of the 9p21 non-coding coronary artery disease risk interval in mice. Nature. 2010;464(7287):409–412. doi: 10.1038/nature08801
- Wan J, Bao Y, Hou LJ, et al. lncRNA ANRIL accelerates wound healing in diabetic foot ulcers via modulating HIF1A/VEGFA signaling through interacting with FUS. J Gene Med. 2023;25(2):e3462. doi: 10.1002/jgm.3462
- Kong Y, Sharma RB, Nwosu BU, et al. Islet biology, the CDKN2A/B locus and type 2 diabetes risk. Diabetologia. 2016;59(8):1579–1593. doi: 10.1007/s00125-016-3967-7
- Biswas S, Coyle A, Chen S, et al. Expressions of serum lncRnas in diabetic Retinopathy - a potential diagnostic tool. Front Endocrinol. 2022;13:851967. doi: 10.3389/fendo.2022.851967
- Loos BG, Van Dyke TE. The role of inflammation and genetics in periodontal disease. Periodontol. 2000;83(1):26–39. doi: 10.1111/prd.12297
- Liu Z, He Y, Xu C, et al. The role of PHF8 and TLR4 in osteogenic differentiation of periodontal ligament cells in inflammatory environment. J Periodontol. 2021;92(7):1049–1059. doi: 10.1002/JPER.20-0285
- Zeng XZ, Zhang YY, Yang Q, et al. Artesunate attenuates LPS-induced osteoclastogenesis by suppressing TLR4/TRAF6 and PLCγ1-Ca2+-NFATc1 signaling pathway. Acta Pharmacol Sin. 2020;41(2):229–236. doi: 10.1038/s41401-019-0289-6
- Tang Y, Zhang L, Tu T, et al. MicroRNA-99a is a novel regulator of KDM6B-mediated osteogenic differentiation of BMSCs. J Cell Mol Med. 2018;22(4):2162–2176. doi: 10.1111/jcmm.13490
- Zheng Y, Devitt C, Liu J, et al. A distant, cis-acting enhancer drives induction of arf by Tgfβ in the developing eye. Dev Biol. 2013;380(1):49–57. doi: 10.1016/j.ydbio.2013.05.003
- Lian J, Wu X, Liu Y, et al. Potential roles of miR-335-5p on pathogenesis of experimental periodontitis. J Periodontal Res. 2020;55(2):191–198. doi: 10.1111/jre.12701
- Qiu W, Wu H, Hu Z, et al. Identification and characterization of a novel adiponectin receptor agonist adipo anti-inflammation agonist and its anti-inflammatory effects in vitro and in vivo. Br J Pharmacol. 2021;178(2):280–297. doi: 10.1111/bph.15277
- Bochenek G, Häsler R, El Mokhtari NE, et al. The large non-coding RNA ANRIL, which is associated with atherosclerosis, periodontitis and several forms of cancer, regulates ADIPOR1, VAMP3 and C11ORF10. Hum Mol Genet. 2013;22(22):4516–4527. doi: 10.1093/hmg/ddt299
- Kim JH, Kim K, Kim I, et al. BCAP promotes osteoclast differentiation through regulation of the p38-dependent CREB signaling pathway. Bone. 2018;107:188–195.
- Koga Y, Tsurumaki H, Aoki-Saito H, et al. Roles of cyclic AMP response element binding activation in the ERK1/2 and p38 MAPK signalling pathway in central nervous system, cardiovascular system, osteoclast differentiation and mucin and cytokine production. Int J Mol Sci. 2019;20(6):20. doi: 10.3390/ijms20061346
- Lee K, Seo I, Choi MH, et al. Roles of mitogen-activated protein kinases in osteoclast Biology. Int J Mol Sci. 2018;19(10):3004. doi: 10.3390/ijms19103004
- Tao X, Qi Y, Xu L, et al. Dioscin reduces ovariectomy-induced bone loss by enhancing osteoblastogenesis and inhibiting osteoclastogenesis. Pharmacol Res. 2016;108:90–101. doi: 10.1016/j.phrs.2016.05.003
- Wu X, Qiao S, Wang W, et al. Melatonin prevents peri‑implantitis via suppression of TLR4/NF-κB. Acta Biomaterialia. 2021;134:325–336. doi: 10.1016/j.actbio.2021.07.017
- Karreth FA, Tay Y, Perna D, et al. In vivo identification of tumor- suppressive PTEN ceRnas in an oncogenic BRAF-induced mouse model of melanoma. Cell. 2011;147(2):382–395. doi: 10.1016/j.cell.2011.09.032
- Yu L, Tu Q, Han Q, et al. Adiponectin regulates bone marrow mesenchymal stem cell niche through a unique signal transduction pathway: an approach for treating bone disease in diabetes. Stem Cells (Dayton, Ohio). 2015;33(1):240–252. doi: 10.1002/stem.1844
- Wang Y, Du Y, Yuan H, et al. Human amnion-derived mesenchymal stem cells enhance the osteogenic differentiation of human adipose-derived stem cells by promoting adiponectin excretion via the APPL1-ERK1/2 signaling pathway. IUBMB Life. 2020;72(2):296–304. doi: 10.1002/iub.2165
- Wu X, Qiu W, Hu Z, et al. An adiponectin receptor agonist reduces type 2 diabetic periodontitis. J Dent Res. 2019;98(3):313–321. doi: 10.1177/0022034518818449
- Yang S, Liu H, Liu Y, et al. Effect of adiponectin secreted from adipose-derived stem cells on bone-fat balance and bone defect healing. J Tissue Eng Regen Med. 2019;13(11):2055–2066. doi: 10.1002/term.2915
- Salmi A, Quacquarelli F, Chauveau C, et al. An integrative bioinformatics approach to decipher adipocyte-induced transdifferentiation of osteoblast. Genomics. 2022;114(4):110422. doi: 10.1016/j.ygeno.2022.110422
- Zhang L, Fu X, Ni L, et al. Hedgehog signaling controls bone homeostasis by regulating osteogenic/adipogenic fate of skeletal stem/Progenitor cells in mice. J Bone Miner Res. 2022;37(3):559–576. doi: 10.1002/jbmr.4485