Abstract
Microglia, the main immune effector cells in the central nervous system, play a dual role in the function/structure of the blood–brain barrier (BBB) and brain health. During and soon after a cerebral ischemic injury, microglia produce neurotrophic factors and neurotoxins that can impact on the injury itself and pathology progression. At the same time, microglia undergo polarization to M1 or M2 pro- vs. anti-inflammatory subtypes that also help drive the outcome of the injury process. Thus, agents that can mitigate cerebral ischemic injury progression, promote protective functions of microglia, and help maintain BBB and overall brain health/host neurologic function after a cerebral ischemic event would be of great use in clinical settings. Protective effects from gallic acid (GA) in cerebral ischemia/re-perfusion-induced injury to the BBB and other sites in the brain have not yet been assessed. To address this, a middle cerebral artery occlusion (MCAO) method was used to establish an experimental ischemic stroke model in mice. Mice were placed in sham operation (Sham), model (MCAO), MCAO + GA (50 mg/kg), MCAO + GA (100 mg/kg), or MCAO + GA (150 mg/kg) groups. At various times post-stroke, cerebral infarct volume and host neurological function were evaluated. In addition, qRT-PCR, Western blotting, and ELISA were used to evaluate the expression and tissue content of microglia-related factors. The results showed GA treatment protected the integrity of the BBB, significantly reduced brain edema, and helped lead to improved neurological function scores in the MCAO mice. Whether these changes were due to that GA attenuated cerebral ischemia/re-perfusion-induced activation of microglial cells overall, in part, by inhibiting their polarization to the M1 subtype, is uncertain. Taking these outcomes together, for now it is reasonable to suggest that use of GA either as a prophylactic or immediately in the event of a cerebral ischemic event/stroke could help to promote neuronal survival and allow for a more likely of host neurological function over time.
Introduction
Strokes occur at a high incidence, often resulting in a distressingly high level of disability and mortality. Ischemic strokes accounts for about 80–87% of all events, and the incidence of this is increasing yearly (Kim et al. Citation2016). It has been reported that the neural injury cascade in the acute phase of cerebral ischemia plays an important role in the preservation of the blood–brain barrier (BBB) function (Dirnagl et al. Citation1999; Emsley and Hopkins Citation2008). As the middle cerebral artery (MCA) is the area seen to be most affected during ischemic cerebrovascular disease, a rat [or mouse middle] cerebral artery occlusion (MCAO) model has been developed to simulate a human ischemic brain injury that leads to a complete blockage of blood supply in the area of responsibility (Sidney et al. Citation2013). Even with these new models, specific pathological mechanisms underlying ischemic stroke as yet remain unclear.
A variety of mechanisms are involved in the pathological processes associated with acute ischemic stroke. In the hyper-acute phase, BBB permeability increases and the central nervous system is infiltrated by peripheral immune cells (Orellana-Urzua et al. Citation2020). Local microglia are in turn (over)-activated and release a variety of inflammatory factors and chemokines, leading to enhanced inflammation and aggravation of any brain tissue damage (Akhtar and Kamal Citation2013). These activated microglia can secrete a large number of biologically-active factors such as tumor necrosis factor (TNF), interleukins (IL), interferon (IFN), and transforming growth factor (TGF) in an autocrine or paracrine manner (Song et al. Citation2019). As a result of this release, inflammatory immune cells are activated as well during the ischemic event (Willis et al. Citation2020). These inflammatory cells then aggravate local tissue damage through increased production/release of oxygen free radicals, cytotoxic enzymes, vasoactive amines, and chemokines that then recruit even more immune cells to the damaged tissue area (Karve et al. Citation2016).
Because of this complex cascade of events, monitoring the integrity of brain tissue and/or the activation of microglia could be used to assess potential therapeutic effects of any novel drugs/natural product proposed for use in the treatment of ischemic stroke (Roach et al. Citation2015). There have been several studies that have examined potential neuroprotective effects of a wide range of compounds – both synthetic and natural.
Gallic acid (GA) is a polyphenolic compound that can be fully absorbed in the body (and form 4-O-methyl gallate during metabolism) (Fernandes and Salgado Citation2016). In the context of the immune response, previous studies have indicated that GA can impart anti-allergy and anti-inflammatory effects in situ (Kahkeshani et al. Citation2019). Mechanistically, it has been shown that GA can regulate the polarization processes of M1-type macrophages and inhibit inflammatory responses in part by inhibiting M1-type polarization of macrophages. Furthermore, GA–chitosan complexes have been found to modulate inflammatory responses in lipopolysaccharide-stimulated RAW264.7 cells (macrophage line) by impacting on the cellular NF-κB, AP-1, and MAPK pathways (Ahn et al. Citation2016). Even with those studies showing the potential for GA to act as an immunomodulant, its potential utility for neuroprotection during severe acute and/or prolonged inflammatory events remains unclear.
In research on the central nervous system, Diaz et al. (Citation2020) reported that a 10 mg GA/kg (by intraperitoneal injection, once a day for 60 days) treatment regimen in rats with metabolic syndrome improved their recognition memory and helped to reduce oxidative-inflammatory damage in their hippocampus. Moreover, Mirshekar et al. (Citation2018) reported that GA imparted some neuroprotective in rats with traumatic brain injury. Thus, there are indications of the potential protective effect against neurologic damage from this natural product. To build upon that premise, in the study reported here, experiments were undertaken to provide data to examine whether GA could provide a protective effect against cerebral ischemia/re-perfusion-induced BBB inflammation-associated injuries in a mouse model. To evaluate any protective effects of GA on such inflammation-based injuries, a middle cerebral artery occlusion (MCAO) method was employed to induce cerebral ischemia/re-perfusion-induced BBB pathologies.
Materials and methods
Animals
C57BL/6J mice (male, 8-week-of-age, 25–27 g) were obtained from Shanghai Model Organisms (Shanghai, China) for use in this study. On arrival, all mice were housed in pathogen-free rooms maintained at 25–27 °C, with a 50–60% relative humidity, and a 12-h light:dark cycle. All mice had ad libitum access to sterilized standard food pellets and filtered tap water. All experimental protocols were approved by the Institutional Animal Care and Use Committee of the Liaocheng People's Hospital.
