Abstract
Per- and polyfluoroalkyl substances (PFASs) are used in a multitude of processes and products, including nonstick coatings, food wrappers, and fire-fighting foams. These chemicals are environmentally-persistent, ubiquitous, and can be detected in the serum of 98% of Americans. Despite evidence that PFASs alter adaptive immunity, few studies have investigated their effects on innate immunity. The report here presents results of studies that investigated the impact of nine environmentally-relevant PFASs [e.g. perfluorooctanoic acid (PFOA), perfluorooctane sulfonic acid potassium salt (PFOS-K), perfluorononanoic acid (PFNA), perfluorohexanoic acid (PFHxA), perfluorohexane sulfonic acid (PFHxS), perfluorobutane sulfonic acid (PFBS), ammonium perfluoro(2-methyl-3-oxahexanoate) (GenX), 7H-perfluoro-4-methyl-3,6-dioxa-octane sulfonic acid (Nafion byproduct 2), and perfluoromethoxyacetic acid sodium salt (PFMOAA-Na)] on one component of the innate immune response, the neutrophil respiratory burst. The respiratory burst is a key innate immune process by which microbicidal reactive oxygen species (ROS) are rapidly induced by neutrophils in response to pathogens; defects in the respiratory burst can increase susceptibility to infection. The study here utilized larval zebrafish, a human neutrophil-like cell line, and primary human neutrophils to ascertain whether PFAS exposure inhibits ROS production in the respiratory burst. It was observed that exposure to PFHxA and GenX suppresses the respiratory burst in zebrafish larvae and a human neutrophil-like cell line. GenX also suppressed the respiratory burst in primary human neutrophils. This report is the first to demonstrate that these PFASs suppress neutrophil function and support the utility of employing zebrafish larvae and a human cell line as screening tools to identify chemicals that may suppress human immune function.
Introduction
Per- and polyfluoroalkyl substances (PFASs) are a diverse group of anthropogenic chemicals that contain linked chains of carbon and fluorine. As a class, these compounds are used to produce nonstick cookware, hydrophobic and oleophobic fabrics and coatings, fire-fighting foams, food wrappers, and more (Evich et al. Citation2022). The convenience provided by the unique chemistry of these compounds is now overshadowed by their persistence and ubiquity in the environment (Ahrens et al. Citation2009; Wang et al. Citation2017), and the fact that many PFASs studied to date bioaccumulate in biota (Conder et al. Citation2008; Buck et al. Citation2011; Ng and Hungerbühler Citation2014). Human exposure to these compounds is commonplace due to their presence in surface and drinking water – it is estimated that PFASs contaminate the drinking water of ∼200 million Americans and are detectable in the serum of 98% of Americans (Calafat et al. Citation2007; Hu et al. Citation2016; Centers for Disease Control and Prevention (CDC Citation2019; Andrews and Naidenko Citation2020). Of concern, PFASs are associated with a myriad of adverse health outcomes in humans, experimental animals, and wildlife (Agency for Toxic Substances and Disease Registry (ATSDR) Citation2021; Fenton et al. Citation2021).
PFASs have been linked to several immunotoxic outcomes in humans including ulcerative colitis (Steenland et al. Citation2013), allergic sensitization (Humblet et al. Citation2014; Buser and Scinicariello Citation2016; Averina et al. Citation2019), decreased response to vaccines (Grandjean et al. Citation2017a,Citation2017b), and increased susceptibility to infectious disease (Dalsager et al. Citation2016; Goudarzi et al. Citation2017; Ait Bamai et al. Citation2020). This body of evidence has led the National Toxicology Program (NTP) to conclude that two PFASs, perfluorooctanoic acid (PFOA) and perfluorooctane sulfonic acid (PFOS), are “presumed to be immune hazards to humans” (National Toxicology Program (NTP) Citation2016).
It has been well-established that these PFASs are immunotoxic in humans and experimental animal models (DeWitt et al. Citation2019). To date, however, most studies have focused on the impact of these and a small number of other PFASs on lymphocytes and their functions, including studies of their effects on T- and/or B-cell proliferation (Peden-Adams et al. Citation2007; Dong et al. Citation2009; Zheng et al. Citation2009; Brieger et al. Citation2011), cytokine production (Zhen et al. Citation2011a; Midgett et al. Citation2015), and NK cell cytolysis (Peden-Adams et al. Citation2007, Citation2008; Keil et al. Citation2008; Dong et al. Citation2009; Zheng et al. Citation2009; Brieger et al. Citation2011). Most notably, there have been several studies on the effects of PFAS exposure on antibody production in experimental animals (Yang et al. Citation2002; DeWitt et al. Citation2008, Citation2009, Citation2016; Zheng et al. Citation2009; Dong et al. Citation2011; Zheng et al. Citation2011a; Zhong et al. Citation2020; De Guise and Levin Citation2021), as well as immunophenotyping (Dong et al. Citation2009; Zheng et al. Citation2009; Rockwell et al. Citation2013; DeWitt et al. Citation2016), albeit the latter is a less sensitive and specific measure of functional immunotoxicity.
The effects of PFASs on innate myeloid cells (e.g. neutrophils, macrophages) have gone understudied, with only a few studies investigating if PFASs modulate innate immune function (Brieger et al. Citation2011; Berntsen et al. Citation2018; Liang et al. Citation2022). Even with this large data gap, manufacturing of PFASs continues, and the number of PFASs is growing, with the Organization for Economic Cooperation and Development Database listing nearly 5,000 unique PFASs (Organization for Economic Co-operation and Development 2018) and the United States Environmental Protection Agency (USEPA) listing more than 12,000 PFASs (Environmental Protection Agency (EPA) Citation2020). Toxicological studies have been conducted on a relatively small number of these PFASs, and there remains a scarcity of studies regarding their effects on innate immune function. Filling this gap is critical to understand the breadth of immunological ramifications of exposure to PFASs.
Recently, laboratories here reported that larval zebrafish can be used as a screening tool to identify compounds that suppress one component of innate immune function, the respiratory burst (Phelps et al. Citation2020). The respiratory burst is a critical function of neutrophils and macrophages involving the rapid production of reactive oxygen species (ROS), which have microbicidal properties and act as second messengers in signal transduction, further amplifying immune responses (Dupré-Crochet et al. Citation2013; Herb and Schramm Citation2021). In neutrophils, the respiratory burst is preceded by degranulation (Manara et al. Citation1991), and ROS production is dependent upon the activities of myeloperoxidase (MPO) and NADPH oxidase (Dahlgren et al. Citation2019), indicating that measuring the respiratory burst can serve as a broad readout of neutrophil function. Mutations in MPO, NADPH oxidase, or upstream kinases (e.g. protein kinase C [PKC]) have been linked to immunosuppression and an increased susceptibility to infection (Klebanoff Citation1970; Drutman et al. Citation2020; Neehus et al. Citation2021, Citation2022; Yu et al. Citation2023).
The present study sought to expand on the earlier work here by screening nine environmentally-relevant PFASs for their ability to suppress the respiratory burst in larval zebrafish. Additionally, the in vivo methods here were also adapted for use in the human neutrophil-like HL-60 cell line (Gallagher et al. Citation1979) to measure the respiratory burst in vitro to better model human responses after exposure to PFASs. HL-60 cells are a human promyeloblast cell line that can be differentiated to a neutrophil-like phenotype which can perform key neutrophil functions such as the respiratory burst, phagocytosis, chemotaxis, and release of neutrophil extracellular traps (NETs), making them an excellent model for human neutrophil function (Blanter et al. Citation2021). To validate the in vitro results, isolated primary human neutrophils were also exposed to PFASs ex vivo and assessed for their respiratory burst. With the in vivo, in vitro, and ex vivo respiratory burst assays, the present data demonstrate for the first time that a short-chain perfluoroether carboxylic acid − GenX − suppressed the neutrophil respiratory burst.
Materials and methods
Chemicals
Full names, chemical structures, and CAS numbers of test compounds are in and . Stock solutions of all PFASs were created at a concentration of 80 mM. Due to solubility limitations, the camptothecin (CAMP) stock solution was prepared at a concentration of 15 mM. Because recent reports indicate that DMSO is not a suitable solvent for GenX (Gaballah et al. Citation2020; Liberatore et al. Citation2020; Zhang et al. Citation2022), the stock solutions for the per- and polyfluorinated ether acids (GenX, Nafion byproduct 2, and PFMOAA-Na) were prepared in molecular biology-grade water; all other stock solutions were prepared in DMSO. All PFASs were stored at −80 °C; CAMP was stored at −20°C, as recommended by the manufacturer.
Figure 1. PFASs used in this study. Names and structures of the PFASs used in this study. Vendors and CAS-RN numbers are provided in . Chemical structures were generated with ChemDraw Professional (v16.0.1.4) using International Union of Pure and Applied Chemistry (IUPAC) nomenclature.
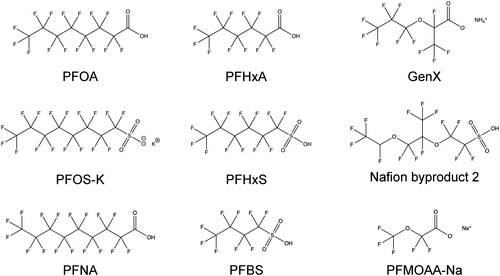
Table 1. PFAS names, CAS numbers, sources, and characteristics.
Exposure solutions were prepared on the first day of dosing by thawing and diluting aliquots of stock solutions. Exposure solutions were prepared at a 250X concentration in amber glass vials to minimize adsorption. Solutions were prepared using serial dilutions using the solvent in which the compound was originally prepared. Vehicle controls (DMSO at a final concentration of 0.4% or molecular-biology grade water) were also employed.
Zebrafish methods
Vertebrate animals
Zebrafish husbandry and experiments involving live animals were approved by the North Carolina State University Institutional Animal Care and Use Committee. Adult zebrafish were maintained in a recirculating aquarium facility (Aquatic Habitats, Apopka, FL) at 28 °C with a 14 hr light/10 hr dark cycle and fed a commercial grade zebrafish diet. Wild-type zebrafish were originally purchased from LiveAquaria (Dayton, OH) and Doctors Foster and Smith (Rhinelander, WI) and maintained and bred in-house for > 5 years. Zebrafish embryos were obtained by natural spawning (Westerfield Citation2007). At 2 hr post-fertilization (hpf), embryos were treated with 0.06% sodium hypochlorite (bleach [v/v]) in 10% Hanks saline (Westerfield Citation2007) in ultrapure water using two 5-min washes to eliminate extra-ovum microbes. The embryos were then maintained in 100 mm Petri dishes in 10% Hank’s saline at 28 °C until use.
In vivo exposures of zebrafish larvae to PFASs and developmental toxicity assays
Zebrafish embryos were exposed to individual PFASs as described in Phelps et al. (Citation2020) with slight modifications. In the present study, 6 hpf embryos were sorted into 6-well plates containing 5 ml of 10% Hank’s saline at a density of 10 embryos per well. After sorting, 20 µl of the 250X exposure solution were added to each well for a 1X final chemical concentration. Plates were gently swirled to disperse the chemical, wrapped in parafilm, and placed in a 28°C incubator on a 14 hr light/10 hr dark cycle. Prior to daily media changes, the plates were inspected for abnormal or dead embryos/larvae, which were removed from the wells. The plates then received a 99% media change by performing two consecutive 90% media changes. The test compound was replenished after each media change.
