Abstract
After one exposure of C3H/HeJ pregnant females (at mid-gestation) to B(α) P (at 150 μ g/g BW), their progeny evidenced suppression of both humoral and cell-mediated immunity, as well as quantitative deficiencies in the levels of Lyt1+ and Lyt2+cells, and CD4+ CD8+, Vγ 3+, Vγ δ+, and Vα β+T-cells. We hypothesized that these conditions could be a result, in part, of covalent binding of BPDE to DNA within these cells. To test this, antiserum to BPDE-DNA was generated in rabbits; after multiple purification steps, an anti-BPDE-DNA (rendered ≈99.5% specific for BPDE-DNA and did not react with free BPDE or DNA antigens at dilutions even of < 1:50) antiserum was isolated. Flow cytometry and immunofluorescence analyses showed the adduct was present in CD4+ cells of progeny fetal and in both post-natal thymus and spleen tissues. Using a [32P]-post-labelling method, adduct was also detected in samples of fetal liver during the period from Day 15 to 18 gestation. Surprisingly, it was found that thymus cells from B(α)P-exposed mice not exhibiting the adduct could severely suppress allogeneic mixed lymphocyte responses, while those in which the adduct was detected caused had a more pronounced suppression. We suspect from the findings here that the presence of BPDE-DNA adducts in T-cells contributes to, but is not necessarily the causa sola for, the immunosuppression that develops in the offspring of pregnant mothers who are exposed to B(α)P (among many other agents) via smoking, ingestion, or inhalation of environmental pollution.
INTRODUCTION
It has been known for many years (i.e., see reviews from Searle, Citation1984 through Feng et al., Citation2007) that a major carcinogenic constituent of coal tar, soot, coal pyrolysis, coke oven residues, and petro-products (e.g., engine exhaust) is benzo(α) pyrene [B(α) P]. Like other members of the polycyclic aromatic hydrocarbon (PAH) family, B(α) P is a ubiquitous pollutant in atmospheres of various industrial and commercial environments. Domestic B(α) P exposure also arises due to its generation in a variety of sources, including cigarette smoking and combustion of wood and other organic substrates for heating and cooking (Li et al., Citation2003; Kuo et al., Citation2006; Lu and Zhu, Citation2007). In several Third World nations, it is known that pregnant women or infants held in their mothers' arms are routinely repeatedly exposed from 3–6 hr/day to high amounts (equal to or greater than those of coke oven or foundry workers) of B(α) P in the smoke generated from burning biomass during cooking (Smith et al., Citation1983; deKoning et al., 1985; reviewed in Naeher et al., Citation2007). Even certain cooking methods can lead to an increased presence of B(α) P on consumed foodstuffs themselves (reviewed in Simko, Citation2005). As a result, both adults and children are potentially extensively [non-occupationally] exposed each day to this dangerous carcinogen.
Like many PAHs, B(α) P exerts its carcinogenic activity through binding to genomic DNA. Most of the current theories of chemical carcinogenesis involving initiation, promotion, and progression, interrelated with metabolic oxygenation and the formation of reactive metabolites, are applicable to B(α) P. Specifically, B(α) P is metabolized via the cytochrome P450 system (collectively termed aryl hydrocarbon hydroxylase [AHH]) to a variety of reactive derivatives such as dihydrodiol epoxides (reviewed in Luch, Citation2005). More precisely, the initial step in B(α) P metabolism is its oxygenation by the CYP1A1 and/or CYP1B1 mixed function oxidases into arene oxides (see Luch and Baird, Citation2005). The latter then undergo enzymatic hydrolysis via the activity of epoxide hydrolase (EH) so as to yield trans-dihydrodiols; these, in turn, are then oxidized by CYP enzymes into dihydrodiol-epoxides. The latter cause somatic cell damage since the intermediate binds with DNA to form covalent adducts—a key event in chemical carcinogenesis (reviewed in Baird et al., Citation2005). For B(α) P, the reactive metabolites (+)- and (−)-(B(α) P-7,8-dihydrodiol-9,10-epoxides (BPDE) have been deemed the ultimate carcinogens formed during this metabolism (see Luch and Baird, Citation2005) as their covalent binding to DNA results in possible base changes and DNA rearrangements (Stevenson, Citation1990; Luch, Citation2005).
The BPDE metabolite adducts to the DNA (and cellular proteins) of many cell types, including peripheral blood lymphocytes, monocytes, and macrophages (Ng et al., Citation1998; Phillips, Citation2002, Citation2005; van Grevenynghe et al., Citation2003, Citation2004; Tochigi et al., Citation2005). The presence of these adducts in these cell types has been documented in occupationally-exposed individuals (i.e., see examples of Haugen et al., Citation1986; Santella et al., Citation1993) and in smokers (extensively reviewed in Phillips, Citation2002, Citation2005). For example, coke oven workers exposed to 1–4 or 7–9 μg B(α) P/m3 air (values dependent on use of air-filtering masks; Haugen et al., Citation1986), and foundry workers exposed to < 5, 5–12, or 12–60 μ g B(α) P/m3 air (Santella et al., Citation1993), were shown to bear BPDE-DNA adducts in their white blood cells at levels that corresponded to the amounts of B(α) P available and/or length of exposure at a given concentration. Among the foundry workers, average levels of 5.2, 6.1, and 9.6 adducts/108 nucleotides were detected in blood samples taken from members in the 3 respective exposure groups. Among the oven workers, a mere 3-wk respite from daily exposure led to a near-complete disappearance of the adduct presence (i.e., from > 13.7 down to 0.15 fmol/μ g DNA) in lymphocytes. Because of this good correlation between exposure (dose/time) and adduct levels, the presence of BPDE-DNA adducts within various circulating immune cells has been increasingly accepted as a good biomarker for B(α) P exposure in general (Reddy and Randerath, Citation1990; Castanzo-Vinyals et al., Citation2004; Perera et al., Citation2004; Luch and Baird, Citation2005; Shantakumar et al., Citation2005). It is important to note that exposure to B(α) P need not only occur by inhalation or ingestion to attain these same outcomes in immune system (or other type) cells. As shown by several other investigators, injection(s) of the starting B(α) P leads to formation of BPDE that covalently binds to DNA and proteins (Bolognesi et al., Citation1985, Baird et al., Citation2005; Luch, Citation2005).
Malmgren et al. (Citation1952) were the first to show the immunosuppressive action of PAHs. Other investigators have since demonstrated both carcinogenic and immunosuppressive effects of PAHs (including B(α) P) in adult animals, including mice. Of even greater significance were findings that exposure to B(α) P during fetal life induced a more severe carcinogenic effect than when it was introduced into adults (Bulay and Wattenberg, Citation1971; Urso and Gengozian, Citation1980). Several investigators have reported that a presence of epoxide hydrolase (EH) in fetal liver and other tissues (e.g., Pacifici and Rane, Citation1983; Rouet et al., Citation1983, Citation1984; Borlakoglu et al., Citation1993) as well as in various cell types (e.g., endothelial and dendritic cells, monocytes and macrophages) might, along with AHH (Uziel et al., Citation1985; Neubert and Tapken, Citation1988), also be mediating the biotransformation of B(α) P in the progeny exposed in utero.