Study design
After 1 week of acclimatization, mice were randomly allocated into the various experimental groups described below (N = 40/treatment group). Gallic acid (GA) (purity ≥ 98%, Sigma, St Louis, MO) was dissolved in phosphate-buffered saline (PBS, pH 7.2) at fixed levels so that doses of 50, 100 or 150 mg GA/kg could be provided in identical volumes across groups. PBS was used as the negative control. In these studies, the GA or PBS was administered 30 min (end of ischemia, starting time of perfusion), 1 h (half an hour after perfusion), as well as 12, 24, 48, and 72 h after the MCAO modeling. In all cases, treatment was by intraperitoneal injection (IP); injection volume never exceeded 100 µl/mouse. The treatment groups here were: 1) Sham = sham-operated (MCAO–) and received normal saline (10 ml/kg [IP] once daily, IP); 2) MCAO = MCAO+ and received normal saline (10 ml/kg [IP] once daily) 30 min, as well as at 1, 12, 24, 48, and 72 h after MCAO modeling; and (3) GA + MCAO = MCAO+ and received GA at 50, 100 or 150 mg/kg (IP) using the same timepoints. All procedures were completed between 8:00 and 12:00 AM. At 3 d post-MCAO, brain water content, and 2,3,5-Triphenyltetrazolium chloride (TTC) staining and Evans blue staining were performed on tissues isolated from dedicated batches of mice from each grouping (see details of each protocol below). Western blot, qRT-PCR and ELISA experiments are the same batch of mice. Measures of modified neurological service scores (mNSS) were performed at the timepoints indicated below. In all studies, each group contained eight mice.
MCAO induction
The Longa method was used to establish a transient middle cerebral artery occlusion (MCAO) model in the mice (Belayev et al. Citation1996; Wang et al. Citation2018). In brief, mice were fasted for 6–8 h before surgery. Isoflurane gas (1–2%) was used to induce anesthesia (i.e., mouse limbs relaxed and spontaneous breathing deep and slow). At that point, a solution of 8% (w/v) sodium sulfide was used to remove the hair from the mouse neck. A lateral incision was then made at the top of the skull, and forceps were used to gently peel the connective tissue from the skull surface. An optical fiber probe from a laser Doppler blood flow meter was fixed with bio-adhesive to the area 2 mm behind the bregma and 5 mm on the left side, i.e., blood supply area of the middle cerebral artery.
The mouse was then placed on its back again, and a midline neck incision made. The muscle and fascia were separated along the inner edge of the sternocleidomastoid muscle, and the left common carotid artery (CCA), external carotid artery (ECA) and internal carotid artery (ICA) were separated. Arteriole clamps were used to temporarily clamp the ICA and CCA. The ECA was ligated and cut at the distal end, and a nylon monofilament thread (plug) coated with silicone fed into the ECA. Resistance was encountered when the thread plug reached a depth of 9–11 mm, causing a sharp drop in blood flow that was noted on the Doppler blood flow instrument. The thread (plug) was then fixed in place. Timing was started from when the thread (plug) entered the cerebrovascular vessel and the blood flow dropped. The thread (plug) was then slowly pulled after 30 min, the ECA was knotted at the proximal end, and the arterial clamp at the CCA was quickly released.
The skin on the neck and head of the mouse was then sutured and iodine was used to disinfect the wound area. The mice were placed on a temperature-regulating electric heating plate to maintain body temperature at 36–37 °C until they recovered from the operation. The animals in a sham operation (Sham) group were anesthetized and only their internal/external cervical branches exposed. An electrocoagulation pen was used to coagulate the lateral cervical vascular branches without occluding the middle cerebral artery. The surgical procedures are shown in Figure S1.
Figure 1. Gallic acid (GA) effects on cerebral ischemia/re-perfusion-induced brain edema and neurologic-deficits in mice. Brain water contents 3 day post-MCAO were compared between the (a) ipsilateral injury hemisphere and (b) contralateral un-injured hemisphere. Neurological deficit scores were measured at pre-, 1, 7, 14, and 21 d post-MCAO. N = 8 for each group. Data shown are means ± SD. *p < 0.05, **p < 0.01, ***p < 0.001 vs. MCAO group. ∧p < 0.05, ∧∧p < 0.01, ∧∧∧p < 0.001 vs. Sham group. One-way ANOVA followed by Dunn's multiple comparisons test and a two-way ANOVA followed by a Tukey's multiple comparisons test.
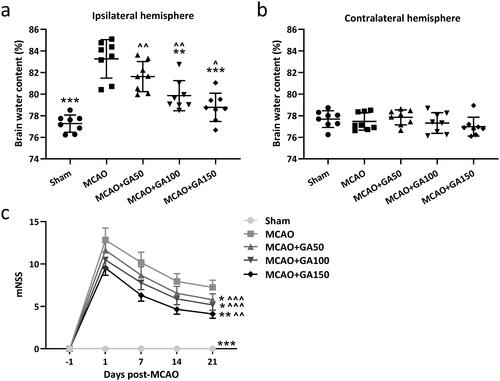
Modified neurological service scores (mNSS)
An mNSS approach was used to evaluate neurologic function pre- as well as 1, 7, 14, and 21 days post-MCAO. mNSS scoring was divided into three parts. In the first, when a mouse was lifted, the mouse that showed every following symptom was scored with one point: contralateral forelimb (left forelimb) was flexed, contralateral hind limb was flexed, and/or head rotation within 30 s deviated from central axis by > 10°. The sum of the three sub-items was the total score of the first part. In the second part, the mouse moved autonomously on a plane for 60 s. Each mouse was scored points as follows: 0 for normal walking, 1 point for inability to walk in straight line, 2 points for turning to hemiplegic side (left), and 3 points for involuntary movement for dumping to hemiplegic side. The third part was a test of balance. The scoring was as follows: mouse could stand on balance beam and maintain normal balance (0 points); mouse limbs could grab edge of wood (1 point); mouse could grab edge of balance beam (2 points); mouse could grab edge of beam and had two feet slip or keep turning on beam (3 points); mouse tried to maintain balance for > 40 s but still fell from beam (4 points); mouse tried to maintain balance for > 20 s but still fell from beam (5 points); and, mouse dropped from beam within 20 s (6 points). The total score was the sum of scores of the three parts. The final total score was then further characterized as 1–3 indicating mild injury, 4–7 for moderate injury, and 8–12 suggesting severe injury.
Brain water content
Brain water content was measured 3 d post-MCAO in a dedicated set of mice (N = 8) from each group. Mice were euthanized by IP injection of sodium pentobarbital (80 mg/kg). The brain tissues were the removed immediately, weighed (wet weight), and then placed in a 110 °C oven for 6–8 h to obtain brain tissue dry weight. Samples underwent repeated baking and weighing until the brain tissue quality difference was < 0.2 mg. Brain tissue water content was calculated as (% = 100%×([wet weight of brain tissue-dry weight of brain tissue])/wet weight of brain tissue.