Developmental toxicity assays in larval zebrafish
Embryos/larvae were exposed to PFASs as described above and . Each day, embryos/larvae were inspected for death and malformations. At 96 hpf, larvae were anesthetized using tricaine methanesulfonate (MS-222; final concentration of 100 mg/L), oriented into the lateral position, and imaged using a Nikon AZ100 macroscope. Images were analyzed using DanioScope (v1.1, Noldus Information Technology, Wageningen, the Netherlands). A micrometer was imaged concurrently to convert the number of pixels to micrometers. Body length (in µm) and eye area (in µm2) were measured. These endpoints were chosen because they can be measured using non-automated methods, they are quantitative, and they are well-correlated with other measurements of developmental toxicity (Truong et al. Citation2014; Zhang et al. Citation2017). Mortality, spinal curvature, pericardial edema, and other overt malformations were also determined qualitatively to measure the percentage of normal larvae. Individual experiments were excluded from this study when ≥25% of the embryos/larvae in the vehicle control group displayed abnormal phenotypes. PFAS concentrations where body length, eye size, or percentage of normal larvae were significantly different from the vehicle control were considered to be developmentally-toxic and excluded from subsequent respiratory burst experiments.
Figure 2. In vivo and in vitro screens. (A) Zebrafish embryos were exposed to several concentrations of different PFASs from ∼6 hpf to 96 hpf. At 96 hpf, larvae were assessed for developmental toxicity or respiratory burst. (B) HL-60 cells were differentiated to a neutrophil-like phenotype (nHL-60) via DMSO treatment for 5 days. At the end of this period, cells were dosed with PFASs and plated into a 96-well plate. At 96 hr post plating, cells were assessed for cytotoxicity or respiratory burst. This strategy was also employed for a 24-hr PFAS exposure. Panel A is adapted from Phelps et al. (Citation2020). Neutrophil images in Panel B were acquired from BioRender.
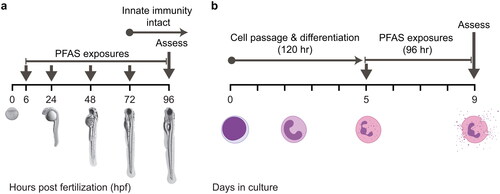
In vivo respiratory burst assays for zebrafish larvae
The respiratory burst assay used here was modified from a previously-published assay (Phelps et al. Citation2020). In brief, PFAS-exposed larvae were washed using three consecutive 80% media changes. Larvae were then plated individually into black, clear-bottom 96-well plates and stimulated with 10% Hank’s saline containing 2′,7′-dichlorofluorescin-diacetate (H2DCFDA [#D399]; ThermoFisher Scientific, Waltham, MA) and phorbol 12-myristate 13-acetate (PMA [#P8139]; Sigma, St. Louis, MO). In contrast to in a previous study (Phelps et al. Citation2020), the concentrations of H2DCFDA and PMA were increased to 1 µg/ml and 400 ng/ml, respectively, to increase the sensitivity of the assay. Wells containing larvae that received no H2DCFDA were included, as well as wells containing larvae that received no PMA; these larvae received DMSO as a vehicle control for both of these reagents. As a positive control for inhibition of the respiratory burst, larvae treated with bisindolylmaleimide I (Bis I [#203290]; EMD Millipore, Burlington, MA) at a final concentration of 10 µM for ∼10 min prior to plating were also included. Bis I was not washed out prior to plating. As a quality control check for each assay, experiments were excluded in which the fluorescent signal from the Bis I-treated larvae was not significantly reduced when compared to the vehicle control.
Fluorescence was measured as described in Phelps et al. (Citation2020). Data presented represent the maximum fluorescence from each well over the entire testing period. In addition to this, the area under the curve (AUC) was also measured to reflect all fluorescence over the entire testing period. AUC was calculated using the DescTools package (v. 0.99.44) in R using the AUC function which used a spline to calculate the AUC as close as possible to the curve (Signorell Citation2021). Data for both endpoints were normalized to the vehicle control by dividing each data point by the mean of the vehicle control and multiplying by 100 to convert it to a percentage.
Quantifying neutrophil number with Sudan black staining of zebrafish larvae
In order to count the neutrophils in larval zebrafish, Sudan Black staining - which labels neutrophil granules - was utilized (Sheehan and Storey Citation1947). For these experiments, zebrafish were exposed to PFHxA, GenX, or their respective vehicle controls as detailed above. To inhibit pigmentation, zebrafish embryos were maintained in 10% Hanks saline supplemented with 1-phenyl 2-thiourea (PTU) at a final concentration of 30 mg/L (Karlsson et al. Citation2001).
At 96 hpf, zebrafish larvae were anesthetized and transferred to a 24-well plate. Larvae were then fixed with 4% PFA/PBS for 2 hr at room temperature. Staining with Sudan Black (#380B-1KT; Sigma) was performed as previously described (Le Guyader et al. Citation2008). After staining, larvae were stored overnight in 100% glycerol at 4°C to improve transparency and reduce residual background staining. Cell counting was performed manually under a dissecting scope and representative images were taken with a Nikon AZ100 macroscope (5X magnification; Nikon, Tokyo, Japan).
HL-60 methods
In vitro exposures of human nHL-60 neutrophil-like cells to PFASs
HL-60 cells (ATCC CCL-240) were maintained at 37°C and 5% CO2 in RPMI-1640 with L-glutamine and without phenol red (Gibco [#11-835-055], Waltham, MA) supplemented with 10% heat-inactivated fetal bovine serum (Corning [#11-835-055], Corning, NY) and penicillin-streptomycin (Corning [#30-002]). HL-60 cells were authenticated using STR markers and confirmed to be free of Mycoplasma (IDEXX BioAnalytics, Columbia, MO). HL-60 cells were differentiated to a neutrophil-like phenotype (hereafter referred to as nHL-60) as previously described (Karlsson et al. Citation2011; Rincón et al. Citation2018) by passaging cells into fresh media supplemented with DMSO (1.3% final concentration) at a density of 0.3 x 106 cells/ml and incubating for 5 days.
nHL-60 cells were centrifuged at 0.4 x g for 10 min, and the supernatant was discarded. Cells were resuspended in 1.0 ml of media and counted using a Cellometer Vision (Nexcelom Biosciences, Lawrence, MA) using trypan blue exclusion to determine cell concentration and viability. Cells were then diluted to a concentration of 0.1 x 106 cells/ml in fresh media supple-mented with DMSO (final concentration of 1.3%). DMSO was required to maintain the neutrophil-like phenotype (Supplemental Figures S10 and S11). Cells were aliquoted into micro-centrifuge tubes for dosing where 1.0 ml of cells was dosed with 4.0 µl of a 250X PFAS exposure solution. Exposed cells were plated into a black-walled, clear-bottom 96-well plate at 100 µl/well. Plates were then incubated at 37°C and 5% CO2 for 24 or 96 hr prior to subsequent assays.
Cytological analyses of the differentiated state of HL-60 cells
For cytological analysis of HL-60 cells, cells were differentiated to a nHL-60 phenotype as described above or passaged without differentiation. After 5 days, cells were passaged again to create three groups: undifferentiated HL-60 cells, nHL-60 cells maintained in fresh media supplemented with 1.3% DMSO, and nHL-60 cells maintained in fresh media without DMSO. After 4 days, cells were collected via centrifugation, counted, and cell counts were normalized. Cells were then transferred to a cytospin funnel attached to a glass microscope slide with 0.5 × 106 cells in each side of the funnel. The cells were then centrifuged in a Shandon CytoSpin 4 (ThermoFisher) at 1000 rpm for 5 min. Microscope slides of cytospin preparations were batch-stained with Wright-Giemsa on a Wescor Aerospray Pro 7151 (Logan, UT) auto-slide staining machine per manufacturer instructions.
Cytology preparations of cytospin-prepared cells (undifferentiated HL-60, nHL-60, and nHL-60 with DMSO withdrawn) were evaluated by a board-certified veterinary pathologist (KEL) for morphologic features of neutrophil myeloid differentiation and mitotic activity. Based on cellular differentiation features, cells were classified as having features of promyelocytes, myelocytes, metamyelocytes, band neutrophils, or mature neutrophils. The number of each differentiated myeloid cell stage and the mitotic index were enumerated by a 300-cell differential count of cytology preparations using a BX43 microscope (Olympus, Tokyo, Japan) at 100X (oil objective) magnification. Treatments were performed in triplicate, and cell counts were averaged among triplicates.
In vitro cell viability assays for nHL-60 neutrophil-like cells
Range-finding studies for HL-60 cell viability were performed using PrestoBlue (ThermoFisher [#A13261]), according to manufacturer protocols. At the time of each assay, 11 µl PrestoBlue was added to each of the 96 wells containing 100 µl of cells (see above). To measure PrestoBlue background fluorescence, wells containing media (no cells) were included. After adding PrestoBlue to the wells, the plates were shaken on an orbital shaker at ∼100 rpm for ∼10 sec to uniformly mix the reagent. The plate was then incubated at 37°C and 5% CO2 for 30 min. After incubation, plates were analyzed on a Fluoroskan Ascent system with an excitation wavelength at 544 nm and a 590 nm emission wavelength. As a positive control, cells were induced to undergo apoptosis by treatment with 10 µM CAMP (King and Radicchi-Mastroianni Citation2002). Experiments in which the fluorescent signal from the CAMP-treated cells was not significantly reduced when compared to the vehicle control were excluded. Background fluorescence was calculated as the mean fluorescence of wells containing PrestoBlue with no cells. This value was subtracted from all other wells in the data set. Data were then normalized to the background-subtracted vehicle control by dividing each data point by the mean of the vehicle control and multiplying by 100 to convert it to a percentage.
In vitro respiratory burst assays for HL-60 neutrophil-like cells
In vitro respiratory burst assays were performed by adapting the in vivo methods described above in combination with previously reported methods for primary neutrophils (Martin et al. Citation2017; Westerman et al. Citation2018). Dihydrorhodamine-123 (DHR [#D1054]; Sigma) stocks were prepared at a final concentration of 28.87 mM in DMSO and then stored at -20°C. At 24 or 96 hr post-PFAS exposure, cells were stimulated with cell culture media containing PMA and DHR at final concentrations of 400 ng/ml and 10 µM, respectively. In contrast to the in vivo assay, the cells were not washed prior to stimulation. Wells with no cells were included to measure background fluorescence of DHR. Wells containing cells that received no DHR were included, as well as wells containing cells that received no PMA; these cells received DMSO as a vehicle control for both of these reagents. As a positive control, cells treated with Bis I at a final concentration of 10 µM for ∼10 min prior to stimulation were also included. Experiments in which the fluorescent signal from the Bis I-treated cells was not significantly reduced when compared to the vehicle control were excluded from analysis. Fluorescence was measured as described above for the in vivo assays, and maximum fluorescence and area-under-the-curve (AUC) values were calculated. Background fluorescence was calculated as the mean fluorescence of the wells with DHR and no cells. This value was subtracted from all other wells in the data set. Data for both maximum and AUC fluorescence were then normalized to the background-subtracted vehicle control by dividing each data point by the mean of the vehicle control and multiplying by 100 to convert it to a percentage.