Building upon the findings of the increased carcinogenic effect of B(α) P in progeny that were exposed in utero, Urso and Gengozian (Citation1982, Citation1984) then showed that there was also a greater sensitivity to immune suppression in these progeny. More precisely, these offspring evinced both increased severities of suppression of humoral immunity and increased deficiencies in host T-cell-mediated immunity. In later reports (Urso and Johnson, Citation1987; Urso et al., Citation1992, Citation2007), it was also demonstrated that there were quantitative changes and qualitative dysfunction within T-cell subsets and that some of their significant molecules may have been involved in altered positive and negative selection and signal transduction events. Furthermore, it was shown that B(α) P rendered T-cells, such as CD5+ (Lyt1+), CD8+ (Lyt2+) (Urso and Johnson, Citation1987) and the T-cell receptor (TCR) molecule-bearing Vγ 3+, Vγ δ+, Vα β+, and CD4+ (L3T4+) cells, defective in the progeny. More recent studies have attempted to correlate these changes with those in fetal liver BPDE levels, and in turn, with increased tumorigenesis in these progeny (Urso et al., Citation2007).
The work here was prompted by preliminary studies from this laboratory on the detection of BPDE-DNA adducts in lymphoid tissues of B(α) P-exposed progeny after intraperitoneal (IP) injection of dams on Day 12 of pregnancy (see Moolenaar et al., Citation1996; Urso et al., Citation1996). It has been well documented that B(α) P given to a dam at mid-gestation can enter the fetus where it is then metabolized to BPDE that is toxic not only to fetal lymphocytes, but also to cells (e.g., dendritic, epithelial, macrophages) of the fetal liver and thymus (Santella et al., Citation1992; Ng et al., Citation1998; Shultz et al., Citation2001). Our intentions here were two-fold, i.e., to show that in mice exposed to B(α) P in utero: (1) BPDE adducts to the DNA of T-cells of the thymus and spleen, as well as of cells in the fetal liver—events that could impact on the total numbers of T-cells available for maintaining immunocompetency; and, (2) BPDE adduction to the DNA of a variety of thymic (immature and mature) cells can reduce cross-talk between developing CD4+CD8+ double positive (DP) T-cells and Class II (or Class I) stromal cells, resulting in functionally immuno-incompetent T-cells among any survivors of positive or negative selection.
MATERIALS AND METHODS
Animals and Treatments
C3H/HeJ mice (5–6-weeks-old, Jackson Laboratories, Bar Harbor, ME) were separated by gender and housed 5 mice per cage (until 11–12 weeks-of-age), and provided food and water ad libitum. Sets of 2 females were then housed with one male, and the females were observed daily for vaginal plugs. When a plug was noted (Day 0 of gestation), the female was housed by itself (> 90% of plugged females become pregnant). At Day 12 of gestation, the female was injected intraperitoneally (IP) with 150 μ g B(α) P (Aldrich Chemical Company, Milwaukee, WI) per gram body weight (BW).
While it may appear that 150 μ g/g BW has little clinical relevance, this dose was chosen based on its wide use in numerous other investigations examining effects of B(α) P in the progeny of exposed dams (i.e., Lummus and Henningsen, Citation1995; Rodriguez et al., Citation1999, Citation2002). Interestingly, some other investigators have used much higher doses (Shum et al., Citation1979; Mattison et al., Citation1980; Wang and Lu, Citation1990; Holladay and Smith, Citation1994), and others slightly or much lower ones (i.e., Welch et al., Citation1972; Adler, Citation1983; Legraverend et al., Citation1984; Bolognesi et al., Citation1985; Ross et al., Citation1991; Lu et al., Citation1993) to successfully induce expression of BPDE-DNA adducts in various tissues of different species, including mice. As such, the dose used here can be characterized as mid-range in comparison to these other studies.
Isolation and Preparation of Cells from Progeny
Fetuses were removed for use at Days 15 to 18 of gestation; offspring were also used at 1 wk post-birth. Thymus, spleen, and fetal liver cells were then isolated and prepared for use in various assays as described by Urso et al. (Citation1987, Citation2007). The Day 18 thymuses were pooled from 3–5 pups, while 2 thymuses were pooled from the 1-week-old pups and kept on ice in a petri dish containing phosphate-buffered saline (PBS) until use. Two spleens from post-natal progeny were pooled, as were 2 fetal livers from mice collected at Days 15 to 18 gestation. At all times, care was taken to prevent blood spillage from the surrounding thymus. If blood contamination did occur, the thymus cells were layered over Ficoll–Hypaque (F-H; specific gravity = 1.089), as were the spleen and fetal liver cells. After centrifugation, the buffy coat (interface) was recovered and kept on ice until use. It was noted that cell loss after F-H separation was minimal relative to that encountered if red blood cell lysing media was employed.
Preparation of BPDE-DNA and Generation of Anti-BPDE-DNA Antibody
Normal DNA was isolated from pooled thymuses of 6-week-old naïve C3H/HeJ mice following protocols provided in a non-organic extraction kit (Oncor, Gaithersburg, MD). After teasing the organs apart, liberated thymus cells were placed in 9 ml wash solution, incubated 15 min at 25°C, and then centrifuged (1000 × g, 20 min, 25°C). Ethanol (2 vol) was added to the recovered supernatant. After the DNA present precipitated it was spooled on a Pasteur pipette, air-dried, dipped in 70% ethanol, and air-dried again. The DNA was placed in buffer and incubated at 50°C overnight. After vortexing, the DNA (now in solution) was diluted 1:50 with buffer and total DNA content then quantified spectrophotometrically.
To allow for the reaction of BPDE with DNA, the harvested DNA (at 2 mg/ml) was first mixed with an equal volume of acetone. One mg BPDE (anti-7,8-dihydrodiol-9,10-epoxide; IIT Research Institute, Chicago, IL) in 1 ml ethanol was then combined with 1 ml DNA:acetone solution (i.e., 1 mg DNA), and the mixture incubated 18 hr at 37°C in the absence of light. Unbound BPDE was then removed and the nucleic acids precipitated with 0.1 vol 2 M sodium acetate and 2.5 vol ethanol. After overnight incubation at −20°C, precipitate was recovered by centrifugation at 5000 RPM (≈3350 × g) and dissolved in 0.1 ml distilled water. Adduct presence was then determined (post-acid hydrolysis; Vahakangas et al., Citation1988)) by synchronous fluores-cence spectrophotometry (SFS; emission at 300 nm, excitation at 337 nm), with peak adduct detection expected from 347–382 nm. Increased detection of tetraol moieties (B(α) P tetraols acid-liberated from parent DNA gave a peak spanning a 355–375 nm emission () corresponded to increases in levels of adduction between BPDE and DNA (Vahakangas et al., Citation1988). According to these Authors, SFS is likely more specific for BPDE-DNA adducts than [32P]-post-labelling and ultra-sensitive enzyme radioimmunoassay (USERIA).
FIG. 1 Synchronous fluorescence spectrophotometry for determining adduction of BPDE to DNA. Result of one representative experiment is shown. Maximum excitation wavelengths for tetraols are 349 to 352 nm, and the wavelength remains high at 355 to 375 nm.
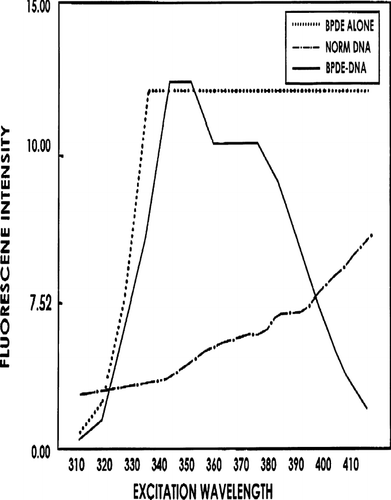
In preparation for immunizing, BPDE-DNA was dissolved in 0.1 M NaCl, and the DNA then denatured by boiling and then rapidly chilling in water. One percent (1%) methylated BSA (in water) was then added until the final weight ratio was 1.0. This complex was emulsified in Titermax (Sigma, St. Louis, MO) and then injected into White New Zealand rabbits (≈2 kg; Charles River, Wilmington, MA) to raise anti-BPDE-DNA antibody-bearing serum. Specifically, equal volumes were injected intramuscularly into the right and left gastrocnemius, and subcu-taneously into the left and right inguinal regions. Ten to 14 days post-immunization, 10–35 ml blood was collected from the marginal ear vein of each rabbit. After clotting, the serum was removed, centrifuged at 2000 RPM (≈550 × g) for 30 min in the cold, and then stored at −70°C until assayed for the anti-BPDE-DNA antibody.