2,3,5-Triphenyltetrazolium chloride (TTC) staining
TCC staining was performed 3 d post-MCAO on samples isolated from another dedicated set of mice (N = 8) from each group. Mice were euthanized by sodium pentobarbital injection and the brain of each mouse then quickly removed and placed at −20 °C for 10–15 min. Thereafter, six coronal brain slices of 1-mm thickness were continuously cut from the anterior pole (AP). Each slice was placed in a 1% TTC solution (dissolved in saline) and incubated for 10 min at 37 °C in a water bath. The slices were then removed and placed in 10% formaldehyde neutral buffer (pH 7.4) overnight to delineate normal (red) from infarct (pale/white) tissues. The slices were then examined under light microscopy in a blinded manner. Image ProPlus-6.0 (IPP.6.0) analysis software (Media Cybernetics, Bethesda, MD) was used to measure areas of the contralateral brain area, the non-infarct brain area on the injury side, and the infarct area. The average infarct area of the front and back of the brain slice was multiplied by the slice thickness (1 mm) to obtain the volume of the brain slice. The sum of the volume of all six brain slices for each mouse was then deemed the volume of the corresponding brain area.
Measurement of Evans blue extravasation
Measurement of Evans blue extravasation was performed 3 d post-MCAO in yet another dedicated set of mice (N = 8) from each group. Mice were anesthetized using 1–2% isoflurane (i.e., limbs relaxed and breathing became deep and slow) and then injected intravenously with 0.5% Evans blue Stain until their eyes and skin appeared blue. The mice were euthanized 0.5–1 h later (with sodium pentobarbital) and their brains removed. Each brain was placed in 1 ml PBS and a tissue homogenizer was used to quickly homogenize the materials. After centrifugation at 12,000 rpm for 15 min at 4 °C, the resulting supernatant was removed. An aliquot was then combined with an equal amount of 20% (w/v) trichloroacetic acid solution and the mixture incubated at 4 °C for 30 min. The solution was then assessed for absorbance at 620 nm using a Dataclolor UV–Vis spectrophotometer (Dataclolor, Beijing, China). The OD620 values of standard Evans blue solutions of different concentrations were measured in parallel to yield a standard curve from which dye levels in the samples could be extrapolated.
Biochemical testing
The ELISA, Western blot, and qRT-PCR assays were performed on tissues taken from dedicated sets of mice (N = 8) from each group at 3 d post-MCAO. Mice were euthanized by pentobarbital injection and then under aseptic conditions, ischemic cortical brain tissue was removed and placed on ice. From each sample, 20 mg tissue was isolated for use in ELISA, 10 mg for protein extraction, and 5 mg for RNA extraction. All samples were processed using a TGrinder (Tiangen) to fully disrupt and homogenize each tissue sample. Detailed methods for each of the three assays are presented below.
Enzyme-linked immunosorbent assay (ELISA)
Each tissue sample was homogenized in 500 µl PBS in a 1.5 ml centrifuge tube held on ice. Samples were then centrifuged at 12,000 rpm for 10 min (4 °C) and resultant supernatant was collected. ELISA kits specific for measures of interleukin (IL)-1β, IL-6, IL-10, monocyte chemoattractant protein (MCP)-1, tumor necrosis factor (TNF)-α, and macrophage inflammatory protein (MIP)-2 were bought from eBioscience (San Diego, CA). Each assay was performed according to manufacturer protocols. All samples were assayed in triplicate. Levels of cytokine in each sample were extrapolated from standard curves generated in parallel using kit-provided standards. Concentration of each cytokines was ultimately expressed in terms of pg/mg brain tissue. The sensitivity of the kits was 1.2 pg IL-1β/ml (Cat #BMS6002), 0.21 pg IL-6/ml (#BMS603HS), 32 pg IL-10/ml (#88-7105-22), 0.75 pg TNFα/ml (#BMS607HS), 2.2 pg MCP-1/ml (#BMS6005), and 10 pg MIP-2/ml (#EMCXCL2).
Western blot assay
Each tissue sample was homogenized in 500 µl RIPA lysing solution (Thermo Scientific, Waltham, MA) in a 1.5 ml centrifuge tube held on ice. The samples were then centrifuged at 12,000 rpm for 10 min (4 °C). Resultant supernatants were collected and total protein concentration determined using an Enhanced BCA Protein Assay Kit (Beyotime Institute of Biotechnology, Shanghai, China). Equal total protein amounts of each sample were then loaded into 10% polyacrylamide gels and the contents resolved using SDS–polyacrylamide constant pressure gel electrophoresis. The separated proteins were then electrotransferred to a PVDF membrane (Millipore, Darmstadt, Germany). The PVDF membrane was placed in 10% skimmed milk powder–TBST (pH 7.5, 10 mM Tris–HCl, 150 mM NaCl, 0.1% Tween 20) for 1 h at room temperature. For analysis of specific proteins, dedicated membranes were generated; this allowed for avoidance of issues of membrane stripping compromising the measured outcomes. Each dedicated membrane was then placed in a 5% bovine serum albumin–TBST solution containing the primary antibody of interest (at indicated manufacturer-recommended dilution). The primary antibodies employed were rabbit anti-mouse: Iba1/AIF-1 [E4O4W] XP#17198 (1:500, Cell Signaling Technology, Boston, MA), MMP9 [RM1020] (1:1000, ab283575, Abcam, Cambridge, UK), ZO1 tight junction protein [EPR19945-296] (1:800, ab221547, Abcam), Claudin 5 [EPR7583] (1:1000, ab131259, Abcam), and GAPDH (D16H11) XP#5174 (1:5000, Cell Signaling Technology). The monitoring of GAPDH was used for confirmation of gel loading.
Each membrane was incubated with its single antibody at 4 °C overnight before being washed (at room temperature) gently three times in PBS for 10 min. Each membrane was then placed in 5% bovine serum albumin–TBST solution containing horseradish peroxidase (HRP)-labeled secondary antibody (goat–anti-rabbit IgG, 1: 800, Beijing Zhongshan, Beijing) for 1 hr at room temperature. Each membrane was then gently rinsed with three times with TBST and incubated with ECL (Pierce, Elkhart, IN) luminescent substrate. The membranes were scanned (every 10 sec, stopping at 2 min) using an ImageQuant LAS.4000 Mini system (GE, Rahway, NJ) and data interpreted using system-associated software. Ratios of the gray value for each target protein compared to that for internal GAPDH reference were analyzed semi-quantitatively.