Primary neutrophils methods
Isolation of primary human neutrophils
Human blood collection protocols were reviewed and approved by the North Carolina State University Institutional Review Board (approval #616). Whole blood (10-30 ml) was collected from consenting male and female donors aged 20-45 years using heparinized syringes. Polymorphonuclear leukocytes (PMN) were isolated from whole blood using Ficoll-Paque Plus (GE Healthcare, Chicago, IL) density gradient centrifugation. In brief, heparinized whole blood was mixed with 0.6% dextran in a 15 ml polypropylene conical tube and allowed to settle for 45-60 min at room temperature. Up to 10 ml of leukocyte rich plasma was aspirated using a bulb syringe and layered on 5 ml Ficoll in a separate 15 ml conical tube. Cells were then centrifuged for 20 min at 1800 rpm with no brake. The supernatant was discarded and remaining red blood cells within the cell pellet were depleted by 60 sec of hypotonic lysis. Isolated PMN were resuspended and washed in sterile HBSS (Life Technologies, Carlsbad, CA) without additives. Cell number and viability was quantified using trypan blue exclusion (1:1) and a manual hemocytometer count. PMN isolated by this method typically have > 98% viability and are >95% neutrophils (hereafter referred to as neutrophils). Neutrophils were maintained at 37°C and 5% CO2 in RPMI-1640 with L-glutamine and without phenol red supplemented with 10% FBS and 1X penicillin/streptomycin, as with the nHL-60 cells above. Media was also supplemented with recombinant human GM-CSF (EMD Millipore; catalog number GF304; final concentration of 100 ng/ml). Data from each human donor was treated as a separate biological replicate for ex vivo experiments.
Ex vivo exposures of primary human neutrophils to PFASs
Isolated PMN were centrifuged at 1200 rpm for 10 min, and the supernatant was discarded. Cells were then resuspended in 1.0 ml of media and diluted to a concentration of 0.1 x 106 cells/ml in fresh media. Cells were aliquoted into microcentrifuge tubes and exposed to PFASs as described above for the in vitro exposures. Cells were plated into a black 96-well plate with 100 µl per well and incubated at 37°C and 5% CO2 for 24 hr prior to subsequent assays.
Ex vivo cell viability assays for primary human neutrophils
At 24 hr post-PFAS exposure, 1.0 ml of neutrophils from each treatment group was transferred to a microcentrifuge tube and stained with propidium iodide (PI; final concentration of 2.0 µM). Cells were incubated for ∼15 min at room temperature prior to analysis by flow cytometry. As a positive control for cell death, a group of neutrophils were heat-killed by incubating at 55°C for 30 min prior to staining. Flow cytometry experiments were performed in the Flow Cytometry and Cell Sorting facility at the North Carolina State University College of Veterinary Medicine using a Becton Dickinson LSRII flow cytometer (Franklin Lakes, NJ). Cells were gated based on forward-scatter and side-scatter to gate for cellular events, excluding debris, and then gated for singlet events to exclude any large cellular debris or groups of cells that were not in single-cell suspension. A final gate was drawn in the singlet population to quantify PI+ cells. For each sample, 10,000 singlet events were recorded. Data were analyzed using FCS Express 6 (De Novo Software, Pasadena, CA).
Ex vivo respiratory burst assays for human primary neutrophils
Respiratory burst assays with primary neutrophils were performed using previously described methods (Martin et al. Citation2017; Westerman et al. Citation2018). In brief, at 24 hr post-PFAS exposure, cells were stimulated with cell culture media containing PMA and DHR at final concentrations of 50 ng/ml and 10 µM, respectively. Wells with no cells were included to measure background fluorescence of DHR. Wells containing cells that received no DHR were included, as well as wells containing cells that received no PMA; these cells received DMSO as a vehicle control for both of these reagents. As a positive control, cells treated with Bis I at a final concentration of 10 µM for ∼10 min prior to stimulation were also included. Experiments in which the fluorescent signal from the Bis I-treated cells was not significantly reduced when compared to the vehicle control were excluded from analysis. Fluorescence was measured as described above to calculate normalized maximum fluorescence and AUC.
Acellular myeloperoxidase (MPO) assays
In order to ask whether the current positive hits had direct effects on MPO activity, an acellular system was employed to test the chlorination and peroxidation processes of human MPO after PFAS exposure. A Myeloperoxidase Inhibitor Screening Assay Kit (Abcam [#ab133080]) was used in 96-well plates according to manufacturer protocols. Three independent replicates were performed with different dosing solutions. Control conditions included assessing if each PFAS interacted with the fluorescent reagents (no MPO). 4-Aminobenzhydrazide (4-ABH; 10 µM) was used as a positive control for inhibition of MPO. Plates were incubated at room temperature for 10 min in the dark, shaking at 100 RPM. After incubation, fluorescence was read using a FilterMax F5 Multi-Mode Plate Reader (Molecular Devices, San Jose, CA). Chlorination assays were read with excitation/emission of 435/535 nm, and peroxidation assays were read with excitation/emission of 535/585 nm. Data are presented as relative fluorescence units (RFU) after background fluorescence was subtracted.
Statistical analysis
All data presented represent at least three independent, combined biological replicates. Statistical analyses for developmental toxicity, cytotoxicity, and immunotoxicity assays were performed as previously described (Phelps et al. Citation2020). In brief, one-way analysis of variance (ANOVA) tests were performed using Prism software (v7.0e, GraphPad Software, La Jolla, CA). When statistical significance (p < 0.05) was observed for the ANOVA, a Dunnett’s post-hoc test was performed for pairwise comparisons between each treatment and the vehicle control. For ex vivo experiments with primary neutrophils, data were stratified by sex and treatment group for each PFAS. Two-way ANOVA was performed to determine if there were effects of sex and/or treatment. If there was an effect of sex, a Dunnett’s post-hoc test was performed within both sexes for pair-wise comparisons between treatment groups and the vehicle control. If there was not an effect of sex, a Sidak’s post-hoc test was performed for pair-wise comparisons of treatment groups and the vehicle control regardless of sex. Acellular myeloperoxidase activity assays were analyzed using a one-way ANOVA with a Tukey’s post-hoc test for pairwise comparison between all groups. When multiple concentrations of a test compound were tested and statistical significance was observed at the highest concentration, AC50 values were calculated using the drc package in R (Ritz et al. Citation2015). This was done using a two-parameter logistic regression where the maximum was fixed at 100% and the minimum was fixed at the mean of the highest concentration for each dataset.
Results
PFOS-K, PFNA, PFHxS, and Nafion byproduct 2 induced developmental toxicity in zebrafish larvae
The initial goal of this study was to expand on earlier work (Phelps et al. Citation2020) by testing the immunosuppressive properties of nine different PFASs (e.g. PFOA, PFOS-K, PFNA, PFHxA, PFHxS, PFBS, GenX, Nafion byproduct 2, and PFMOAA-Na; structures, chemical names, and CAS numbers are provided in and ) in zebrafish larvae using the exposure schematic in . To identify non-teratogenic concentrations of these compounds, range-finding studies were performed to assess developmental toxicity by measuring for three separate endpoints: body length, eye size, and percentage of normal, viable larvae in each experiment (Supplemental Figures S(1–3)). In this screen, PFOS-K, PFNA, PFHxS, and Nafion byproduct 2 were identified as developmentally toxic. Only PFOS-K and Nafion byproduct 2 induced toxicity in all three endpoints. To derive potency for each endpoint, AC50 values were calculated with a two-parameter logistic regression (Supplemental Figure S(4)). Across all three endpoints, PFOS-K was the most potent PFAS. In contrast, even though Nafion byproduct 2 was a positive hit in all three endpoints, it was the least potent PFAS for these endpoints. PFNA, which was positive only for decreasing body length, was the second-most potent, followed by PFHxS. Subsequent respiratory burst studies of these four compounds using zebrafish larvae excluded concentrations where developmental toxicity was observed.
PFHxA and GenX suppressed respiratory burst in vivo
Using non-teratogenic concentrations established in range-finding studies, the question was asked as to which of the nine PFASs in this study were able to suppress the respiratory burst in zebrafish larvae. At 96 hr post-fertilization (hpf), PFAS-exposed larvae were treated with phorbol 12-myristate 13-acetate (PMA) to induce ROS production, which was measured using the fluorescent probe H2DCFDA. The maximum fluorescence of H2DCFDA as well as the total fluorescence for the entire testing period (area under the curve, AUC) were measured. Out of the nine PFASs that were tested, two suppressed the respiratory burst with statistical significance: PFHxA and GenX. Both compounds suppressed ROS production as measured by the maximum and AUC endpoints ( and Supplemental Figure S(5)). As with the developmental toxicity positive hits, AC50 values were derived for both compounds for both endpoints measuring immunotoxicity (Supplemental Figure S(6)). While they were similar in potency, PFHxA was slightly more potent than GenX in this assay. It was also observed that 8.20 µM PFBS was a positive hit in this screen; however, given the non-monotonicity (i.e. lack of uniformly decreasing response with concentration) of this response, studies here did not pursue this compound further. To ask whether the suppression of the respiratory burst in vivo was mediated by a reduction in neutrophil numbers, Sudan Black staining, which selectively stains neutrophils, was utilized. In doing so, it was found that neither PFHxA nor GenX altered neutrophil numbers in 96 hpf zebrafish larvae (Supplemental Figure S(7)).
Figure 3. PFHxA and GenX suppressed the respiratory burst in vivo at 96 hrs. Zebrafish larvae were exposed for 96 hr to (A) PFOA, (B) PFHxA, (C) GenX, (D) PFOS-K, (E) PFHxS, (F) Nafion byproduct 2, (G) PFNA, (H) PFBS, or (I) PFMOAA-Na. Larvae were then washed and distributed into a 96 well-plate. Larvae were then treated with PMA, to induce ROS production, and H2DCFDA to measure ROS produced. Fluorescence of H2DCFDA was measured for 2.5 hr on a fluorescent plate reader set to 28.5°C. Larvae receiving no PMA and no H2DCFDA were included as controls. Larvae treated with Bis I, a protein kinase C inhibitor, were included as a positive control for inhibition of the respiratory burst. Data shown are from at least three, combined, independent biological replicates with 4-16 larvae/treatment group. Data represent the maximum amount of fluorescence over the entire testing period, with each symbol representing an individual larvae. Statistical significance (*p < 0.05) was determined by a one-way ANOVA with a Dunnett’s post-hoc test for pair-wise comparisons to the vehicle control. AUC measures can be seen in Supplemental Figure S5. Concentration responses are provided in Supplemental Figure S6.
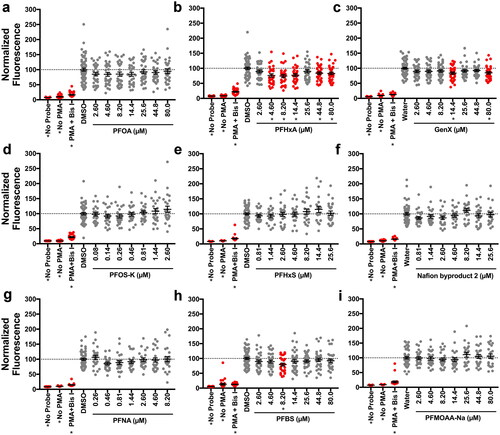
PFOA, PFOS, PFNA, PFHxS, and Nafion byproduct 2 react with PrestoBlue
To build upon the previous in vivo work here with zebrafish larvae, an in vitro model of the respiratory burst using the neutrophil-like nHL-60 human cell line was utilized. Initial studies began with range-finding cytotoxicity assays using PrestoBlue to assess viability of nHL-60 cells after exposure to individual PFASs. Cytotoxicity was not observed for any of the PFASs that were tested up to 80 µM. Unexpectedly, however, it was seen that long-chain PFASs interacted directly with PrestoBlue, causing an increase in fluorescence (Supplemental Figures S(8,9)). Because the viability of cells at these PFAS concentrations could not be confirmed, they were excluded from subsequent experiments.