Precipitation and Purification of Rabbit Anti-BPDE-DNA Antibody
To detect antibody against carcinogen-DNA adducts, the antiserum was precipitated with 30–40% ammonium sulfate (1:1 v/v) and the subsequent precipitate then dissolved in water (yielding mostly rabbit IgG). The solution containing IgG (protein in serum was 85–90% globulin rich) was then added to an EZ-Sep polyclonal antibody purification kit (Sigma). Antibody protein levels in the final resolved product were determined spectrophotometrically at 280 nm. If any further purification of antibody was necessary, BPDE was coupled with cyanogen bromide-activated Sepharose 6B gel and column chromatography was performed. In all cases, column eluates were tested for presence of anti-BPDE-DNA IgG by enzyme-linked immuno-sorbent assay (ELISA). An ELISA kit was purchased from Kirkgaard and Perry Laboratories (Gaithersburg, MD) and the procedures used were those recommended by the manufacturer. Mouse serum antigen (MSA), BSA, BPDE, DNA, or BPDE-DNA were placed in designated triplicate wells in the test plate and served as potential antigens for antibodies in each eluate. Preliminary studies already showed that the rabbit anti-BPDE-DNA did not cross-react with MSA, and only very weakly with BSA.
[32P]-Post-Labelling Procedure to Detect BPDE-DNA
A slight modification of the procedure of Reddy and Randerath (Citation1990) was used. Briefly, mouse DNA was isolated, and then 50 μ g samples (in a 1 ml total volume) were then digested at 37°C for 5 hr with 16 U microccocal mononuclease (1 U/ml) and 16 μ g spleen phosphodiester-ase (1 μ g/ml). The mixture was then added to 30 μ g P1 nuclease and incubated 45 min; it was then labeled for 30 min at 37°C with 3.5 U T4 polynucleotide kinase and 50 μ Ci [32P]-ATP (Amersham, Arlington Heights, IL). The material was then applied to a PEI-cellulose thin layer chromatography plate to separate adducted nucleotides. The plate was sequentially developed, then placed with X-ray film to localize adducts. Confirmation of the location of the specific BDPE-DNA adducts on the plate was based on comparison to results with a plate spotted with BPDE-DNA adduct standard (prepared from the same material used to generate antibodies [see above]) and then processed in parallel. Specific spots corresponding to those on the film were removed from the plate, mixed with scintillation cocktail, and analyzed (in terms of cpm in adducted nucleotides and relative adduct levels [RAL]).
Magnetic-Activated Cell Sorting (MACS)
This procedure followed the manufacturer's instructions (Miltenyi Biotec, Auburn, CA); specifics of the MACS protocol, as well as the monoclonal antibodies used herein have recently been outlined in detail in Urso et al. (Citation2007).
Immunofluorescent Staining and Flow Cytometry
As an example of one series of studies, 3–4 ml of thymus cells (at 1–50 × 106/ml) were layered over an equal volume of F-H and centrifuged at 3500 RPM (≈1650 × g) and 20°C for 20 min (note: thymus cells not contaminated with red blood cells were not treated with F-H). Interface cells were then combined with rat or mouse anti-CD4 monoclonal antibodies (Pharmingen, San Diego, CA) and incubated in the cold for ≈15 min. After gentle washing with PBS, the cells were then treated with fluorescein isothiocyanate (FITC)- or phycoerythrin (PE)-conjugated) goat anti-rat or FITC- or PE-rat anti-mouse antibody. The mixture was again washed gently with PBS to remove unbound antibody and the cells counted. Dilution of the cell preparation was necessary when the count was suspected of being > 105–106.
After the last incubation in the staining, the cells were washed twice, resuspended in 0.5 ml PBS, and an aliquot removed and immediately placed in a Becton Dickinson (BD) flow cytometer for analysis. The results obtained with the BD system were further compared against those from a Coulter Flow Cytometer in the Flow Cytometry Laboratory in The Winship Cancer Center at Emory University. Overall, the data obtained from both systems (for matched samples) were consistently highly similar. Cell viabilities were verified by forward-angle scatter and exclusion of trypan blue. In all samples, viability of cells was found to be > 90%. In each analysis, a total of 10,000 sample events were obtained to generate the data used for analyses.
Functional Capacity of B(α) P-Exposed Thymus Cells
The ability of CD4+ thymus cells (C3H/HeJ B(α) P-exposed responder thymic cells bearing BPDE-DNA adduct) to proliferate in the presence of stimulator cells was assayed by the allogeneic mixed-lymphocyte response (MLR) as outlined previously (Urso et al., Citation1992, Citation2007). The thymic cells contained CD4+CD8− surface molecules and had been isolated using mini-macs column (beads conjugated with rat anti-mouse CD4) after initial removal of CD8-bearing cells via MACS. To further select for adduct-containing cells from both sets of progeny, saponin was added to the CD4+ SP cells followed by rabbit anti-BPDE-DNA and goat anti-rabbit IgG FITC. Using flow cytometry, fluorescent (i.e., CD4+BPDE-DNA+) and non-fluorescent (i.e., CD4+BPDE-DNA−) cells were segregated and isolated, and these responder cells were then assayed for proliferative capacity in the MLR.
Briefly, allogeneic stimulator spleen cells [(Balb/c × DBA/2) F1] were first inactivated with 1000 Rads of γ -radiation. Triplicate samples of thymic cells from 1-week-of-age B(α) P-exposed or corn oil-exposed control (CO) progeny were then placed [at 107/ml] in RPMI-1640 containing 5% fetal calf serum and then added to designated wells along with an equal volume and number of allogeneic splenic cells (Balb/cXDBA/2) F1. After 72 hr incubation, cultures received 0.5 μ Ci [3H]-thymidine (DuPont Chemicals, Wilmington, DE) and cells were recovered 24 hr later on glass fiber filters using a Skatron harvester. The filters were placed in vials, scintillation cocktail was added, and the vials incubated at 37°C overnight. Radioactivity in each sample was then counted in a Beckman LS 5801 scintillation counter (Fullerton, CA) and proliferation expressed in terms of counts/min (CPM).
Statistics
Tests for significance were determined by Student's t-test for small samples of equal or unequal size.
RESULTS
General Observations on Progeny
Though the actual data is not included in this report, as repeatedly observed in earlier studies (i.e., Urso and Gengozian Citation1982; Wolisi et al., Citation2001) that employed identical regimens to those used here, fetal viability, litter size, and pup survival at birth from B(α) P-exposed pregnant dams were not significantly different from that seen with the dams (or their progeny) in the corn oil-treatment groups. Furthermore, as also seen earlier (Urso and Johnson, Citation1987), exposure of the pregnant dams to the 150 μ g B(α) P/g dose led to a significant decrease in body weight as well as in the thymus, spleen, and liver weights of progeny on post-natal days 3 though 5; fetal parameters were not significantly altered over the period of gestation day 15 to birth. Thereafter, the status of all experimental and control offspring appeared normal (until about 9 mo post-partum when the B(α) P-exposed progeny began to suffer the same increased mortality as noted in our earlier studies; see Urso and Gengozian, Citation1984).