RNA extraction and qRT-PCR analysis
The total RNA in each sample was extracted using an RNeasy Mini Kit (Qiagen, Beijing, China) and then transcribed to cDNA using a FastKing RT Kit (with gDNase) (Tiangen, Beijing, China). QRT-PCR analysis was then performed using a FastKing One Step RT-qPCR Kit (SYBR Green) purchased from Tiangen. The 2–ΔΔCT method was used to calculate the relative expression of the target gene to be tested. GAPDH was used as the internal reference gene. The genes of interest to be analyzed in each sample were Iba-1, iNOS, COX-2, MCP-1, Arg-1, CD206 and IL-10. The primers used () were obtained from BGI (Shenzhen, China).
Table 1. Primers used in the study.
Statistical analysis
Data here are expressed in terms of means ± SD. To determine any significant differences among the groups for each given endpoint, an one-way analysis of variance (ANOVA) followed by a Dunn's multiple comparisons test, or a two-way ANOVA followed by a Tukey's multiple comparisons test, was performed. All analyses were done using Prism software (GraphPad, San Diego, CA). A p value < 0.05 was regarded as significant.
Results
GA treatment significantly reduced brain edema\neurologic function scores in MCAO mice
Cerebral edema is a common complication of ischemic stroke and reducing the edema is an important means to relieve symptoms of acute cerebral ischemia. In the study here, a wet/dry weight method was used to evaluate degree of brain edema in the mice. The results in showed the brain water content of mice in the MCAO group was significantly higher than in the sham group, and that a 50 mg GA/kg treatment had no significant effect on this parameter. In comparison, 100 or 150 mg GA/kg treatments led to a greatly-reduced brain water content.
The measured mNSS scores here reflected the motor function, sensory acuity, balance and reflex capabilities of the MCAO mice. The results shown in indicate there was no neurological deficit in the pseudo-operation group (Sham group; 0). In the MCAO models, most of the mice tilted their body to the opposite side of the injury when they walked freely, and even showed symptoms of involuntary movement. Score among some of these hosts reached ≈13 points. Using different concentrations of GA treatment led to significant reductions in these function score; in fact, there appeared to be a dose-related effect from the GA on these outcomes. The results suggested that the high-concentration GA treatment (150 mg/kg) could most significantly reduce brain edema and neurological changes in the MCAO model mice. Thus, to save on the use of animals, the 150 mg GA/kg dose was used in all the remaining experiments.
GA treatment significantly reduced cerebral infarction and protected blood–brain barrier (BBB) integrity in MCAO mice
Triphenyl tetrazolium chloride (TTC) is a light-sensitive proton acceptor for the pyridine nucleotide structural enzymes in the respiratory chain. A reaction of TTC with dehydrogenases in normal tissues is red; as enzyme activity in ischemic tissue is decreased, the TTC does not react and the tissue remains pale. The TTC staining results () showed that compared with in sham mice, infarction of the injured brain tissue was obvious after ischemia, and the total infarct area was about ≈40%. However, in hosts that received 150 mg GA/kg treatment, the cerebral infarction volume was significantly reduced (p < 0.001).
Figure 2. GA effects on cerebral ischemia/re-perfusion-induced brain infarct and blood–brain barrier (BBB) injury in mice. (a) Representative TTC staining 3 days after MCAO and (b) calculation of infarct area. Evans blue extravasation was used to measure BBB permeability [3 days post-MCAO] between (c) ipsilateral injury hemisphere and (d) contralateral un-injured hemisphere. N = 8 for each group. Data shown are means ± SD. ***p < 0.001 vs. MCAO group. ∧∧p < 0.01, ∧∧∧p < 0.001 vs. Sham group. One-way ANOVA followed by Dunn's multiple comparisons test.
![Figure 2. GA effects on cerebral ischemia/re-perfusion-induced brain infarct and blood–brain barrier (BBB) injury in mice. (a) Representative TTC staining 3 days after MCAO and (b) calculation of infarct area. Evans blue extravasation was used to measure BBB permeability [3 days post-MCAO] between (c) ipsilateral injury hemisphere and (d) contralateral un-injured hemisphere. N = 8 for each group. Data shown are means ± SD. ***p < 0.001 vs. MCAO group. ∧∧p < 0.01, ∧∧∧p < 0.001 vs. Sham group. One-way ANOVA followed by Dunn's multiple comparisons test.](/cms/asset/9add4c50-8412-4fc0-92aa-64ab0b0defa2/iimt_a_2043494_f0002_b.jpg)
The blood–brain barrier (BBB) protects brain tissue from toxicants in the blood and large shifts in electrolyte levels. During acute ischemic stroke, there is a change in BBB permeability. In the case of cerebral ischemia and re-perfusion, BBB permeability increases and water/plasma components leak into the surrounding brain tissue, causing vasogenic brain edema. In the present study, Evans blue extravasation was used to assess BBB permeability. The results showed that administration of 150 mg GA/kg significantly reduced the volume of cerebral infarction and reduced the exudation rate of the Evans blue dye ().
GA regulates the BBB injury-related protein expression in MCAO mice
Proteolytic matrix metalloproteinases (MMP) are involved in extracellular matrix degradation in various body tissues and have the effect of destroying vascular collagen, laminin, and fibronectin. Several papers have reported an increase in MMP expression after cerebral ischemia and re-perfusion (Rempe et al. Citation2016; Vafadari et al. Citation2016; Qin et al. Citation2019). Some MMP contribute to BBB destruction; among them, MMP-9 is particularly critical in that it degrades tight junction (TJ) proteins, vascular basement membranes, and extracellular matrix, etc. Here, Western blot assays were used to assess effects of GA on expression of BBB injury-related proteins. The results showed while MMP-9 was only slightly expressed in normal brain tissues, levels were significantly increased in ipsilateral injury hemispheres at 3 d post-MCAO () and that GA treatment significantly reduced this MMP-9 expression.
Figure 3. GA effects on cerebral ischemia/re-perfusion induced blood–brain barrier injury in mice. qRT-PCR and Western blotting were used to analyze mRNA/proteins levels of MMP9 in the ipsilateral injury hemispheres 3 d post-MCAO (a–c). Western blotting used to measure ZO-1 and Claudin-5 protein levels (d) in ipsilateral injury hemispheres 3 d post-MCAO. Relative expressions are shown (e and f). N = 3 from 8 mice for each group. Data shown are means ± SD. **p < 0.01, ***p < 0.001 vs. MCAO group. ∧p < 0.05, ∧∧p < 0.01 vs. Sham group. One-way ANOVA followed by Dunn's multiple comparisons test.