Maintaining the neutrophil-like phenotype of nHL-60 cells requires DMSO
Prior to testing PFASs in the cell culture respiratory burst assay, the differentiated state of the nHL-60 cells was characterized. Although DMSO is required to differentiate HL-60 cells to a neutrophil-like nHL-60 phenotype (Supplemental Figures S(10,11)), the question was raised as to whether DMSO supplementation was necessary to maintain the neutrophil-like phenotype. Undifferentiated HL-60 cells were compared to two different groups of nHL-60 cells; one group of nHL-60s was maintained in media supplemented with DMSO while the other group had DMSO withdrawn after differentiation.
Continuous DMSO treatment of HL-60 cells induced cytomorphological features of neutrophil myeloid differentiation and maturation (Supplemental Figure S(10)). Undifferentiated HL-60 cells retained an immature phenotype, and 100% of cells had features of promyelocytes, an immature stage of myeloid differentiation. Cells were in a proliferative state and mitotic figures were present (average 7/300; 2.3%). In contrast, DMSO treatment induced neutrophilic, myeloid differentiation in HL-60 cells. All cells were more mature, displaying features of myelocyte, metamyelocyte, band neutrophil, or mature neutrophil morphology. The cells were smaller and had smaller nuclei that, in more mature cells, had nearly absent mitotic /300; 0.1%). DMSO withdrawal from neutrophil-like HL-60 cells was associated with a return to promyelocyte morphology and to mitotic activity (average 5/300; 1.7%) that was more similar to untreated cells, indicating that continued DMSO exposure was needed to main myeloid differentiation in HL-60 cells.
Functionally, these results were confirmed by performing respiratory burst assays with HL-60 cells in each state of differentiation. Parental, undifferentiated HL-60 cells did not produce ROS when treated with PMA. However, differentiated nHL-60 cells maintained in DMSO produced high levels of ROS (Supplemental Figure S(11)). nHL-60(-DMSO) cells also produced ROS in response to PMA; however, it was seen that this respiratory burst is blunted when compared to nHL-60 cells. To maintain this neutrophil-like phenotype and high dynamic range in subsequent assays, differentiated cells were maintained in the presence of DMSO.
PFOA, PFHxA, and GenX suppressed the respiratory burst in vitro after 96 hr of exposure
Using the concentrations of PFASs identified as non-cytotoxic in range-finding studies, the immunotoxicity of these compounds was next assessed in vitro. Specifically, HL-60 cells were differentiated to a nHL-60 phenotype and maintained in 1.3% DMSO for 96 hr PFAS exposures. Cells were then stimulated with PMA to induce ROS production, and DHR was used to measure ROS production. Of the nine PFASs tested, three inhibited the respiratory burst in this model: PFOA, PFHxA, and GenX (). PFOA, however, was only a positive hit for the maximum fluorescence and not the AUC for all fluorescence, unlike PFHxA and GenX ( and Supplemental Figure S(12)). AC50 values were also derived for these endpoints where PFOA was the most potent, followed by PFHxA and then GenX (Supplemental Figure S(13)). It was also observed that 0.46 µM PFNA was a positive hit in this screen. As with the in vivo assay above, due to the non-monotonicity of this response, this was not pursued further.
Figure 4. PFOA, PFHxA, and GenX suppressed the respiratory burst in vitro at 96 hr. After differentiation to nHL-60, cells were exposed to vehicle control or (A) PFOA, (B) PFHxA, (C) GenX, (D) PFOS-K, (E) PFHxS, (F) Nafion byproduct 2, (G) PFNA, (H) PFBS, or (I) PFMOAA-Na for 96 hr. Cells were then plated into a 96 well plate and stimulated with PMA to produce ROS, which was detected with DHR. Maximum fluorescence values are reported here. The entire fluorescence (AUC) values are provided in Supplemental Figure S12. Wells with no cells but with PMA and DHR, and cells receiving no PMA were included as controls. Cells treated with Bis I, a protein kinase C inhibitor, were included as a positive control for inhibition of the respiratory burst. Data shown are from three, combined, independent biological replicates, except for PFHxA, which had four biological replicates. Each biological replicate included eight technical replicates per treatment group. Individual symbols represent individual wells of a 96-well plate. Statistical significance (*p < 0.05) was determined by a one-way ANOVA with a Dunnett’s post-hoc test for pair-wise comparisons to the vehicle control.
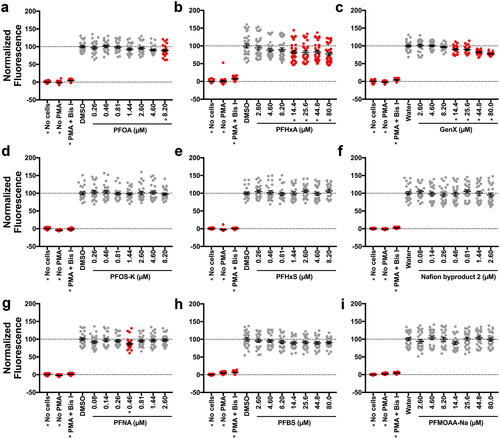
PFHxA and GenX suppressed the respiratory burst in vitro after 24 hr of exposure
For the three PFASs that suppressed the respiratory burst in nHL-60 cells after a 96 hr exposure, the question arose as to if a shorter exposure was sufficient to replicate the suppressive phenotype. Accordingly, nHL-60 cells were exposed to PFOA, PFHxA, or GenX for 24 hr, and the respiratory burst was measured. It was observed that after a 24 hr exposure, PFHxA and GenX suppressed the respiratory burst ( and Supplemental Figure S(14)). AC50 values for this endpoint contrasted from the AC50 values at 96 hr as GenX was more potent at 24 hr than at 96 hr; GenX was also more potent than PFHxA at this timepoint (Supplemental Figure S(15)).
Figure 5. PFHxA and GenX suppressed the respiratory burst in vitro at 24 hr. After differentiation to nHL-60, cells were dosed with vehicle control or (A) PFOA, (B) PFHxA, or (C) GenX and then plated into a 96-well plate. At 24 hr, cells were stimulated with PMA to produce ROS, which was detected with DHR. Maximum fluorescence values are reported here. The entire fluorescence (AUC) values are provided in Supplemental Figure S14. Wells with no cells but with PMA and DHR, and cells receiving no PMA were included as controls. Cells treated with Bis I, a protein kinase C inhibitor, were included as a positive control for inhibition of the respiratory burst. Data shown are from three, combined, independent biological replicates. Each biological replicate included eight technical replicates per treatment group. Individual symbols represent individual wells of a 96-well plate. Statistical significance (*p < 0.05) was determined by a one-way ANOVA with a Dunnett’s post-hoc test for pair-wise comparisons to the vehicle control.
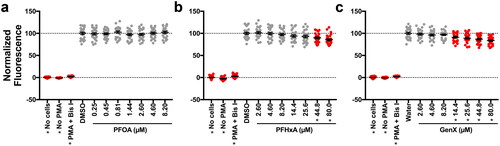
GenX suppressed the respiratory burst ex vivo
To test whether the positive hits were representative of human exposure, primary human neutrophils were isolated from six different donors and exposed ex vivo to PFHxA, GenX, or PFBS. PFBS was included to evaluate the specificity of this assay since it was not bioactive in our previous assays. Using a flow cytometry-based approach, none of the compounds were found to be cytotoxic, as measured by PI staining (Supplemental Figure S(16–17)). After 24 hr of exposure, only GenX showed significant suppression of the respiratory burst, and no effect of sex was observed with this compound ( and Supplemental Figure S(18)). In calculating AC50 values for this assay, it was noted that GenX was slightly less potent in the ex vivo assay than the in vitro assay (Supplemental Figure S(19)); this may be due to variability between donors.
Figure 6. GenX suppressed the respiratory burst ex vivo at 24 hr. After isolation from individual human donors, neutrophils were dosed with vehicle control, (A) PFHxA, (B) GenX, or (C) PFBS and distributed into a 96 well plate. At 24 hr, cells were stimulated with PMA to produce ROS, which was detected with DHR. Maximum fluorescence values are reported here. The entire fluorescence (AUC) values are provided in Supplemental Figure S18. Fluorescence of DHR was measured for 2.5 hr on a fluorescent plate reader set to 37°C. Wells with no cells but with PMA and DHR, and cells receiving no PMA were included as controls. Cells treated with Bis I, a protein kinase C inhibitor, were included as a positive control for inhibition of the respiratory burst. Data shown are from six human donors (3 male, 3 female). Each biological replicate included 3-6 technical replicates/treatment group. Individual symbols represent individual wells from a 96-well plate. Statistical significance (*p < 0.05) was determined by a two-way ANOVA with a Dunnett’s or Sidak’s post-hoc test for pair-wise comparisons to the vehicle control.
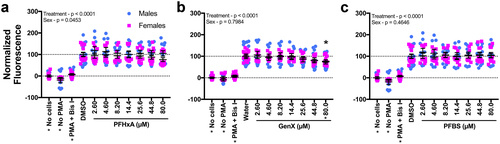
Figure 7. Summary of PFAS impact on zebrafish development, cell viability and respiratory burst in vivo, in vitro and ex vivo. AC50 values of each assay are displayed on a heatmap. Empty squares indicate no statistically significant effect at any dose. Squares with a slash indicate that the test compound was not tested in that assay. AC50 values were calculated as described in the Methods. Concentration response graphs for individual test compounds can be found in the Supplemental Figures.
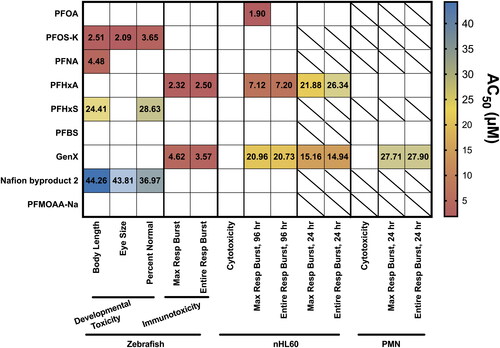
PFOA and PFHxA enhanced peroxidation by myeloperoxidase (MPO)
Finally, to investigate potential mechanisms of this observed phenotype, an acellular myeloperoxidase (MPO) activity assay was utilized to determine whether PFOA, PFHxA, or GenX had direct effects on two different activities of human MPO. Myeloperoxidase performs two functions – chlorination and peroxidation (reviewed in Siraki (Citation2021)). Chlorination is the process by which MPO creates two hypochlorous acid molecules by utilizing one hydrogen peroxide molecule as a substrate. Next, in order to return to its ground state in preparation for further chlorination reactions, peroxidation is performed. Chlorination was first measured to determine if there was an effect of the test compounds on MPO ROS production. None of the tested PFASs had a statistically-significant effect on chlorination (Supplemental Figure S(20(a))); however, treatment with GenX did trend toward a significant reduction (p = 0.1212 vs. water control). Next, MPO peroxidation activity was measured for each of the compounds. In this assay, treatment with GenX again trended toward a statistically-significant decrease (p = 0.2582 vs. water control). Conversely, treatment with PFOA or PFHxA increased MPO peroxidation activity with statistical significance (Supplemental Figure S(20(b))).