Detection of BPDE-DNA
Following addition of the rabbit anti-serum to the EZ-Sep polyclonal rabbit purification kit, the recovered antibody in PBS was tested against BPDE, BPDE-DNA-BSA, BPDE-DNA, BSA, and DNA antigens at a 1:25 dilution. The rabbit antiserum reacted with all antigens (there was a very low reaction against BSA [data not shown]). Since it was further necessary to purify the rabbit anti-BPDE-DNA antiserum, immunoaffinity purification columns were employed. The adherent antiserum of this column reacted against DNA, BPDE, and BPDE-DNA, BSA, or MSA at a dilution of 1:100 or less. On the other hand, the antiserum eluant containing rabbit anti-BPDE-DNA reacted well against BPDE-DNA at 1:100 and lower dilutions (i.e., dilutions > 1:1000), but did not react with the other antigens, and most importantly, against either BPDE or DNA alone (data not shown). This strongly indicated that the eluant containing rabbit anti-BPDE-DNA was specific for BPDE-DNA, although it may cross-react with DNA adducts formed by other B[a]P metabolites that could not be examined in the present study.
Detection of BPDE-DNA in CD4+CD8+ Thymic and Spleen Cells
Thymus cells of Day 18 gestation and 1-week-old progeny were applied to MACS containing rat ant-mouse CD4; the cells recovered from the beads (i.e., CD4+CD8+ DP [double positive] and CD4+CD8− SP [single positive] cells) were then permeabilized with saponin and incubated with rabbit anti-BPDE-DNA antibody. After blocking with non-cross-reactive rat anti-Fc antibody, the cells were mixed with goat anti-rabbit IgG FITC (green) antibody and propidium iodide (PI, to stain DNA red) and analyzed by flow cytometry.
Among the thymus cells of 1-week-old progeny, BPDE-DNA was shown to be present in the CD4+CD8+ DP and/or CD4+CD8− SP cells that localized to Quadrant 2 (18%) as green [anti-adduct] and red (DNA) dual fluorescence (). In control progeny samples (), as expected, BPDE-DNA was not detected. This is borne out by comparisons of their Quadrant 2 values to those seen in that reflected levels of dual fluorescing cells after binding of a non-specific 1° antibody (i.e., also reflects background non-specific binding of 2° antibody in the reaction system). Although the values were not the same, the Quadrant 2 data for the Day 18 fetal thymus was comparable to that of the thymus of a 1-week-old pup (data not shown). As expected, neither cell population displayed significant shifts beyond Quadrant 3 (analogous to double negative cells) in the absence of primary/secondary antibodies ( and ).
FIG. 2 Detection of BPDE-DNA adducts in MACS-isolated CD4+CD8+ DP and CD4+ CD8− SP cells from thymus of B(α) P- and corn oil (CO)-exposed progeny (1-wk-of-age). Data shown are representative of 4 experiments. X-axis: Intensity of Green Fluorescence. Y-axis: Intensity of Red Fluorescence. Results presented are from: (A) CO thy cells only; (B) B(α) P thy cells only; (C) CO cells + propidium iodide (PI) + rabbit anti-BPDE-DNA 1° Ab + FITC-goat anti-rabbit IgG 2° Ab; (D) B(α) P cells + PI + 1° Ab + 2° Ab; and (E) B(α) P thy cells + PI + non-specific rabbit IgG + 2° Ab (to reflect non-specific binding of 2° Ab). Numbers in Quadrant 2 are percentage of cells detected containing BPDE-DNA adducts. For example, in , 18% of cells stained with PI (DNA-red) and rabbit anti-BPDE-DNA FITC (green), indicating presence of BPDE-DNA in progeny cells.
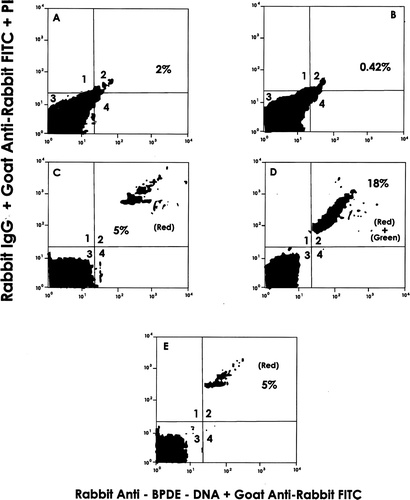
As the data in was intriguing, double immunofluorescence studies were under-taken with spleen cells of the 1-week-old mice. Again, cells were passed through MACS containing rat anti-mouse CD8. The effluent containing (among many cells present) putative CD4+CD8− SP cells was then applied to a mini-macs column containing biotinylated rat ant-mouse CD4 and strepavidin. Bead-associated cells recovered were subjected to double staining, (i.e., first treated with rat anti-mouse CD8 followed by PE-conjugated goat anti-rat Ig, then with rat anti-mouse CD4 followed by FITC-goat anti-rat Ig) and then analyzed by flow cytometry.
Repeated experiments indicated that > 97% of the MACS effluent cells were CD4+CD8− (data not shown). The recovered CD4+CD8− cells were mixed with saponin and PI, and then with rabbit anti-BPDE-DNA IgG and FITC-goat anti-rabbit IgG, before the mixture was subjected to flow cytometry. As before, the data in , Quadrant 2, represents the dual fluorescence obtained from the PI (for DNA) and FITC (for BPDE), indicating that these CD4+CD8− SP cells expressed BPDE-DNA (a portion of these adduct-bearing cells may also express γ δ –see Urso et al., Citation2007).
Presence of BPDE-DNA in Fetal Liver as Determined by [32P]-Post-Labelling
Preliminary reports on the detection of BPDE-DNA in fetal liver by a [32P]-post-labelling method were given by Moolenaar et al. (Citation1996) and Urso et al. (Citation1996). Here, CD4+CD8− fetal liver cells were isolated as described above for spleen cells. Although BPDE-DNA adduct was evident in livers of B(α) P-exposed progeny as early as Day 15 of gestation (data not shown), maximal levels (determined based on total radioactivity and relative adduct levels) were reached on Day 17 (i.e., 10 × 108 nucleotides; ). Furthermore, BPDE-DNA analysis showed a strong spot in the same region as the 17-day gestation fetal liver (data not shown). In livers of fetuses from dams that had been exposed to corn oil, no adduct was present at these time points. In each case, that the adduct isolated and analyzed was attributable to BPDE rather than by other B[α]P metabolites was based upon the localization of the adduct to the same spot as that with a BPDE-DNA adduct standard (data not shown).
Functional Assay of CD4+ BPDE-DNA Adduct-Bearing Thymus Cells
The allogeneic MLR was used to assay functionality of the responder thymic cells from B(α) P-exposed progeny. Cells that contained (i.e., BPDE-DNA+) or lacked (i.e., BPDE-DNA−) adduct each evinced very poor proliferative responses (16 and 22% of control mouse cell levels, respectively) (). However, the response by BPDE-DNA+ cells was significantly (p < 0.001) worse than that by the BPDE-DNA− cells. The mean values obtained were 5.70 × 103 (±0.14, SD) and 3.90 × 103 (±0.06) for the BPDE-DNA− and BPDE-DNA+ cells, respectively. Thus, the presence of the BPDE-DNA adduct appeared to have exerted some influence on an already subnormal MLR in cells from these exposed progeny. These results with BPDE-DNA− cells bolster earlier observations that thymic cells from B(α) P-exposed progeny are markedly disabled in supporting responses to allogeneic antigens (Urso and Gengozian, Citation1984; Citation1992; Citation2007).