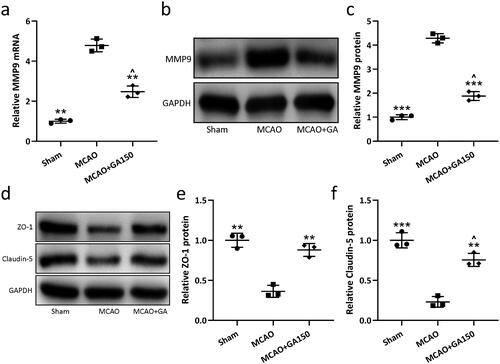
Tight junctions in endothelial cells are important for maintaining BBB integrity. These structures include some special mosaic proteins, like claudins and occludin. Accordingly, zonula occludens 1 (ZO-1) and claudin-5 expression in the ipsilateral injury hemispheres was assessed at 3 d post-MCAO. The results showed that ZO-1 and claudin-5 levels were significantly reduced by the cerebral ischemia–re-perfusion and GA treatment significantly increased the expression of ZO-1 and claudin-5 compared to in MCAO-only mice ().
GA inhibits microglia activation in MCAO mice
After ischemic brain injury, microglia were activated and morphological/functional changes occurred. Normally, after injury, the number of Iba-1+ microglia increases significantly, which leads to a cascade of damage to the central nervous system. In the present study, qRT-PCR and Western blot analyses were used to analyze mRNA and proteins levels of Iba-1 in the ipsilateral injury hemispheres at 3 d post-MCAO to evaluate microglia activation status. The results showed that both mRNA and proteins levels of Iba-1 were significantly increased in MCAO mice compared to in sham controls; treatment with 150 mg GA/kg suppressed this up- regulation ().
Figure 4. GA effects on cerebral ischemia/re-perfusion-induced microglial activation in mice. qRT-PCR and Western blotting were used to analyze mRNA/proteins levels of Iba-1 in ipsilateral injury hemispheres 3 d post-MCAO (a–c). N = 3 from 8 mice for each group. Data shown are means ± SD. ***p < 0.001 vs. MCAO group. ∧p < 0.05 vs. Sham group. One-way ANOVA followed by Dunn's multiple comparisons test.
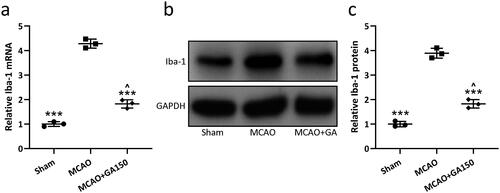
GA attenuates cerebral ischemia/re-perfusion induced inflammatory responses in mice
To investigate effects of GA on inflammatory responses induced by cerebral ischemia/re- perfusion, levels of multiple cytokines in the ipsilateral injury hemispheres of the mice were evaluated using ELISA. The data indicate that MACO treatment induced dramatic increases in IL-1β, MCP-1, TNFα, IL-6, and MIP-2 (, respectively) levels in the injured brain tissues. However, 150 mg/kg GA treatment induced a significant decrease in each of these. Moreover, the GA treatment induced significant up-regulation of IL-10 levels in the injured brain tissue compared to in the MCAO-only mice ().
Figure 5. GA effects on cerebral ischemia/re-perfusion-induced inflammatory responses in mice. Protein levels of (a) IL-1β, (b) MCP-1, (c) TNFα, (d) IL-6, (e) MIP-2, and (f) IL-10 in ipsilateral injury hemispheres 3 d post-MCAO [ELISA]. N = 8 for each group. Data shown are means ± SD. *p < 0.05, **p < 0.01, ***p < 0.001 vs. MCAO group. ∧p < 0.05, ∧∧∧p < 0.001 vs. Sham group. One-way ANOVA followed by Dunn's multiple comparisons test.
![Figure 5. GA effects on cerebral ischemia/re-perfusion-induced inflammatory responses in mice. Protein levels of (a) IL-1β, (b) MCP-1, (c) TNFα, (d) IL-6, (e) MIP-2, and (f) IL-10 in ipsilateral injury hemispheres 3 d post-MCAO [ELISA]. N = 8 for each group. Data shown are means ± SD. *p < 0.05, **p < 0.01, ***p < 0.001 vs. MCAO group. ∧p < 0.05, ∧∧∧p < 0.001 vs. Sham group. One-way ANOVA followed by Dunn's multiple comparisons test.](/cms/asset/5f03da48-1173-4cf6-88e4-4fa7029e5fc5/iimt_a_2043494_f0005_b.jpg)
GA inhibits M1-type polarization but promotes M2-type polarization of microglia after cerebral ischemia/re-perfusion injury in mice
To explore if any ability of GA to modulate the inflammatory response was related to activation of innate immune cells in the brain and the microglia (and a phenotypic transition), real-time quantitative PCR was used to measure tissue changes in expression of typical markers of M1/M2-type microglia in the cerebral infarction area after ischemic stroke. The results showed that at 3 d after MCAO modeling, mRNA levels of microglia M1 type markers iNOS, COX-2, and MCP-1 (, respectively) and M2 type markers Arg-1, CD206, and IL-10 (, respectively) increased. These outcomes suggested that in the mice, after ischemic brain injury, microglia were activated and levels of both M1- and M2-type microglia were significantly increased. However, after treatment with 150 mg GA/kg, the levels of M1 markers iNOS, COX-2, and MCP-1 were remarkably decreased, whereas those of M2 markers Arg-1, CD206, and IL-10 were further increased compared to in tissues from MCAO-only mice. This suggested that in the acute phase of ischemic brain injury, GA (at least at 150 mg GA/kg) could inhibit M1-type conversions of microglia and increase their conversion to M2 type.
Figure 6. GA effects on M1-type and M2-type microglial polarization after cerebral ischemia/re-perfusion injury in mice. mRNA levels of (a) iNOS, (b) COX-2, (c) MCP-1, (d) Arg-1, (e) CD206, and (f) IL-10 in ipsilateral injury hemispheres 3 d post-MCAO [qRT-PCR]. N = 3 from 8 mice for each group. GAPDH was evaluated in parallel as a housekeeping gene. Data shown are means ± SD. **p < 0.01, ***p < 0.001 vs. MCAO group. ∧p < 0.05, ∧∧∧p < 0.001 vs. Sham group. One-way ANOVA followed by Dunn's multiple comparisons test.