Discussion
In this study, the immunosuppressive properties of nine environmentally-relevant PFASs were investigated, as discussed below (Sun et al. Citation2016; Kotlarz et al. Citation2020; Yao et al. Citation2020). The present study began by expanding upon previous work employing zebrafish larvae to assess if specific chemical exposures suppressed the respiratory burst in larval zebrafish (Phelps et al. Citation2020). The initial range-finding studies identified four developmentally-toxic PFASs: PFOS-K, PFNA, PFHxS, and Nafion byproduct 2, all of which are long-chain PFASs. PFOS-K, PFNA, and PFHxS have been reported as developmental toxicants in larval zebrafish and other models (Zheng et al. Citation2011b; Williams et al. Citation2017; Gaballah et al. Citation2020; Mylroie et al. Citation2021). However, to date, our laboratory appears to be the first to report that Nafion byproduct 2 is toxic in larval zebrafish. To date, only three studies have investigated toxicity for this compound in experimental models including Conley et al. (Citation2022) who concluded that Nafion byproduct 2 is developmentally-toxic in rats (Lang et al. Citation2020; Wang et al. Citation2022). Interestingly, however, Gaballah et al. (Citation2020) did not identify Nafion byproduct 1 (CAS 29311-67-9) as development-ally-toxic in larval zebrafish despite its structural similarity to Nafion byproduct 2. With the present assessment of respiratory burst after exposure to these compounds in vivo, two PFASs were identified that suppress the respiratory burst in zebrafish larvae, i.e. PFHxA and GenX (; Supplemental Figure S(6)). This seems to be the first demonstration that either of these PFASs suppress the respiratory burst in any species.
The present study then sought to recapitulate these findings in a human neutrophil-like cell line. Using nHL-60 cells, range-finding cytotoxicity testing was undertaken to mirror the in vivo approaches. Initial cytotoxicity experiments revealed that none of the PFASs were cytotoxic (up to 80 μM; Supplemental Figure S(8)), an outcome consistent with previous literature, albeit those reports examined other cell types (Rosenmai et al. Citation2016; Williams et al. Citation2017; Behr et al. Citation2018; Bangma et al. Citation2020; Behr et al. Citation2020; Jabeen et al. Citation2020; Williams et al. Citation2021). To our knowledge, however, this laboratory is the first to report that specific long-chain PFASs directly interact with the PrestoBlue Cell Viability Reagent. Initially, it was hypothesized that this was a proliferative phenotype, given that others have reported that multiple PFASs can induce cell proliferation in various cell types (Pierozan et al. Citation2018; Pierozan and Karlsson Citation2018; Jabeen et al. Citation2020; Williams et al. Citation2021). However, upon incubating PFOA with PrestoBlue in the absence of cells, an increase in fluorescence was noted that was interpreted as a reaction of the two compounds (Supplemental Figure S(9)). The chemistry underlying this reaction is currently unknown; thus, investigators in the field at-large are cautioned to avoid using PrestoBlue (or other resazurin-based cytotoxicity reagents) in combination with PFAS exposure unless the cells are washed prior to the addition of PrestoBlue.
Employing in vitro respiratory burst assays with nHL-60 cells, as observed in vivo, it was determined that a 96-hr exposure to PFHxA or GenX suppressed the respiratory burst. Further, PFOA also suppressed the respiratory burst in vitro (). PFOA was identified as the most potent in this assay, followed by PFHxA and then GenX (Supplemental Figure S13). When comparing the positive hits shared between models, both PFHxA and GenX were more potent in vivo than in vitro. One possible explanation for this observation is the species differences between zebrafish and humans, as it has been reported that neutrophils vary in several aspects among different species (Fingerhut et al. Citation2020). Despite their genetic similarity (Howe et al. Citation2013), zebrafish may simply be more sensitive to immunotoxic effects of PFASs when compared to humans. This discrepancy between the models may also be driven by the larval zebrafish being an in vivo model with multiple cell types capable of producing ROS and immune-mediators (e.g. cytokines) that can influence ROS production, while nHL-60 cells are of a single cell lineage. The complexity of PFAS-induced immunotoxicity may also be complicated by absorption, distribution, metabolism, and elimination (ADME) of the compound in vivo, whereas test compounds are not subject to the same ADME considerations in vitro. Although cellular levels of PFAS were not measured, most studies on human PFAS exposure report only extra-cellular (e.g. serum) levels (Calafat et al. Citation2007; Centers for Disease Control and Prevention (CDC) Citation2019; Kotlarz et al. Citation2020; Yao et al. Citation2020). Further, measuring cellular levels of PFAS could be misleading, as PFASs may be associated with phospholipids (Fitzgerald et al. Citation2018; Nouhi et al. Citation2018) and it would be unclear if cellular levels reflect membrane-associated or intracellular PFASs.
The next question addressed here was if a shorter (24 hr) exposure to these PFASs would reproduce the observed suppression after 96-hr exposures (). Although PFOA did not suppress the respiratory burst after a 24-hr exposure, this duration of exposure to both PFHxA and GenX resulted in immunosuppression. In fact, a 24-hr GenX exposure was more potent than a 24-hr PFHxA exposure and, interestingly, than a 96-hr GenX exposure (Supplemental Figure S15). With regards to PFOA, it may indicate that longer exposures are needed to induce the suppressive phenotype. Interpreting the suppression induced by PFHxA is simpler to understand; exposure for 4 days (96 hr) resulted in an AC50 roughly three times more potent than a 1-d exposure (24 hr). Conversely, the suppressive phenotype caused by GenX in vitro is intriguing, as its potency decreased over time. This may suggest cells have compensatory mechanisms to recover from GenX exposure, or uptake kinetics of GenX change over time in this model. AC50 values for all assays are summarized in .
As primary human neutrophils can be maintained in culture for a few days (Brach et al. Citation1992), it was wondered if a 24-hr exposure to PFHxA, GenX, or PFBS would suppress the respiratory burst in primary human neutrophils (). Of these, only GenX suppressed the respiratory burst ex vivo with statistical significance. An effect of sex (p = 0.0453) was observed with PFHxA-treated cells, and this may indicate there are differences in susceptibility to PFHxA between males and females. Additional statistical power is needed to address this hypothesis. It is also worth noting that the serum concentrations of PFASs in the human donors evaluated here are unknown, which may have affected results. Regardless, PFOA, PFHxA, and GenX were identified as immunotoxic in at least one of the innate immune assays used here. Previous studies have also reported suppression of the T-cell-dependent antibody response, an adaptive immune endpoint, in mice after exposure to PFOA or GenX (Yang et al. Citation2002; DeWitt et al. Citation2008; Rushing et al. Citation2017; Shane et al. Citation2020). While there are currently no reports of PFHxA influence on T-cell-dependent antibody responses, PFHxA and one of its precursors have been linked to other immunotoxic outcomes in rodents (Rice et al. Citation2020).
While production of PFOA has been phased out in the United States, PFHxA and GenX were phased in to replace PFOA; it is intriguing that a legacy compound (PFOA) and two of its replacements (PFHxA and GenX) display the same phenotype in vitro. It is tempting to link this phenotype to the shared carboxylic acid motif in these compounds, but PFNA and PFMOAA-Na, which also possess carboxylic acid head groups, were inactive in the assays employed here, further raising questions of how this immunotoxic phenotype arises. More research is necessary to link chemical structure to the phenotypes observed in this report.
Finally, ToxPi (Reif et al. Citation2010; Marvel et al. Citation2018) was used as a way to integrate the current data using an unbiased weight-of-evidence framework to compare the present results for these nine PFASs and prioritize them for further testing. GenX and PFHxA were the most bioactive in the assays here, despite their lack of developmental toxicity, and they clustered separately from all other tested PFASs (Supplemental Figure S21). PFNA, PFHxS, and Nafion byproduct 2 cluster closely together, likely based on developmental toxicity; given the lack of publicly available toxicity data for Nafion byproduct 2, this may provide a starting point for future research to assess mechanisms of its hazard. These data also reveal that developmental toxicity was not predictive of immunotoxicity for the nine PFASs in this report. Because of this analysis, it is suggested that PFHxA and GenX be prioritized for further immunotoxicity studies.
This study chose to investigate nine PFASs known to be in surface water, drinking water, and in sera of residents downstream of a fluorochemical manufacturer. Some PFAS concentra-tions tested here were higher than those found in these matrices. For example, when Sun et al. (Citation2016) detected GenX in drinking water, the maximum concentration measured was 4,560 ppt (or ∼0.13 µM). However, in a study of humans that consumed this water (Kotlarz et al. Citation2020), serum levels of PFOS at the 95th percentile were within the testing range used here (26.8 ppb; ∼ 0.05 µM). Similarly, a cohort living near a different fluorochemical plant presented with a maximum serum concentration of PFOA to be 5,626 ppb (∼13.59 µM) (Yao et al. Citation2020), which is well within the present in vivo testing range and exceeds the concentration at which respiratory burst suppression was observed in vitro in the current study. Their sampling also detected maximum concentrations of PFMOAA at 158.2 ppb (∼ 0.88 µM), Nafion byproduct 2 at 23.9 ppb (∼ 0.05 µM), PFHxS at 14.61 ppb (∼ 0.037 µM), and PFOS at 145.1 ppb (∼ 0.29 µM); each of these measurements fall within the tested concentrations for at least one of the assays employed here. Similar observations have been made for serum collected from fish in the Cape Fear River, of which the maximum concentration detected of PFOS was 977 ppb (∼ 1.95 µM). The administered concentrations used in this study were intentionally higher than concentrations measured in human serum or reported for water samples due to the long biological half-lives reported for most PFAS. The duration of exposure for our studies was quite short and does not reflect the chronic and ongoing exposures experienced by living organisms environmentally exposed to PFAS. Thus, the concentrations used here were increased to reflect the chronic nature of environmentally relevant exposures.
The authors here caution against direct comparisons between the present work and these studies as the current experimental exposures do not account for mixtures of PFASs observed in waterways, fish, and humans in these previous studies. Follow-up studies are necessary to ascertain how PFAS mixtures may further influence the respiratory burst and other components of innate immunity. The exposures in this report also occurred on a relatively short time frame, with a maximum exposure duration of 96 hr. This short window of exposure may not fully account for the complexity of a chronic exposure to persistent bioaccumulative compounds, of which the ADME are not fully understood. Few chronic studies of PFAS-induced immunotoxicity have been performed. To date, the longest exposure where functional immunotoxicity outcomes were investigated has been a 60-day exposure to PFOS. In three separate studies, one laboratory observed altered NK cell activity, decreased lymphocyte proliferation (Dong et al. Citation2009), decreased T-cell dependent antibody response (Dong et al. Citation2009, Citation2011), increased IL-10 expression, decreased IL-2 and IFNγ expression (Dong et al. Citation2011), and increased pro-inflammatory cytokine (e.g. TNFα, IL-1β, IL-6) expression by macrophages (Dong et al. Citation2012). In a 2-year exposure of rats to PFOA, the NTP observed a decrease in spleen and thymus weights, but no functional immunotoxicity endpoints were measured (National Toxicology Program (NTP) Citation2020). Given the lack of functional innate immune endpoints in those studies, the current study may serve as a starting point from which to begin prioritization.
While the findings here are conclusive, further research is needed on several fronts. There is a need to understand the structural and mechanistic implications of the developmental toxicity induced by PFOS, PFNA, PFHxS, and Nafion byproduct 2. It must be emphasized that, even though multiple PFASs were not positive hits in the respiratory burst screens used here, this does not mean these chemicals are not immunotoxic at higher concentrations, in mixtures, or to other immune cell lineages. In a recent study, exposure to a mixture of PFASs induced ROS production in primary human neutrophils, monocytes, and lymphocytes; those authors hypothesized this may be due to the β2-adrenergic receptor (Berntsen et al. Citation2022). Many mechanisms have been proposed for PFAS-induced immunotoxicity (Neagu et al. Citation2021; Berntsen et al. Citation2022; Liang et al. Citation2022), yet it remains unclear how the data presented here fit into the proposed frameworks − the molecular mechanisms by which these PFASs suppress the respiratory burst remain unknown.