FIG. 5 One-way allogeneic MLR by isolated SP CD4+ thymus cells that do or do not express the BPDE-DNA adduct. Values shown represent mean percent of corn oil mouse [control] MLR activity (± SD) from 4 experiments. Control thymus cell MLR CPM = 25 × 103. Difference between relative activities of BPDE-DNA+ and BPDE-DNA− cells was significant (p < 0.001).
![FIG. 5 One-way allogeneic MLR by isolated SP CD4+ thymus cells that do or do not express the BPDE-DNA adduct. Values shown represent mean percent of corn oil mouse [control] MLR activity (± SD) from 4 experiments. Control thymus cell MLR CPM = 25 × 103. Difference between relative activities of BPDE-DNA+ and BPDE-DNA− cells was significant (p < 0.001).](/cms/asset/3aa6d127-8c16-4e5f-ba4e-f2784ba1ff87/iimt_a_267861_uf0005_b.gif)
DISCUSSION
In this report, the presence of BPDE-DNA adducts in CD4+ (both CD4+CD8+ DP and CD4+CD8 − SP) cells of the post-natal thymus and spleen, as well as in cells (as yet, undefined) in the fetal liver, of progeny from B(α) P-exposed dams was demonstrated. These represent the first in vivo studies detecting the ultimate carcinogenic intermediate of B(α) P metabolism, BPDE, in specific subsets of T-cells from both of these lymphoid organs in exposed progeny. Although the fundamental mechanism underlying the immunosuppression seen here is still obscure, it seems clearer that the reactive intermediate BPDE plays an important—albeit not exclusive—role as previously suspected (White and Holsapple, 1983; Wojdani et al., 1983; Holladay and Smith, Citation1994; Holladay and Luster, Citation1996; Lee et al., Citation1996; Lee and Urso, Citation2007).
It has been long-postulated that the BPDE-DNA adduct is ultimately responsible for the genotoxicity (as well as carcinogenicity and teratogenicity) of B(α) P; as such, any genotoxic effects within lymphocytes and/or stromal cells (i.e., dendritic cells, macrophages/monocytes, epithelial cells) residing in lymphoid organs could be especially critical to the development of immunomodulation. Although fetal tissue can metabolize B(α) P into various products damaging to the developing immune system, metabolic activation can also occur in the dams. It is possible that the BPDE can cross the placenta into the fetus; BPDE has been detected in the placenta (Lu et al., Citation1993).
Based on finding of other investigators, it can also be inferred that maternal BPDE crosses into the fetus itself, covalently binds to fetal DNA, and, causes immune damage and other genotoxic injuries in the fetus (i.e., carcinogenic outcomes) (Lu et al., Citation1993). Even with no or limited BPDE transplacental transfer, it is already known that after injection into a dam, B(α) P crosses the placenta and enters the fetus wherein it is metabolized (via AHH/EH) to BPDE (Lu and Wang, Citation1990). It appears that the fetal liver represents a strong source of reactive metabolites (like BPDE) that could then initiate toxicities in the fetus (Pedersen et al., Citation1985; Blake et al., Citation1979; Lu and Wang, Citation1990). Stromal cells in the thymus and other tissues also have the AHH capability to generate BPDE; local lymphocytes, and perhaps their T-class components, in the fetus might also directly metabolize B(α) P through the AHH system (Kouri et al., Citation1982; Uziel et al., Citation1985; Haugen et al., Citation1986) to also generate the BPDE.
Although not specifically studied for this report, it is highly likely that macrophages (i.e., dendritic cells) also play a prominent role in the induction of the observed immunodeficiency. These ubiquitous cells, which include alveolar and peritoneal macrophages, liver Kupffer cells, and splenic macrophages (including other sites) (Ladics et al., Citation1992; Tochigi et al., Citation2005)—also contain the mixed function oxidase P450 enzymes CYP1A1 and CYP1B1 among other CYP compounds (Luch and Baird, Citation2005). Upon encountering B(α) P, these cells will metabolize the PAH into intermediate metabolites including BPDE (Dehnen, Citation1975; Ng et al., Citation1998; Shultz et al., Citation2001; van Grevenynghe et al., Citation2004) that is then, in turn, toxic to them. Therefore, even if DP cells were present and unaffected by the B(α) P or BPDE, the macrophages would likely be dysfunctional (or at least fewer in number), and thus positive and negative selection would be severely hampered.
Because stromal cells present in the newborn thymus and spleen are able to metabolize B(α) P to its reactive intermediates, even in the absence of any adducts or associated genetic modulation among lymphocytes there could be potential shifts induced in the normal immune function in the exposed progeny. For example, changes in the genomes of the various stromal cell types could potentially lead to abnormal maturation and differentiation, and changes in surface marker expression (Laupeze et al., Citation2002). These types of alterations could then lead to reduced interactions between major histocompatibility complex (MHC) molecules on dendritic cells/macrophages and TCR sites on the “normal” T-cells. Similarly, because development of immunological efficiency requires interactions between functional stromal cells and histocompatible CD4+CD8+DP T-cells to ultimately establish functional CD4+ (and CD8+) SP T-cells, the cross-talk for these events between both cell types could potentially become minimal or negligible if the stromal cells develop aberrant surface marker expression(s). Of course, if BPDE-DNA adducts are also present within one or more of the T-cell subtypes, then each of the scenarios above can occur with greater potential frequency (i.e., if both stromal and T-cells are affected) or even in the absence of any changes in any “unaffected” (i.e., adduct-deficient) macrophages/dendritic cells.
Another major significance of the findings here is that the fetogenic developing immune system was compromised by B(α) P. As noted in earlier studies by this and other laboratories, this effect lasts for almost the entire lifespan of the prenatally exposed mouse. Building upon earlier findings of others using B(α) P (or other PAHs; Holladay and Smith, Citation1994; Holladay and Luster, Citation1996; Holladay and Smialowicz, Citation2000), a presence of BPDE-DNA adducts in neonate lymphoid organ cells, as well as cells of the fetal liver, could suggest that this persistent immune deficiency likely arises from a failure to fully repair this initial damage to the immune system. Moreover, it seems that any repair of this damage may be of little consequence; B(α) P-exposed progeny remain immunodeficient for at least 18 mo and also develop tumors (beginning at 9 mo-of-age) that leads to mortality rates of > 80% (Urso and Gengozian, Citation1982, Citation1984). It is intriguing that these animals exposed in utero appeared normal for at least 9 mo post-birth. This initial finding of “normal” may have been more a product of the fact that the progeny were housed in extremely clean/aseptic conditions all their lives.
While a linkage between damage to lymphoid organ cells to development of immunodeficiencies in the progeny could appear to be somewhat straightforward, the relationship to this same damage in liver cells is less direct. Holladay and Smith (Citation1994) found a reduction in levels of pro-lymphocytic cells bearing CD44+and terminal deoxynucleotidyl transferase (TdT+) cells in GD18 fetal liver after in utero B(α) P exposure. Holladay and Luster (Citation1996) later noted studies (Gregoire et al., Citation1977) that showed that among the TdT+ cells in fetal liver were prothymocytes, the immediate precursors of thymocytes. The Authors also cited a study by Fine et al. (Citation1989) in mice exposed to TCDD at GD14 that suggested that a reduced fetal liver capacity to synthesize TdT could ultimately result in a failure to supply a developing thymus with prothymocyte stem cells. It would not then be implausible to surmise that a presence of BPDE-DNA adducts in fetal liver cells could cause impairments that would lead to a reduction of progenitor (prothymocyte) cells that could migrate to the developing thymus (i.e., pro-T-cells).