![Figure 6. GA effects on M1-type and M2-type microglial polarization after cerebral ischemia/re-perfusion injury in mice. mRNA levels of (a) iNOS, (b) COX-2, (c) MCP-1, (d) Arg-1, (e) CD206, and (f) IL-10 in ipsilateral injury hemispheres 3 d post-MCAO [qRT-PCR]. N = 3 from 8 mice for each group. GAPDH was evaluated in parallel as a housekeeping gene. Data shown are means ± SD. **p < 0.01, ***p < 0.001 vs. MCAO group. ∧p < 0.05, ∧∧∧p < 0.001 vs. Sham group. One-way ANOVA followed by Dunn's multiple comparisons test.](/cms/asset/dfda4cd0-cc7b-444a-88d0-ea2bfc313ad8/iimt_a_2043494_f0006_b.jpg)
Discussion
At present, the cost of treating strokes annually imposes a heavy economic burden on society and on many families. The mechanism of ischemic stroke mainly involves excitotoxic injury, oxidative stress injury, inflammatory response and apoptosis (Radu et al. Citation2017). The research and development of drugs for ischemic stroke also focuses on the above mechanisms. Most of the effective drugs or compounds in pre-clinical trials have shown a variety of drawbacks in clinical trials and could not be well applied to the treatment of acute ischemic stroke (Pan et al. Citation2019; Rabinstein Citation2020). Therefore, finding effective drugs for the treatment of acute ischemic stroke is the top priority of ischemic cerebrovascular disease research.
Cerebrovascular disease is a common frequently-occurring pathology. The complexity of the pathologies present, and the underlying mechanisms for their development after cerebral ischemia determines the difficulty of treatment. Finding new and effective drugs that could block or reduce the cerebral ischemic cascade are an intense focus of research. Studies have shown that within a few hours after cerebral ischemia, an inflammatory response occurs immediately, and microglia are activated (Xu et al. Citation2020). The role of microglia in this response to cerebral ischemia is complex; it is believed that microglial activation leads to increased release of nitric oxide, oxygen free radicals, and other toxic substances, all which act to damage neurons (Jiang et al. Citation2020). As activation of microglia continues for a few weeks after ischemia, it would seem that interventions against this second type of brain injury (i.e., caused by inflammatory reactions) should become an additional target for the developers of neuroprotective drugs.
Gallic acid (GA), a polyphenol compound that exists widely in nature (Kosuru et al. Citation2018), has been shown to impart a variety of effects, i.e., antidepressant, anti-Parkinson’s disease, antidiabetic, antimalaria, and antiviral (Kratz et al. Citation2008; Patel and Goyal Citation2011). GA has also been reported to help to alleviate acetaminophen-induced liver injury in mice, reduce liver ischemia–re-perfusion as well as CCl4-induced acute liver injury in rats, and suppress sodium fluoride-induced oxidative stress in the kidneys of rats (Kanai and Okano Citation1998; Nabavi et al. Citation2013).
In terms of neuropharmacologic effects, GA effectively inhibits oxidative stress damage (and mitigates memory dysfunction) in animal models caused by intracerebral streptozotocin injection (Mansouri et al. Citation2013). It is also known that in vitro, GA can inhibit the viability, proliferation, invasivity, and angiogenic activities of human glioma cells (Lu et al. Citation2010) and can also inhibit proliferation of lymphocytes (Serrano et al. Citation1998). Even with the above-cited findings, it is still unclear as to whether or how GA might be of use in preventing/treating cerebral ischemia–re-perfusion injury. Accordingly, the main purpose of this study was to assess potential neuroprotective effects of GA in vivo, by evaluating its value in the treatment of ischemic brain injury. Using an MCAO mouse model, it was seen here that after ischemic brain injury, GA treatment was effective in improving the motor sensory function of mice, and in reducing the area of brain edema and volume of cerebral infarction. These outcomes suggested to us that GA may have a protective effect against ischemic brain injury.
Microglia constitute a first line of immune defense in the brain (Prinz et al. Citation2019) and participate in a variety of brain injury pathological processes, including stroke. After brain injury, innate microglia quickly mobilize to the injured area and secrete a variety of cytokines/chemokines to recruit other immune cells (Karve et al. Citation2016). While there is still uncertainty about the precise role(s) of microglia in ischemic brain injury (Ma et al. Citation2017), recent studies suggested dual “protective” and “damaging” effects after a stroke as well as in some other neurological pathologies (Qin et al. Citation2019). With regard to any purported protective effect, one study showed that selective inhibition of microglia proliferation aggravated the tissue damage during post-brain injury periods (Ma et al. Citation2017). Another study showed that exogenous injection of microglia into the brain parenchyma alleviated symptoms of brain injury (Xu et al. Citation2016). In contrast, damaging effects of microglia/macrophages appear mainly attributable to excessive secretion of neurotoxic substances that prevent regeneration of neurons\axons after stroke and limits on any thrombolytic effects (Abe et al. Citation2020). Other studies suggested that excessive activation of microglia caused secondary effects that aggravated brain damage after ischemia (Rajan et al. Citation2019).
It is believed the diversity of microglia functions mainly depends on the phenotypes induced by different microenvironments. Activated microglia can be divided into M1 type and M2 subtypes that impart pro- and anti-inflammatory effects, respectively (Szalay et al. Citation2016). A dynamic transition among the M1:M2 phenotypes of microglia after cerebral ischemic injury has been reported in related studies (Tang and Le Citation2016). In the early stage after ischemic brain injury, a large number of microglial cells migrate to the infarct area and mostly manifest as the M2 type (Zhao et al. Citation2017) that exert a “protective” function. Compared to the M1 subtype, M2 type microglia evince enhanced phagocytic activity and reduced secretion of inflammatory mediators (Wang et al. Citation2018). Studies have shown that these M2 type microglia remove cell debris through cell-to-cell contact and promote survival of cortical neurons (Ma et al. Citation2020). Other studies have reported that M2 type microglia can promote neuronal axon growth or germination, suggesting they play an important role in tissue repair and nerve regeneration (Wang et al. Citation2018). Therefore, the early polarization of endogenous M2 type microglia can be considered as an endogenous protective mechanism in a body to limits the infarct volume by removing necrotic tissue (Zhang et al. Citation2019). On this basis, it would seem then that maintaining microglia in the type M2 state may help to alleviate/mitigate the extent of brain damage that occurs after ischemia.