With the present in vivo model, it was hypothesized that a decrease in neutrophil number may be responsible for the observed decrease in respiratory burst. However, follow-up experiments did not support this hypothesis, as there was no significant difference in neutrophil numbers between PFHxA- or GenX-treated larvae and untreated larvae (Supplemental Figure S(7)). The study here next considered that the presently-detected positive hits may be acting directly on myeloperoxidase (MPO), an enzyme expressed almost exclusively in neutrophils to create halogenated ROS (Siraki Citation2021). In assessing chlorination activity of MPO in an acellular system, no effect of PFOA, PFHxA or GenX on chlorination was observed, indicating these compounds were unable to inhibit MPO ROS production (Supplemental Figure S(20(a))). However, it was noted that PFOA and PFHxA, but not GenX, enhanced MPO peroxidation activity (Supplemental Figure S(20(b))). Peroxidation is the process by which MPO returns to “ground state” to further react with hydrogen peroxide and form halogenated ROS (reviewed in Pick (Citation2020)). These results were counter to the original hypothesis that these PFASs act directly on MPO to inhibit its function. It is unclear how these results may contribute to the observed suppression of the respiratory burst. Given the exposure for this assay was performed acellularly on a much shorter timeframe than the other in vitro assays performed here (≤10 min vs. ≥24 hr), direct comparisons are challenging.
In addition to MPO, NADPH oxidase is also a key enzyme in the respiratory burst that produces hydrogen peroxide, and it can be activated through multiple pathways, including phosphorylation by PKC or by binding fatty acids (reviewed in Pick (Citation2020)). Because of the structural similarity between endogenous fatty acids and PFASs, it raises the question of whether PFASs may be inhibiting NADPH oxidase directly. To our knowledge, however, there is not a commercially-available method to measure NADPH oxidase activity in an acellular system. Given these confounding results, one may expect that omics approaches will serve as useful tools to generate mechanism-based hypotheses. Transcriptomics may be an excellent first step, but given that PFASs can impact metabolic (Gebreab et al. Citation2020; Hyötyläinen et al. Citation2021; Tian et al. Citation2021) and epigenetic (reviewed in Kim et al. (Citation2021)) endpoints, going beyond transcript-level expression may be necessary to understand the mechanistic underpinnings of the data in this report.
In addition, studies designed to evaluate the immunotoxicity of these compounds across several validated endpoints are needed. The respiratory burst is but one function performed by neutrophils. While its disruption may indicate dysfunction in these cells, follow-up studies to assess where PFASs may be interfering with their function, such as by inhibiting granule formation, degranulation, and/or activities of key enzymes are necessary. Other functional end-points of neutrophils should also be investigated, including chemotaxis, phagocytosis, adhesion, and formation of neutrophil extracellular traps (NETs). Interestingly, the USEPA ToxCast program screened PFOA, PFOS, and PFNA in reporter-based assays for markers of adhesion and chemotaxis; none of these PFASs showed any significant effects in these assays (reviewed in Naidenko et al. (Citation2021)). It will be interesting to determine if GenX, PFHxA and other PFASs impact these neutrophil functions.
Regardless, neutrophils represent a single, but significant, aspect of innate immunity, and each of the aforementioned endpoints are ultimately linked to susceptibility to infectious disease. While few studies have investigated this endpoint, increased susceptibility to pathogens has been observed in mice exposed to PFOS (Guruge et al. Citation2009) and frogs exposed to PFHxS (Brown et al. Citation2021). Human epidemiological studies of immunotoxicity after PFAS exposure have also reported associations between PFAS exposure and increased susceptibility to infection. PFOA, PFOS, and PFHxS have been associated with increased symptoms of infection (e.g. fevers, nasal discharge) and/or increased rates of infectious disease in children (Dalsager et al. Citation2016; Goudarzi et al. Citation2017). Notably, increased serum levels of long-chain PFASs, including PFOA, PFOS, PFNA, perfluoroheptane sulfonic acid (PFHpS), perfluorodecanoic acid (PFDA), perfluoroundecanoic acid (PFUnDA), perfluorododecanoic acid (PFDoDA), and perfluorooctane sulfonamide (PFOSA, a PFOS precursor), have been associated with increased incidence of respiratory tract infections in children (Impinen et al. Citation2018; Ait Bamai et al. Citation2020; Kvalem et al. Citation2020). This phenomenon does not appear limited to long-chain PFASs or children however, as increased plasma levels of PFBA were associated with increased severity of COVID-19 in adults (Grandjean et al. Citation2020). Nevertheless, there remains a critical need for development and implementation of high-throughput infection assays that can be used to assess whether PFASs and other environmental contaminants confer susceptibility to infectious disease.
Conclusions
This report is the first to demonstrate that PFOA, PFHxA, and GenX inhibit the respiratory burst. The study here is also the first to show that GenX suppresses innate immune function in three different models from two different species. This work complements the testing paradigm laid out by Verdon et al. (Citation2021) wherein the utility of using nHL-60 cells to prioritize chemicals prior to testing in primary neutrophils and in vivo models was described. With the present findings, the fact that the respiratory burst can be inhibited by xenobiotics has been further cemented. However, investigation of innate immune function is still lacking from the Health Effects Test Guidelines for Immunotoxicity from USEPA (Office of Prevention, Pesticides, and Toxic Substances (OPPTS) Citation1998), which rely on a tiered approach in low-throughput rodent models. Development of new approach methodologies and new testing guidelines are necessary to implement high-throughput testing strategies and reduce animal testing for immunotoxicity, while also working to incorporate endpoints for both innate and adaptive immunity to protect human and environmental health.
Author contributions
DWP and JAY contributed to study conception. DWP, MKS, SWK, JCD and JAY contributed to study design. Material preparation and data collection were performed by DWP, AIP, HEC, GF, JHD, KEL and MKS. Data analyses were performed by DWP and DMR aided in statistical analysis. The first manuscript draft was written by DWP. All authors commented on previous versions of the manuscript, and read and approved the final manuscript. SWK, JCD, MKS and JAY secured funding for this study. JAY supervised the completion of the project
Supplemental Material
Download PDF (6.1 MB)Acknowledgments
The authors thank Ashley M. Connors (NC State University) for critical review of the manuscript, volunteer donors for their time and blood for ex vivo studies, and the North Carolina State University Center for Human Health and the Environment Comparative Pathology Core for assistance with cytology experiments.
Disclosure statement
DWP was paid a one-time fee by the Center for Environmental Health (Oakland, CA, USA) for serving as a scientific advisor (May 2019–August 2022) to aid in writing a petition under the Toxic Substances Control Act to the United States Environmental Protection Agency, regarding testing of certain PFASs. JCD serves/has served as a plaintiff’s expert witness for several court cases involving PFAS manufacturers. All other authors declare no competing interests relevant to the content of this article.
Data availability
Raw data from zebrafish developmental toxicity assays, cell viability assays, respiratory burst assays, and ToxPi analysis are freely-available through the Dryad database at doi: https://doi.org/10.5061/dryad.qjq2bvqj4.
Additional information
Funding
References
- Agency for Toxic Substances and Disease Registry (ATSDR). 2021. Toxicological Profile for Perfluoroalkyls. Atlanta, GA:U.S. Department of Health and Human Services.
- Ahrens L, Barber J, Xie Z, Ebinghaus R. 2009. Longitudinal and latitudinal distribution of perfluoroalkyl compounds in the surface water of the Atlantic Ocean. Environ Sci Technol. 43(9):3122–3127.
- Ait Bamai Y, Goudarzi H, Araki A, Okada E, Kashino I, Miyashita C, Kishi R. 2020. Effect of prenatal exposure to per- and polyfluoroalkyl substances on childhood allergies and common infectious diseases in children up to age 7 years: The Hokkaido study on Environment and Children’s Health. Environ. Intl. 143:105979.
- Andrews D, Naidenko O. 2020. Population-wide exposure to per- and polyfluoroalkyl substances from drinking water in the United States. Environ Sci Technol Lett. 7(12):931–936.
- Averina M, Brox J, Huber S, Furberg A-S, Sørensen M. 2019. Serum perfluoroalkyl substances (PFAS) and risk of asthma and various allergies in adolescents. The Tromsø study fit futures in northern Norway. Environ Res. 169:114–121.
- Bangma J, Szilagyi J, Blake B, Plazas C, Kepper S, Fenton SC, Fry R. 2020. An assessment of serum-dependent impacts on intracellular accumulation and genomic response of per- and polyfluoroalkyl substances in a placental trophoblast model. Environ. Toxicol. 35(12):1395–1405.
- Behr A, Lichtenstein D, Braeuning A, Lampen A, Buhrke T. 2018. Perfluoroalkylated substances (PFAS) affect neither estrogen and androgen receptor activity nor steroidogenesis in human cells in vitro. Toxicol. Lett. 291:51–60.
- Behr A, Plinsch C, Braeuning A, Buhrke T. 2020. Activation of human nuclear receptors by perfluoroalkylated substances (PFAS). Toxicol in Vitro. 62:104700.
- Berntsen H, Bodin J, Øvrevik J, Berntsen C, Østby G, Brinchmann B, Ropstad E, Myhre O. 2022. A human-relevant mixture of persistent organic pollutants induces reactive oxygen species formation in isolated human leucocytes: Involvement of the β2-adrenergic receptor. Environ Intl. 158:106900.
- Berntsen H, Bolling A, Bjorklund C, Zimmer K, Ropstad E, Zienolddiny S, Becher R, Holme J, Dirven H, Nygaard U, et al. 2018. Decreased macrophage phagocytic function due to xenobiotic exposures in vitro, difference in sensitivity between various macrophage models. Food Chem Toxicol. 112:86–96.
- Blanter M, Gouwy M, Struyf S. 2021. Studying neutrophil function in vitro: Cell models and environmental factors. JIR. 14:141–162.
- Brach M, Brach M, deVos S, Gruss H, Herrmann F. 1992. Prolongation of survival of human polymorphonuclear neutrophils by granulocyte-macrophage colony-stimulating factor is caused by inhibition of programmed cell death. Blood. 80(11):2920–2924.
- Brieger A, Bienefeld N, Hasan R, Goerlich R, Haase H. 2011. Impact of perfluorooctanesulfon-ate and perfluorooctanoic acid on human peripheral leukocytes. Toxicol in Vitro. 25(4):960–968.
- Brown S, Flynn R, Hoverman J. 2021. Perfluoroalkyl substances increase susceptibility of northern leopard frog tadpoles to trematode infection. Environ Toxicol Chem. 40(3):689–694.
- Buck R, Franklin J, Berger U, Conder J, Cousins I, de Voogt P, Jensen A, Kannan K, Mabury S, van Leeuwen S. 2011. Perfluoroalkyl and polyfluoroalkyl substances in the environment: Terminology, classification, and origins. Integr Environ Assess Manag. 7(4):513–541.
- Buser M, Scinicariello F. 2016. Perfluoroalkyl substances and food allergies in adolescents. Environ Intl. 88:74–79.