In conjunction with the potential changes in overall T-cell numbers induced by in utero B(α) P exposure, effects upon the processes involved in the normal development of those T-cells evolving in the thymus might also become a part of this immunomodulatory response. Although the precise abundance of the various forms of TCR molecules on CD4+ SP or CD4+CD8+ DP cells containing the BPDE-DNA adduct was not directly determined here, it is highly likely that at least some of these SP/DP not only contained the adduct but also bore TCR γ δ and/or α β markers (Urso et al., Citation2007). Whether there are shifts in the relative expression of either/both molecules overall in the exposed progeny thymic cells remains to be determined in our ongoing studies. These shifts could arise from effects induced by the presence of the adduct (i.e., base changes and aberrant gene rearrangements) upon the normal gene rearrangements required for fetogenic T-cell development and for positive and negative selection of T-cells in the thymus.
Normally, DP α β -bearing cells must successfully undergo positive and negative selection before they leave the thymus. Positive selection occurs when DP cells bind cortical epithelial cells expressing Class I (or II) MHC-self-peptide complexes with sufficiently high affinity to receive a cell survival signal. In turn, some cells that survive selection may migrate further into the cortico-medullary junction where they encounter (marrow-derived) macrophages and dendritic cells expressing MHC-self peptide complexes. Those cells that bind self peptide-MHC with high affinity at this stage undergo negative selection and die (by apoptosis). Of course, other cells that have successfully rearranged TCRα β but fail to bind MHC-self complexes at all within ≈ 4 days die in the cortex even prior to selection processes. As a result, any changes in expression of TCR molecules would ultimately mean that interactions between thymic stromal cells and DP/SP cells would be reduced. In these scenarios, thymic T-cell development would not progress adequately enough to supply functional immunoresponsive cells to, and there would also be increased levels of dysfunctional T-cell subsets in, the exposed host (Urso and Johnson, Citation1987; Urso et al., Citation2007).
Still, even with the results presented here regarding the disturbances in allogeneic MLR (in conjunction with results from some of our previous studies) with the cells from the B(α) P-exposed progeny, it cannot yet be firmly established that BPDE-DNA adducts are the key factors in the immunosuppression induced in progeny by B(α) P. For example, here, even BPDE-DNA− CD4+ SP cells gave rise to suppressed MLR. It may be that immunosuppression after B(α) P exposure includes deleterious effects by other intermediate reactive metabolites that do not bind to DNA yet have profound effects on the immune system (i.e., 3-OH-B(α) P and B(α) P-7,8-dihydrodiol; Lee et al., Citation1996; Lee and Urso, Citation2007).
In conclusion, this study documents the presence of BPDE-DNA adducts in CD4+CD8−SP and/or CD4+CD8+DP T-cells in the lymphoid organs of progeny exposed to B(α) P in utero. However, while a true cause-and-effect mechanism for BPDE relative to immunosuppression has not been fully established here, the effects of B(α) P strongly implicate this agent and its varied metabolites in impacting on the fetal ontogenesis of the immune system, ultimately leading to immunodeficiency in these progeny. Though the adducts may not be the key factors in the immunosuppressive effects induced by B(α) P, they could lead to genotoxic changes (such as gene rearrangement) that render immune cells (i.e., CD4+CD8+ and CD4+CD8− lymphocytes and/or macrophages) disabled in carrying out their interactions with histocompatible cells.
While the generalized immune deficiency that exists in the progeny may not completely depend on the effect of BPDE-DNA adducts, the latter's presence appears to exacerbate the already-altered functional capabilities of the T-cells. Ultimately, it is most likely that the greatest impact of dam exposure to B(α) P is two-fold, i.e., genetic damage is induced in their progeny that allows for the expression of neoplastic changes in the cells of various tissues and the cancer cells that are created are now no longer able to be recognized adequately (i.e., killed/removed) by the concurrently-disabled immune system.
This work was supported by grants from the Minority Access for Research Careers (MARC) Program, National Institutes of Health (to P.J.M-W.), The United States Agency for International Development (USAID) #CMB 00-0412-0519; # PCE-5053-G-00-3962, and from The United States Environmental Protection Agency (EPA) #R815813. We are indebted to Dr. Joy Zhu for assistance in the performance of the flow cytometry at The Morehouse School of Medicine, and to the Flow Cytometry Laboratory of The Winship Cancer Center at Emory University, Atlanta, GA. The experiments on the mice were done and approved according to the guidelines of The Morehouse School of Medicine, Animal Care Committee.
Current address for Pamela J. Moolenaar-Wirsiy is Georgia Perimeter College, Decatur, GA 30034. Current address for Yungri G. Wirsiy is Piedmont Hospital, Atlanta, GA 30309.
REFERENCES
- Adler I D. New approaches to mutagenicity studies in animals for carcinogenic and mutagenic agents. II. Clastogenic effects determined in transplacentally treated mouse embryos. Teratogen. Carcinogen. Mutagen. 1983; 3: 321–334
- Baird W. M., Hooven L. A., Mahadevan B. Carcinogenic polycyclic aromatic hydrocarbon-DNA adducts and mechanism of action. Environ. Mol. Mutagen. 2005; 45: 106–114
- Blake D. A., Martz F., Gery-Martz A., Gordon G. B., Mellits E. D. Fetal tissues from various strains of induced mice metabolize benzo(α) pyrene to mutagenic metabolites. Teratology 1979; 20: 377–382
- Bolognesi C., Rossi L., Barbieri O., Santi L. Benzo(α)pyrene-induced DNA damage in mouse fetal tissue. Carcinogenesis 1985; 6: 1091–1095
- Borlakoglu J. T., Scott A., Henderson C. J., Jenke H. J., Wolf C. R. Transplacental transfer of polychlorinated biphenyls induces simultaneously the expression of P450 isoenzymes and the protooncogenes c-Ha-ras and c-raf. Biochem. Pharmacol. 1993; 45: 1373–1386
- Bulay O. M., Wattenberg L. W. Carcinogenic effects of polycyclic hydrocarbon administration to mice during pregnancy on the progeny. J. Natl. Cancer Inst. 1971; 46: 397–402
- Castanzo-Vinyals G., D'Errico A., Malats N., Kogevinas M. Biomarkers of exposure to polycyclic aromatic hydrocarbons from environmental air pollution. Occup. Environ. Med. 2004; 61: e12, 1–16, (electronic review)
- Dehnen W. Studies on the metabolism of benzo(α)pyrene in alveolar macrophages. 1. Uptake of benzo(α)pyrene and induction of benzo(α)pyrene hydroxylase. Zentralbl. Bakteriol. 1975; 160: 197–201