The present study provides insights into effects of GA treatment on microglia activation and phenotypic transformation. However, a non-negligible limitation of this study remains that the data generated from mouse stroke models have not yet been translated into effective human treatments based on experiences in the field of stroke research. In fact, it is still controversial as to whether accurate modeling of stroke in animal models can reflect the real situation of this pathology in humans. In addition, a treatment that proved effective in animal experiments (i.e., NXY-059) also failed in human clinical trials (Antonic et al. Citation2018). Although one can employ vessel clipping or insert artificial blood clots to mimic the pathological changes caused by stroke in animal models, it is undeniable that most animals do not develop atherosclerosis naturally. Therefore, it remains for now only plausible to focus on the protective effect of GA on the brain tissue in the stroke region and whole brain after a stroke event. While it was hoped that here it might be possible to physically simulate the changes that occur in the human brain after a stroke, actually delving into the potential preventive effects of GA against stroke remained unachievable. Ongoing studies in our laboratories will continue to focus on this issue in future research.
Conclusions
The study here explored effects of GA treatment on microglia activation and phenotypic transformation. The results showed that in the early stage after cerebral ischemia, the levels of M2 type microglia increased (and M1 type decreased) in the ischemic areas of the brains of the injured mice. It was also noted that the GA treatment significantly reduced the degree of brain edema, led to increased integrity of the BBB, and also led to improved neurologic function scores in these mice. Whether these three parameters are a direct result of the change in M1 vs. M2 subtype presence is not certain, but any decreases in release of pro-inflammatory factors would certainly contribute to reductions in tissue swelling, permit BBB integrity to not undergo attack/challenge, and allow for nerves throughout the brain (even beyond the site of ischemic damage) to suffer from less inflammation-related stress. Taking all of these outcomes together, it is reasonable to suggest that use of GA either as a prophylactic or immediately in the event of a cerebral ischemic event/stroke could help to promote neuronal survival and allow for a more likely of host neurological function over time.
Ethical approval
All experimental animals were provided by the institutional animal care and use committee of Liaocheng People's Hospital.
Disclosure statement
The authors declare no conflicts of interest. The authors alone are responsible for the content of this manuscript.
Data availability statement
The datasets supporting the results of this article are included within the article. Further enquiries can be directed to the corresponding author.
References
- Abe N, Nishihara T, Yorozuya T, Tanaka J. 2020. Microglia and macrophages in the pathological central and peripheral nervous systems. Cells. 9(9):2132.
- Ahn CB, Jung WK, Park SJ, Kim YT, Kim WS, Je JY. 2016. Gallic acid-g-chitosan modulates inflammatory responses in LPS-stimulated RAW264.7 cells via NF-κB, AP-1, and MAPK pathways. Inflammation. 39(1):366–374.
- Akhtar A, Kamal A. 2013. Benefit of neuroprotection in acute ischaemic stroke: Shall we dare to hope? J Pak Med Assoc. 63:792.
- Antonic A, Dottori M, Macleod M, Donnan G, Howells D. 2018. NXY-059, a failed stroke neuroprotectant, offers no protection to stem cell-derived human neurons. J Stroke Cerebrovasc Dis. 27(8):2158–2165.
- Belayev L, Alonso O, Busto R, Zhao W, Ginsberg M. 1996. Middle cerebral artery occlusion in the rat by intraluminal suture. Neurological and pathological evaluation of an improved model. Stroke. 27(9):1616–1622.
- Diaz A, Muñoz-Arenas G, Caporal-Hernandez K, Vázquez-Roque R, Lopez-Lopez G, Kozina A, Espinosa B, Flores G, Treviño S, Guevara J. 2020. Gallic acid improves recognition memory and decreases oxidative-inflammatory damage in the rat hippocampus with metabolic syndrome. Synapse. 75(2):e22186.
- Dirnagl U, Iadecola C, Moskowitz M. 1999. Pathobiology of ischemic stroke: An integrated view. Trends Neurosci. 22(9):391–397.
- Emsley H, Hopkins S. 2008. Acute ischaemic stroke and infection: Recent and emerging concepts. Lancet Neurol. 7(4):341–353.
- Fernandes F, Salgado H. 2016. Gallic acid: Review of the methods of determination and quantification. Crit Rev Anal Chem. 46(3):257–265.
- Jiang C, Wu W, Deng Y, Ge J. 2020. Modulators of microglia activation and polarization in ischemic stroke. Mol Med Rep. 21(5):2006–2018.
- Kahkeshani N, Farzaei F, Fotouhi M, Alavi S, Bahramsoltani R, Naseri R. 2019. Pharmacological effects of gallic acid in health and diseases: A mechanistic review. Iran J Basic Med Sci. 22:225–237.
- Kanai S, Okano H. 1998. Mechanism of the protective effects of sumac gall extract and gallic acid on the progression of CCl4-induced acute liver injury in rats. Am J Chin Med. 26(3–4):333–341.
- Karve I, Taylor J, Crack P. 2016. The contribution of astrocytes and microglia to traumatic brain injury. Br J Pharmacol. 173(4):692–702.
- Kim J, Fann D, Seet R, Jo D, Mattson M, Arumugam T. 2016. Phytochemicals in ischemic stroke. Neuromol Med. 18(3):283–305.
- Kosuru R, Roy A, Das S, Bera S. 2018. Gallic acid and gallates in human health and disease: Do mitochondria hold the key to success? Mol Nutr Food Res. 62:1700080.
- Kratz JM, Andrighetti-Fröhner CR, Kolling DJ, Leal PC, Cirne-Santos CC, Yunes RA, Nunes RJ, Trybala E, Bergström T, Frugulhetti ICPP, et al. 2008. Anti-HSV-1 and anti-HIV-1 activity of gallic acid and pentyl gallate. Mem Inst Oswaldo Cruz. 103(5):437–442.
- Lu Y, Jiang F, Jiang H, Wu K, Zheng X, Cai Y, Katakowski M, Chopp M, To S. 2010. Gallic acid suppresses cell viability, proliferation, invasion and angiogenesis in human glioma cells. Eur J Pharmacol. 641(2–3):102–107.
- Ma D, Zhang N, Zhang Y, Chen H. 2020. Kv1.3 channel blockade alleviates cerebral ischemia/re-perfusion injury by re-shaping M1/M2 phenotypes and compromising the activation of NLRP3 inflammasome in microglia. Exp Neurol. 332:113399.
- Ma Y, Wang J, Wang Y, Yang G. 2017. The biphasic function of microglia in ischemic stroke. Prog Neurobiol. 157:247–272.
- Mansouri M, Naghizadeh B, Ghorbanzadeh B, Farbood Y, Sarkaki A, Bavarsad K. 2013. Gallic acid prevents memory deficits and oxidative stress induced by intracerebroventricular injection of streptozotocin in rats. Pharmacol Biochem Behav. 111:90–96.