- Calafat A, Wong L, Kuklenyik Z, Reidy J, Needham L. 2007. Polyfluoroalkyl chemicals in the U.S. population: Data from the National Health and Nutrition Examination Survey (NHANES) 2003–2004 and comparisons with NHANES 1999–2000. Environ Health Perspect. 115(11):1596–1602.
- Centers for Disease Control and Prevention (CDC). 2019. National Report on Human Exposure to Environmental Chemicals. U.S. Department of Health and Human Services. (March 2022 Update). Atlanta, GA:U.S. Department of Health & Human Services.
- Conder J, Hoke R, de Wolf W, Russell M, Buck R. 2008. Are PFCAs bioaccumulative? A critical review and comparison with regulatory criteria and persistent lipophilic compounds. Environ Sci Technol. 42(4):995–1003.
- Conley J, Lambright C, Evans N, Medlock-Kakaley E, Hill D, McCord J, Strynar M, Wehmas L, Hester S, MacMillan D, et al. 2022. Developmental toxicity of Nafion byproduct 2 (NBP2) in the Sprague-Dawley rat with comparisons to hexafluoropropylene oxide-dimer acid (HFPO-DA or GenX) and perfluorooctane sulfonate (PFOS). Environ Intl. 160:107056.
- Dahlgren C, Karlsson A, Bylund J. 2019. Intracellular neutrophil oxidants: From laboratory curiosity to clinical reality. J Immunol. 202(11):3127–3134.
- Dalsager L, Christensen N, Husby S, Kyhl H, Nielsen F, Host A, Grandjean P, Jensen T. 2016. Association between prenatal exposure to perfluorinated compounds and symptoms of infections at age 1-4years among 359 children in the Odense Child Cohort. Environ Intl. 96:58–64.
- De Guise S, Levin M. 2021. Suppression of TH2 cytokines as a potential mechanism for reduced antibody response following PFOA exposure in female B6C3F1 mice. Toxicol Lett. 351:155–162.
- DeWitt J, Blossom S, Schaider L. 2019. Exposure to per-fluoroalkyl and polyfluoroalkyl substances leads to immunotoxicity: Epidemiological and toxicological evidence. J Expo Sci Environ Epidemiol. 29(2):148–156.
- DeWitt J, Copeland C, Luebke R. 2009. Suppression of humoral immunity by perfluorooctanoic acid is independent of elevated serum corticosterone concentration in mice. Toxicol Sci. 109(1):106–112.
- DeWitt J, Copeland C, Strynar M, Luebke R. 2008. Perfluorooctanoic acid-induced immunomodulation in adult C57BL/6J or C57BL/6N female mice. Environ Health Perspect. 116(5):644–650.
- DeWitt J, Williams W, Creech N, Luebke R. 2016. Suppression of antigen-specific antibody responses in mice exposed to perfluorooctanoic acid: Role of PPARα and T- and B-cell targeting. J Immunotoxicol. 13(1):38–45.
- Dong G, Liu M, Wang D, Zheng L, Liang Z, Jin Y. 2011. Sub-chronic effect of perfluoro-octanesulfonate (PFOS) on the balance of Type 1 and Type 2 cytokine in adult C57BL6 mice. Arch Toxicol. 85(10):1235–1244.
- Dong G, Zhang Y, Zheng L, Liang Z, Jin Y, He Q. 2012. Subchronic effects of perfluoro-octanesulfonate exposure on inflammation in adult male C57BL/6 mice. Environ Toxicol. 27(5):285–296.
- Dong G, Zhang Y, Zheng L, Liu W, Jin Y, He Q. 2009. Chronic effects of perfluoro-octanesulfonate exposure on immunotoxicity in adult male C57BL/6 mice. Arch Toxicol. 83(9):805–815.
- Drutman S, Mansouri D, Mahdaviani S, Neehus A, Hum D, Bryk R, Hernandez N, Belkaya S, Rapaport F, Bigio B, et al. 2020. Fatal cytomegalovirus infection in an adult with inherited NOS2 deficiency. N Engl J Med. 382(5):437–445.
- Dupré-Crochet S, Erard M, Nüβe O. 2013. ROS production in phagocytes: Why, when, and where? J Leukoc Biol. 94(4):657–670.
- Environmental Protection Agency (EPA). 2020. PFAS Master List of PFAS Substances. EPA CompTox Chemicals Dashboard. [accessed 2022 Jan 25]. https://comptox.epa.gov/ dashboard/chemical-lists/PFASMASTER.
- Evich M, Davis M, McCord J, Acrey B, Awkerman J, Knappe D, Lindstrom A, Speth T, Tebes-Stevens C, Strynar M, et al. 2022. Per- and polyfluoroalkyl substances in the environment. Science. 375(6580):eabg9065.
- Fenton S, Ducatman A, Boobis A, DeWitt J, Lau C, Ng C, Smith J, Roberts S. 2021. Per- and polyfluoroalkyl substance toxicity and human health review: Current state of knowledge and strategies for informing future research. Environ Toxicol Chem. 40(3):606–630.
- Fingerhut L, Dolz G, de Buhr N. 2020. What is the evolutionary fingerprint in neutrophil granulocytes? IJMS. 21(12):4523.
- Fitzgerald N, Wargenau A, Sorenson C, Pedersen J, Tufenkji N, Novak P, Simcik M. 2018. Partitioning and accumulation of perfluoroalkyl substances in model lipid bilayers and bacteria. Environ Sci Technol. 52(18):10433–10440.
- Gaballah S, Swank A, Sobus JR, Howey X, Schmid J, Catron T, McCord J, Hines E, Strynar M, Tal T. 2020. Evaluation of developmental toxicity, developmental neurotoxicity, and tissue dose in zebrafish exposed to GenX and other PFAS. Environ Health Perspect. 128(4):47005.
- Gallagher R, Collins S, Trujillo J, McCredie K, Ahearn M, Tsai S, Metzgar R, Aulakh G, Ting R, Ruscetti F, et al. 1979. Characterization of the continuous, differentiating myeloid cell line (HL-60) from a patient with acute promyelocytic leukemia. Blood. 54(3):713–733.
- Gebreab K, Eeza M, Bai T, Zuberi Z, Matysik J, O’Shea K, Alia A, Berry J. 2020. Comparative toxico-metabolomics of perfluorooctanoic acid (PFOA) and next-generation perfluoroalkyl substances. Environ Pollut. 265:114928.
- Goudarzi H, Miyashita C, Okada E, Kashino I, Chen C, Ito S, Araki A, Kobayashi S, Matsuura H, Kishi R. 2017. Prenatal exposure to perfluoroalkyl acids and prevalence of infectious diseases up to 4 years of age. Environ Intl. 104:132–138.
- Grandjean P, Heilmann C, Weihe P, Nielsen F, Mogensen U, Budtz-Jorgensen E. 2017a. Serum vaccine antibody concentrations in adolescents exposed to perfluorinated compounds. Environ Health Perspect. 125(7):077018.
- Grandjean P, Heilmann C, Weihe P, Nielsen F, Mogensen U, Timmermann A, Budtz-Jorgensen E. 2017b. Estimated exposures to perfluorinated compounds in infancy predict attenuated vaccine antibody concentrations at age 5-years. J Immunotoxicol. 14(1):188–195.
- Grandjean P, Timmermann C, Kruse M, Nielsen F, Vinholt P, Boding L, Heilmann C, Mølbak K. 2020. Severity of COVID-19 at elevated exposure to perfluorinated alkylates. PLOS One. 15(12):e0244815.
- Guruge K, Hikono H, Shimada N, Murakami K, Hasegawa J, Yeung L, Yamanaka N, Yamashita N. 2009. Effect of perfluorooctane sulfonate (PFOS) on influenza A virus-induced mortality in female B6C3F1 mice. J Toxicol Sci. 34(6):687–691.
- Herb M, Schramm M. 2021. Functions of ROS in macrophages and anti-microbial immunity. Antioxidants . 10(2):313.
- Howe K, Clark M, Torroja C, Torrance J, Berthelot C, Muffato M, Collins J, Humphray S, McLaren K, Matthews L, et al. 2013. The zebrafish reference genome sequence and its relationship to the human genome. Nature. 496(7446):498–503.
- Humblet O, Diaz-Ramirez L, Balmes J, Pinney S, Hiatt R. 2014. Perfluoroalkyl chemicals and asthma among children 12–19 years of age: NHANES (1999–2008). Environ Health Perspect. 122(10):1129–1133.
- Hu X, Andrews D, Lindstrom A, Bruton T, Schaider L, Grandjean P, Lohmann R, Carignan C, Blum A, Balan S, et al. 2016. Detection of poly- and perfluoroalkyl substances (PFASs) in U.S. drinking water linked to industrial sites, military fire training areas, and wastewater treatment plants. Environ Sci Technol Lett. 3(10):344–350.
- Hyötyläinen T, Bodin J, Duberg D, Dirven H, Nygaard U, Orešič M. 2021. Lipidomic analyses reveal modulation of lipid metabolism by the PFAS perfluoroundecanoic acid (PFUnDA) in non-obese diabetic mice. Front Genet. 12:721507.
- Impinen A, Nygaard U, Lødrup Carlsen K, Mowinckel P, Carlsen K, Haug L, Granum B. 2018. Prenatal exposure to perfluoralkyl substances (PFASs) associated with respiratory tract infections but not allergy- and asthma-related health outcomes in childhood. Environ Res. 160:518–523.
- Jabeen M, Fayyaz M, Irudayaraj J. 2020. Epigenetic modifications, and alterations in cell cycle and apoptosis pathway in A549 lung carcinoma cell line upon exposure to perfluoroalkyl substances. Toxics. 8(4):112.
- Karlsson J, von Hofsten J, Olsson P. 2001. Generating transparent zebrafish: A refined method to improve detection of gene expression during embryonic development. Mar Biotechnol. 3(6):522–527.
- Karlsson T, Glogauer M, Ellen R, Loitto V, Magnusson K, Magalhães M. 2011. Aquaporin 9 phosphorylation mediates membrane localization and neutrophil polarization. J Leukoc Biol. 90(5):963–973.
- Keil D, Mehlmann T, Butterworth L, Peden-Adams M. 2008. Gestational exposure to perfluorooctane sulfonate suppresses immune function in B6C3F1 mice. Toxicol Sci. 103(1):77–85.
- Kim S, Thapar I, Brooks B. 2021. Epigenetic changes by per- and polyfluoroalkyl substances (PFAS). Environ Pollut. 279:116929.
- King M, Radicchi-Mastroianni M. 2002. Effects of caspase inhibition on camptothecin-induced apoptosis of HL-60 cells. Cytometry. 49(1):28–35.
- Klebanoff S. 1970. Myeloperoxidase: Contribution to the microbicidal activity of intact leukocytes. Science. 169(3950):1095–1097.
- Kotlarz N, McCord J, Collier D, Lea CS, Strynar M, Lindstrom A, Wilkie A, Islam J, Matney K, Tarte P, et al. 2020. Measurement of novel, drinking water-associated PFAS in blood from adults and children in Wilmington, North Carolina. Environ Health Perspect. 128(7):77005.
- Kvalem H, Nygaard U, Lødrup Carlsen K, Carlsen K, Haug L, Granum B. 2020. Perfluoroalkyl substances, airways infections, allergy, and asthma related health outcomes – implications of gender, exposure period and study design. Environ Intl. 134:105259.
- Lang J, Strynar M, Lindstrom A, Farthing A, Huang H, Schmid J, Hill D, Chernoff N. 2020. Toxicity of Balb/c mice exposed to recently identified 1,1,2,2-tetrafluoro-2-[1,1,1,2,3,3-hexafluoro-3-(1,1,2,2-tetrafluoroethoxy)propan-2-yl]oxyethane-1-sulfonic acid (PFESA-BP2). Toxicology. 441:152529.