- De Koning H. W., Smith K. R., Last J. M. Biomass fuel combustion and health. WHO Bull. 1985; 63: 11–26
- Feng Y., Shi G., Wu J., Wang Y., Zhu T., Dai S., Pei Y. Source analysis of particulate-phase polycyclic aromatic hydrocarbons in an urban atmosphere of a northern city in China. J. Air Waste Manage. Assoc. 2007; 57: 164–171
- Fine J. S., Gasiewicz T. A., Silverstone A. E. Lymphocyte stem cell alterations following perinatal exposure to 2,3,7,8-tetrachlorodibenzo-p-dioxin. Mol. Pharmacol. 1989; 35: 18–25
- Gregoire K. E., Goldschneider I., Barton R. W., Bollum F. J. Intracellular distribution of terminal deoxynucleotidyl transferase in rat bone marrow and thymus. Proc. Natl. Acad. Sci. USA 1977; 74: 3993–3996
- Haugen A., Becher G., Benestad C., Vahakangas K., Trivers G. E., Newman M. J., Harris C. C. Determination of polycyclic aromatic hydrocarbon in the urine, benzo(α)pyrene diol epoxide-DNA adducts in lymphocyte DNA, and antibodies to the adducts in sera from coke oven workers exposed to measured amounts of polycyclic aromatic hydrocarbons in the work atmosphere. Cancer Res. 1986; 46: 4178–4183
- Holladay S. D., Luster M. I. Alterations in fetal thymic and liver hematopoietic cells as indicators of exposure to developmental immunotoxicants. Environ. Health Perspect. 1996; 104(S4)809–813
- Holladay S. D., Smialowicz R. J. Development of the murine and human immune system: Differential effects of immunotoxicants depend on time of exposure. Environ. Health Perspect. 2000; 106(S3)463–473
- Holladay S. D., Smith B. J. Fetal hematopietic alterations after maternal exposure to benzo(α)pyrene: A cytometric evaluation. J. Toxicol. Environ. Health 1994; 42: 259–273
- Kouri R., McKinney C., Siomiany D., Snodgrass D., Wray N., McLemore T. Positive correlation between high aryl hydrocarbon hydroxylase activity and primary lung cancer as analyzed in cryopreserved lymphocytes. Cancer Res. 1982; 42: 5030–5037
- Kuo C. Y., Chang S. H., Chien Y. C., Chiang F. Y., Wei Y. C. Exposure to carcinogenic PAHs for the vendors of broiled food. J. Exp. Sci. Environ. Epidem. 2006; 16: 410–416
- Ladics G. S., Kawabata T. T., Munson A. E., White K. L. Evaluation of murine splenic cell type metabolism of benzo(α)pyrene and functionality in vitro following repeated in vivo exposure to benzo(α)pyrene. Toxicol. Appl. Pharmacol. 1992; 116: 258–266
- Laupeze B., Amiot L., Sparfel L., Le Ferric E., Fauchet R., Fardel O. Polycyclic aromatic hydrocarbons affect functional differentiation and maturation of human monocyte-derived dendritic cells. J. Immunol. 2002; 168: 2652–2658
- Lee M., Urso P. Suppression of spleen T lymphocyte proliferation to antigenic and mitogenic stimuli by metabolic pathways of benzo(α)pyrene and 2-aminofluorene. Immunopharm. Immunotox. 2007, (In Press)
- Lee M., Kirlin W., Stirrup, Rodriguez J., Urso P. Variation in the response of T-cells to Concanavalin A after in utero exposure to benzo(a)pyrene and 2-amino-fluorene. Immunopharm. Immunotox. 1996; 18: 309–321
- Legraverend C., Guenthner T. M., Nebert D. W. Importance of the route of administration for genetic differences in benzo[a]pyrene-induced in utero toxicity and teratogenicity. Teratology 1984; 29: 35–47
- Li C. T., Lin Y. C., Lee W. J., Tsai P. J. Emission of polycyclic aromatic hydrocarbons and their carcinogenic potencies from cooking sources to the urban atmosphere. Environ. Health Perspect. 2003; 111: 483–487
- Lu H., Zhu L. Pollution patterns of polycyclic aromatic hydrocarbons in tobacco smoke. J. Hazard. Mat. 2007; 139: 193–198
- Lu L. J., Anderson L. M., Jones A. B., Maskal T. J., Salazar J. J., Hokanson J. A., Rice T. M. Persistence, gestation-stage-dependent formation and interrelationship of benzo(a)pyrene-induced DNA adducts in mothers, placentae, and fetuses of Erythrocebus patas monkeys. Carcinogenesis 1993; 14: 1805–1813
- Lu L. J., Wang M. Y. Modulation of benzo(α)pyrene–induced covalent DNA modifications in adult and fetal mouse tissue by gestation stage. Carcinogenesis 1990; 11: 1367–1372
- Polycyclic aromatic hydrocarbon-induced carcinogenesis—An introduction. The Carcinogenic Effects of Polycyclic Aromatic Hydrocarbons, A. Luch. Imperial College Press, London 2005
- Luch A., Baird W. M. Metabolic activation and detoxification of polycyclic aromatic hydrocarbons. The Carcinogenic Effects of Polycyclic Aromatic Hydrocarbons, A. Luch. Imperial College Press, London 2005
- Lummus Z. L., Henningsen G. Modulation of T-cell ontogeny by transplacental benzo(a)pyrene. Int. J. Immunopharmacol. 1995; 17: 339–350
- Malmgren R. A., Bennison B. E., McKinley T. W., Jr. Reduced antibody titers in mice treated with carcinogenic and cancer therapeutic agents. J. Natl. Cancer Inst. 1952; 79: 484–488
- Mattison D. R., White N. B., Nightingale M. R. The effect of benzo(a)pyrene on fertility, primordial oocyte number, and ovarian response to pregnant mare's serum gonadotropin. Pediat. Pharmacol. 1980; 1: 143–151
- Moolenaar P. J., Wirsiy Y. G., Rodriguez J. W., Zhang W., Lee M., Urso P. Dysfunctional T-cells induced by benzo(α)pyrene express BPDE-DNA adducts. Proc. Am. Assoc. Cancer Res. 1996; 37: 446
- Naeher L. P., Brauer M., Lipsett M., Zelikoff J. T., Simpson C. D., Koenig J. Q., Smith K. R. Woodsmoke health effects: A review. Inhal. Toxicol. 2007; 19: 67–106
- Ng D., Kokol N., Hiura T., Faris M., Saxon A., Nel A. Macrophage activation by polyclic armatic hydrocarbons: Evidence for involvement of stress-activator protein-1, and antioxidant response elements. J. Immunol. 1998; 161: 942–951
- Neubert D., Tapken S. Prenatal induction of benzo(a)pyrene hydroxylases in mice. Arch. Toxicol. 1988; 62: 192–199
- Pacifici G. M., Rane A. Epoxide hydrolase in human fetal liver. Pharmacology 1983; 26: 241–248
- Pedersen R. A., Meneses J., Spindle A., Wu K., Gallaway S. M. Cytochrome P450 metabolic activity in embryonic and extraembryonic tissue lineages of mouse embryos. Proc. Natl. Acad. Sci. USA 1985; 82: 3311–3315
- Perera F. P., Tang D., Tu Y., Cruz L. A., Borjas M., Bernert T., Whyatt R. M. Biomarkers in maternal and newborn blood indicate heightened fetal susceptibility to procarcinogenic DNA damage. Environ. Health Perspect. 2004; 112: 1133–1136
- Phillips D. H. Smoking-related DNA and protein adducts in human tissues. Carcinogenesis 2002; 23: 1979–2004
- Phillips D. H. Macromolecular adducts as biomarkers of human exposure to polycyclic aromatic hydrocarbons. The Carcinogenic Effects of Polycyclic Aromatic Hydrocarbons, A. Luch. Imperial College Press, London 2005
- Reddy M. V., Randerath K. A comparison of DNA adduct formation in white blood cells and internal organs of mice exposed to benzo(a)pyrene, dibenzo(c,g)-carbazole, safrole, and cigarette smoke condensate. Mutat. Res. 1990; 241: 37–48