- Mirshekar M, Sarkaki A, Farbood Y, Gharib Naseri M, Badavi M, Mansouri M. 2018. Neuro-protective effects of gallic acid in a rat model of traumatic brain injury: Behavioral, electro-physiological, and molecular studies. Iran J Basic Med Sci. 21:1056–1063.
- Nabavi SM, Habtemariam S, Nabavi SF, Sureda A, Daglia M, Moghaddam AH, Amani MA. 2013. Protective effect of gallic acid isolated from Peltiphyllum peltatum against sodium fluoride-induced oxidative stress in rat's kidney. Mol Cell Biochem. 372(1–2):233–239.
- Orellana-Urzua S, Rojas I, Libano L, Rodrigo R. 2020. Pathophysiology of ischemic stroke: Role of oxidative stress. Curr Pharm Des. 26(34):4246–4260.
- Pan Y, Elm JJ, Li H, Easton JD, Wang Y, Farrant M, Meng X, Kim AS, Zhao X, Meurer WJ, et al. 2019. Outcomes associated with clopidogrel–aspirin use in minor stroke or transient ischemic attack: A pooled analysis of clopidogrel in high-risk patients with acute non-disabling cerebrovascular events (CHANCE) and platelet-oriented inhibition in new TIA and minor ischemic stroke (POINT) trials. JAMA Neurol. 76(12):1466–1473.
- Patel S, Goyal R. 2011. Cardioprotective effects of gallic acid in diabetes-induced myocardial dysfunction in rats. Pharmacogn Res. 3(4):239–245.
- Prinz M, Jung S, Priller J. 2019. Microglia biology: One century of evolving concepts. Cell. 179(2):292–311.
- Qin C, Zhou L-Q, Ma X-T, Hu Z-W, Yang S, Chen M, Bosco DB, Wu L-J, Tian D-S. 2019. Dual functions of microglia in ischemic stroke. Neurosci Bull. 35(5):921–933.
- Qin W, Li J, Zhu R, Gao S, Fan J, Xia M, Zhao RC, Zhang J. 2019. Melatonin protects blood–brain barrier integrity and permeability by inhibiting matrix metalloproteinase-9 via the NOTCH3/NF-κB pathway. Aging (Albany NY). 11(23):11391–11415.
- Rabinstein A. 2020. Update on treatment of acute ischemic stroke. Continuum (Minneap Minn). 26(2):268–286.
- Radu RA, Terecoasă EO, Băjenaru OA, Tiu C. 2017. Etiologic classification of ischemic stroke: Where do we stand? Clin Neurol Neurosurg. 159:93–106.
- Rajan W, Wojtas B, Gielniewski B, Gieryng A, Zawadzka M, Kaminska B. 2019. Dissecting functional phenotypes of microglia and macrophages in the rat brain after transient cerebral ischemia. Glia. 67(2):232–245.
- Rempe R, Hartz A, Bauer B. 2016. Matrix metalloproteinases in the brain and blood–brain barrier: Versatile breakers and makers. J Cereb Blood Flow Metab. 36(9):1481–1507.
- Roach R, Helmerhorst F, Lijfering W, Stijnen T, Algra A, Dekkers O. 2015. Combined oral contraceptives: Risk of myocardial infarction and ischemic stroke. Cochrane Database Syst Rev. 2015:CD011054.
- Serrano A, Palacios C, Roy G, Cespon C, Villar M, Nocito M, Gonzalez-Porque P. 1998. Derivatives of gallic acid induce apoptosis in tumoral cell lines and inhibit lymphocyte proliferation. Arch Biochem Biophys. 350(1):49–54.
- Sidney S, Rosamond W, Howard V, Luepker R, National Forum for Heart Disease and Stroke. 2013. The “heart disease and stroke statistics – 2013 update” and the need for a national cardiovascular surveillance system. Circulation. 127(1):21–23.
- Song Y, Li Z, He T, Qu M, Jiang L, Li W, Shi X, Pan J, Zhang L, Wang Y, et al. 2019. M2 microglia-derived exosomes protect the mouse brain from ischemia–re-perfusion injury via exosomal miR-124. Theranostics. 9(10):2910–2923.
- Szalay G, Martinecz B, Lénárt N, Környei Z, Orsolits B, Judák L, Császár E, Fekete R, West BL, Katona G, et al. 2016. Microglia protect against brain injury and their selective elimination dysregulates neuronal network activity after stroke. Nat Commun. 7:11499.
- Tang Y, Le W. 2016. Differential roles of M1 and M2 microglia in neurodegenerative diseases. Mol Neurobiol. 53(2):1181–1194.
- Wang J, Xing H, Wan L, Jiang X, Wang C, Wu Y. 2018. Treatment targets for M2 microglia polarization in ischemic stroke. Biomed Pharmacother. 105:518–525.
- Wang Y, Huang Y, Xu Y, Ruan W, Wang H, Zhang Y, Saavedra JM, Zhang L, Huang Z, Pang T. 2018. A Dual AMPK/Nrf2 activator reduces brain inflammation after stroke by enhancing microglia M2 polarization. Antioxid Redox Signal. 28(2):141–163.
- Willis EF, MacDonald KPA, Nguyen QH, Garrido AL, Gillespie ER, Harley SBR, Bartlett PF, Schroder WA, Yates AG, Anthony DC, et al. 2020. Repopulating microglia promote brain repair in an IL-6-dependent manner. Cell. 180(5):833–846.
- Xu L, He D, Bai Y. 2016. Microglia-mediated inflammation and neurodegenerative disease. Mol Neurobiol. 53(10):6709–6715.
- Xu S, Lu J, Shao A, Zhang J, Zhang J. 2020. Glial cells: Role of the immune response in ischemic stroke. Front Immunol. 11:294.
- Vafadari B, Salamian A, Kaczmarek L. 2016. MMP-9 in translation: From molecule to brain physiology, pathology, and therapy. J Neurochem. 139(S2):91–114.
- Zhang XJ, Lv MM, Zhu XQ, Tian LY, Li JJ, Shao YP, Gao CJ, Sun XD. 2019. Microglia M1/M2 polarization contributes to electromagnetic pulse-induced brain injury. J Biol Regul Homeost Agents. 33(4):1051–1062.
- Zhao S, Ma L, Chu Z, Xu H, Wu W, Liu F. 2017. Regulation of microglial activation in stroke. Acta Pharmacol Sin. 38(4):445–458.