- Rodriguez J. W., Kirlin W. G., Wirsiy Y. G., Matheravidathu S., Hodge T. W., Urso P. Maternal exposure to benzo[a]pyrene alters development of T-lymphocytes in offspring. Immunopharmacol. Immunotoxicol. 1999; 21: 379–396
- Rodriguez J. W., Kohan M. J., King L. C., Kirlin W. G. Detection of DNA adducts in developing CD4+ CD8+ thymocytes and splenocytes following in utero exposure to benzo[a]pyrene. Immunopharmacol. Immunotoxicol. 2002; 24: 365–381
- Ross J., Nelson G., Erexson G., Kligerman A., Earley K., Gupta R. C., Nesnow S. DNA adducts in rat lung, liver, and peripheral blood lymphocytes produced by IP administration of benzo(α)pyrene metabolites and derivatives. Carcinogenesis 1991; 12: 1953–1955
- Rouet P., Dansette P., Frayssinet C. Endogenous induction of epoxide hydrolase, benzo(α)pyrene hydroxylase and glutathione-S-transferase in “responsive” C57Bl/6 mice and in “non-responsive” DBA/2 mice during pregnancy. Biochem. Biophys. Res. Commun. 1983; 112: 313–319
- Rouet P., Dansette P., Frayssinet C. Ontogeny of benzo(a)pyrene hydroxylase, epoxide hydrolase and glutathione-S transferase in the brain, lung and liver of C57Bl/6 mice. Devel. Pharmacol. Ther. 1984; 7: 245–258
- Santella R. M., Grinberg-Fines R. A., Young T. L., Dickey C., Singh V. N., Wang J. W., Perera F. A. Cigarette smoking-related polynucleotide aromatic hydrocarbons-DNA adducts in peripheral mononuclear cells. Carcinogenesis 1992; 13: 2041–2045
- Santella R. M., Hemminki K, Tang D. L., Paik M., Ottmean R., Yaing T. Polycyclic aromatic hydrocarbons-DNA adducts in white blood cells and urinary 1-hydroxypyrene in foundry workers. Cancer Epidemiol. Biomark. Prevent. 1993; 2: 59–62
- Searle C. E. Chemical Carcinogens. American Chemical Society, Washington, DC 1984; 51–501
- Shantakumar S., Gammon M. D., Eng S. M., Sagiv S. K., Gaudet M. M., Teitelbaum S. L., Britton J. A., Terry M. B., Paykin A., Young T. L., Wang L. W., Wang Q., Stellman S. D., Beyea J., Hatch M., Camann D., Prokopczyk B., Kabat G. C., Levin B., Neugut A. I., Santella R. M. Residential environmental exposures and other characteristics associated with detectable PAH-DNA adducts in peripheral mononuclear cells in a population-based sample of adult females. J. Exp. Anal. Environ. Epidem. 2005; 15: 482–490
- Shultz M. A., Movin D., Chang A.-M., Buckpitt A. Metabolic capabilities of CYP2F2 with various pulmonary toxicants and its relative abundance in mouse lung subcompartments. Pharmacology 2001; 2961: 510–519
- Shum S., Jensen N. M., Nebert D. W. The murine Ah locus: In utero toxicity and teratogenesis associated with genetic differences in benzo(α)pyrene metabolism. Teratology 1979; 20: 365–376
- Simko P. Factors affecting elimination of polycyclic aromatic hydrocarbons from smoked meat foods and liquid smoke flavorings. Mol. Nutr. Food Res. 2005; 49: 637–647
- Smith K. R., Aggarwal A. L., Dave R. M. Air pollution and rural biomass fuels in developing countries. A pilot village study in India and implications for research and policy. Atmos. Environ. 1983; 17: 2343–2362
- Mouse Liver Carcinogenesis. Mechanisms and Species Comparisons, D. Stevenson. Wiley-Liss, New York 1990
- Tochigi Y., Yamashiki N., Ohgiya S., Ganaka S., Yokota H. Isoform specific expression of UDP-glucuronosyl transferase immunoactivated peritoneal macrophages of the rat. Drug Metabol. Dispos. 2005; 33: 1391–1398
- Urso P., Gengozian N. Depressed humoral immunity and increased tumor incidence in mice following in utero exposure to benzo(α)pyrene. J. Toxicol. Environ. Health 1980; 6: 569–576
- Urso P., Gengozian N. Alteration in the humoral immune response and tumor frequencies in mice exposed to benzo(α)pyrene and X-rays before or after birth. J. Toxicol. Environ. Health 1982; 10: 817–835
- Urso P., Gengozian N. Subnormal expression of cell-mediated and humoral immune responses in progeny disposed toward a high incidence of tumors after in utero exposure to benzo(α)pyrene. J. Toxicol. Environ. Health 1984; 14: 569–584
- Urso P., Johnson R. A. Early changes in T-lymphocytes and subsets of mouse progeny defective as adults in controlling growth of a syngeneic tumor after in utero insult with benzo(α)pyrene. Immunopharmacology 1987; 14: 1–10
- Urso P., Moolenaar P. J., Wirsiy Y. G., Rodriguez J. W., Zhang W., Lee M. T-Cells contain BPDE-DNA adducts and become dysfunctional during fetal ontogeny. FASEB J. 1996; 10: A1047
- Urso P., Wirsiy Y. G., Zhang W., Moolenaar-Wirsiy P. J. Defective CD4+, CD8+, Vγ 3, Vγ δ, Vα β T cells and expression of B(α)P 7,8-diol-9,10-epoxide in lymphoid tissue of immunosuppressed progeny after in utero exposure to benzo(α)pyrene. J. Immunotoxicol. 2007, (Submitted)
- Urso P., Zhang W., Cobb J. R. Immunological consequences from exposure to benzo(a)pyrene during pregnancy. Scand. J. Immunol. 1992; 36(S11)203–206
- Uziel M., Griffin G. D., Walsh P. J. Aryl hydrocarbon hydroxylase tissue-specific activities: Evidence for baseline levels in mammalian tissues. J. Toxicol. Environ. Health 1985; 16: 727–742
- Vahakangas K., Pelkonen O., Harris C. C. Synchronous fluorescence spectro-photometry of benzo(α)pyrene diol epoxide-DNA adducts: A tool for detection of in vitro and in vivo DNA damage by exposure to benzo(α)pyrene. IARC Sci. Publ. 1988; 89: 208–212
- van Grevenynghe J., Rion S., LeFerree E., LeVee M., Amior L., Faucher R., Fardel O. Polycyclic aromatic hydrocarbons inhibit differentiation of human monocytes into macrophages. J. Immunol. 2003; 170: 2374–2381
- van Grevenynghe J., Snarfel L., LeVee M., Gilot D., Drenou B., Fauchel R., Fardel O. Cytochrome P450-dependent toxicity of environmental polycyclic aromatic hydrocarbons towards human macrophages. Biochem. Biophys. Res. Commun. 2004; 317: 708–716
- Wang M. Y., Lu L. J. Differential effect of gestation stage on benzo(a)pyrene-induced micronucleus formation and/or covalent DNA modification in mice. Cancer Res. 1990; 50: 2146–2151
- Welch R. M., Gommi B., Alvares A. P., Conney A. H. Effect of enzyme induction on the metabolism of benzo(a)pyrene and 3′-methyl-4-monomethylaminobenzene in the pregnant and fetal rat. Cancer Res. 1972; 32: 973–978
- White K. L., Jr., Holsapple M. P. Direct suppression of in vitro antibody production by mouse spleen cells by the carcinogen benzo(a)pyrene but not by the non-carcinogenic congener benzo(e)pyrene. Cancer Res. 1984; 44: 3388–3393
- Wojdani A., Alfred L. J. Alterations in cell-mediated immune functions induced in mouse splenic lymphocyte by polycyclic aromatic hydrocarbons. Cancer Res. 1984; 44: 942–948
- Wolisi G. O., Majekodunmi J., Bailey G. B., Urso P. Immunomodulation in progeny from thymectomized primiparous mice exposed to benzo(α)pyrene during mid-pregnancy. Immunopharm. Immunotox. 2001; 23: 267–281