- Le Guyader D, Redd M, Colucci-Guyon E, Murayama E, Kissa K, Briolat V, Mordelet E, Zapata A, Shinomiya H, Herbomel P. 2008. Origins and unconventional behavior of neutrophils in developing zebrafish. Blood. 111(1):132–141.
- Liang L, Pan Y, Bin L, Liu Y, Huang W, Li R, Lai K. 2022. Immunotoxicity mechanisms of perfluorinated compounds PFOA and PFOS. Chemosphere. 291(Pt 2):132892.
- Liberatore H, Jackson S, Strynar M, McCord JP. 2020. Solvent suitability for HFPO-DA (“GenX” parent acid) in toxicological studies. Environ Sci Technol Lett. 7(7):477–481.
- Manara S, Chin J, Schneider D. 1991. Role of degranulation in activation of the respiratory burst in human neutrophils. J Leukoc Biol. 49(5):489–498.
- Martin E, Till R, Sheats M, Jones S. 2017. Misoprostol Inhibits equine neutrophil adhesion, migration, and respiratory burst in an in vitro model of inflammation. Front Vet Sci. 4:159.
- Marvel S, To K, Grimm F, Wright F, Rusyn I, Reif D. 2018. ToxPi Graphical User Interface 2.0: Dynamic exploration, visualization, and sharing of integrated data models. BMC Bioinf. 19(1):80.
- Midgett K, Peden-Adams M, Gilkeson G, Kamen D. 2015. In vitro evaluation of the effects of perfluorooctanesulfonic acid (PFOS) and perfluorooctanoic acid (PFOA) on IL-2 production in human T-cells. J Appl Toxicol. 35(5):459–465.
- Mylroie J, Wilbanks M, Kimble A, To K, Cox C, McLeod S, Gust K, Moore D, Perkins E, Garcia-Reyero N. 2021. Perfluorooctanesulfonic acid-induced toxicity on zebrafish embryos in the presence or absence of the chorion. Environ Toxicol Chem. 40(3):780–791.
- Naidenko O, Andrews DQ, Temkin AM, Stoiber T, Uche UI, Evans S, Perrone-Gray S. 2021. Investigating molecular mechanisms of immunotoxicity and the utility of ToxCast for immunotoxicity screening of chemicals added to food. IJERPH. 18(7):3332.
- National Toxicology Program (NTP). 2016. Monograph on Immunotoxicity Associated with Exposure to perfluorooctanoic acid (PFOA) and perfluorooctane sulfonate (PFOS). Research Triangle Park, NC: U.S. Department of Health and Human Services.
- National Toxicology Program (NTP). 2020. NTP technical report on the toxicology and carcinogenesis studies of perfluorooctanoic acid (CASRN 335-67-1) administered in feed to Sprague Dawley (Hsd:Sprague Dawley® SD®) Rats. Research Triangle Park, NC: U.S. Department of Health and Human Services.
- Neagu M, Constantin C, Bardi G, Duraes L. 2021. Adverse outcome pathway in immunotoxicity of perfluoroalkyls. Curr Opin Toxicol. 25:23–29.
- Neehus A, Moriya K, Nieto-Patlán A, Le Voyer T, Lévy R, Özen A, Karakoc-Aydiner E, Baris S, Yildiran A, Altundag E, et al. 2021. Impaired respiratory burst contributes to infections in PKCδ-deficient patients. J Exp Med. 218:e20210501.
- Neehus A, Tuano K, Le Voyer T, Nandiwada S, Murthy K, Puel A, Casanova JL, Chinen J, Bustamante J. 2022. Chronic granulomatous disease-like presentation of a child with autosomal recessive PKCδ deficiency. J Clin Immunol. 42(6):1244–1253.
- Ng C, Hungerbühler K. 2014. Bioaccumulation of perfluorinated alkyl acids: Observations and models. Environ Sci Technol. 48(9):4637–4648.
- Nouhi S, Ahrens L, Campos Pereira H, Hughes A, Campana M, Gutfreund P, Palsson G, Vorobiev A, Hellsing M. 2018. Interactions of perfluoroalkyl substances with a phospholipid bilayer studied by neutron reflectometry. J Colloid Interface Sci. 511:474–481.
- Office of Prevention, Pesticides, and Toxic Substances (OPPTS). 1998. Health Effects Test Guidelines OPPTS 870.7800 Immunotoxicity. Research Triangle Park, NC: U.S. Environmental Protection Agency.
- Organization for Economic Cooperation and Development (OECD). 2018. Environment Directorate Joint Meeting of the Chemicals Committee and the Working Party on Chemicals, Pesticides and Biotechnology. Toward a new comprehensive global database of per- and polyfluoroalkyl substances (PFASs): Summary report on updating the OECD 2007 list of per- and polyfluoroalkyl substances (PFASs). Series on Risk Management #39. Paris, France: Inter-Organization Program for Sound Management of Chemicals (IOMC).
- Peden-Adams M, EuDaly J, Dabra S, EuDaly A, Heesemann L, Smythe J, Keil D. 2007. Suppression of humoral immunity following exposure to the perfluorinated insecticide sulfluramid. J Toxicol Environ Health. 70(13):1130–1141.
- Peden-Adams M, Keller J, Eudaly J, Berger J, Gilkeson G, Keil D. 2008. Suppression of humoral immunity in mice following exposure to perfluorooctane sulfonate. Toxicol Sci. 104(1):144–154.
- Phelps D, Fletcher A, Rodriguez-Nunez I, Balik-Meisner M, Tokarz D, Reif D, Germolec D, Yoder J. 2020. In vivo assessment of respiratory burst inhibition by xenobiotic exposure using larval zebrafish. J Immunotoxicol. 17(1):94–104.
- Pick E. 2020. Cell-free NADPH oxidase activation assays: A triumph of reductionism. Meth Mol Biol. 2087:325–411.
- Pierozan P, Jerneren F, Karlsson O. 2018. Perfluorooctanoic acid (PFOA) exposure promotes proliferation, migration, and invasion potential in human breast epithelial cells. Arch Toxicol. 92(5):1729–1739.
- Pierozan P, Karlsson O. 2018. PFOS induces proliferation, cell-cycle progression, and malignant phenotype in human breast epithelial cells. Arch Toxicol. 92(2):705–716.
- Reif D, Martin M, Tan S, Houck K, Judson R, Richard A, Knudsen T, Dix D, Kavlock R. 2010. Endocrine profiling and prioritization of environmental chemicals using ToxCast data. Environ Health Perspect. 118(12):1714–1720.
- Rice P, Aungst J, Cooper J, Bandele O, Kabadi SV. 2020. Comparative analysis of the toxico-logical databases for 6:2 fluorotelomer alcohol (6:2 FTOH) and perfluorohexanoic acid (PFHxA). Food Chem Toxicol. 138:111210.
- Rincón E, Rocha-Gregg B, Collins S. 2018. A map of gene expression in neutrophil-like cell lines. BMC Genomics. 19(1):573.
- Ritz C, Baty F, Streibig J, Gerhard D. 2015. Dose-response analysis rsing R. PLOS ONE. 10(12):e0146021.
- Rockwell C, Turley A, Cheng X, Fields P, Klaassen C. 2013. Acute immunotoxic effects of perfluorononanoic acid (PFNA) in C57BL/6 mice. Clin Exp Pharmacol Physiol. Suppl 4:S4- 002.
- Rosenmai A, Taxvig C, Svingen T, Trier X, van Vugt-Lussenburg B, Pedersen M, Lesné L, Jégou B, Vinggaard A. 2016. Fluorinated alkyl substances and technical mixtures used in food paper-packaging exhibit endocrine-related activity in vitro. Andrology. 4(4):662–672.
- Rushing B, Hu Q, Franklin J, McMahen R, Dagnino S, Higgins C, Strynar M, DeWitt J. 2017. Evaluation of the immunomodulatory effects of 2,3,3,3-tetrafluoro-2-(heptafluoropropoxy)-propanoate in C57BL/6 mice. Toxicol Sci. 156:179–189.
- Shane H, Baur R, Lukomska E, Weatherly L, Anderson S. 2020. Immunotoxicity and allergenic potential induced by topical application of perfluorooctanoic acid (PFOA) in a murine model. Food Chem Toxicol. 136:111114.
- Sheehan H, Storey G. 1947. An improved method of staining leucocyte granules with Sudan black B. J Pathol Bacteriol. 59(1-2):336.
- Signorell A. 2021. DescTools: Tools for descriptive statistics. R package. https://andrisignorell. github.io/DescTools/.
- Siraki A. 2021. The many roles of myeloperoxidase: From inflammation and immunity to bio-markers, drug metabolism, and drug discovery. Redox Biol. 46:102109.
- Steenland K, Zhao L, Winquist A, Parks C. 2013. Ulcerative colitis and perfluorooctanoic acid (PFOA) in a highly exposed population of community residents and workers in the mid-Ohio valley. Environ Health Perspect. 121(8):900–905.
- Sun M, Arevalo E, Strynar M, Lindstrom A, Richardson M, Kearns B, Pickett A, Smith C, Knappe D. 2016. Legacy and emerging perfluoroalkyl substances are important drinking water contaminants in the Cape Fear River watershed of North Carolina. Environ Sci Technol Lett. 3(12):415–419.
- Tian J, Hong Y, Li Z, Yang Z, Lei B, Liu J, Cai Z. 2021. Immunometabolism-modulation and immunotoxicity evaluation of perfluorooctanoic acid in macrophages. Ecotoxicol Environ Saf. 215:112128.
- Truong L, Reif D, St Mary L, Geier M, Truong H, Tanguay R. 2014. Multi-dimensional in vivo hazard assessment using zebrafish. Toxicol Sci. 137(1):212–233.
- Verdon R, Gillies S, Brown D, Henry T, Tran L, Tyler C, Rossi A, Stone V, Johnston H. 2021. Neutrophil activation by nanomaterials in vitro: Comparing strengths and limitations of primary human cells with those of an immortalized (HL-60) cell line. Nanotoxicology. 15(1):1–20.
- Wang Z, DeWitt J, Higgins C, Cousins I. 2017. A never-ending story of per- and polyfluoroalkyl substances (PFASs)? Environ Sci Technol. 51(5):2508–2518.
- Wang Z, Yao J, Guo H, Sheng N, Guo Y, Dai J. 2022. Comparative hepatotoxicity of a novel perfluoroalkyl ether sulfonic acid, Nafion byproduct 2 (H-PFMO2OSA), and legacy perfluorooctane sulfonate (PFOS) in adult male mice. Environ Sci Technol. 56(14):10183–10192.
- Westerfield M. 2007. The Zebrafish Book. A Guide for the Laboratory Use of Zebrafish (Danio rerio). 5th Edition. Eugene, OR: University of Oregon Press.
- Westerman T, Bogomolnaya L, Andrews-Polymenis H, Sheats M, Elfenbein J. 2018. The Salmonella type-3 secretion system-1 and flagellar motility influence the neutrophil respiratory burst. PLOS One. 13(9):e0203698.
- Williams A, Grulke C, Edwards J, McEachran A, Mansouri K, Baker N, Patlewicz G, Shah I, Wambaugh J, Judson R, et al. 2017. The CompTox chemistry dashboard: A community data resource for environmental chemistry. J Cheminform. 9(1):61.
- Williams A, Williams A, Lambert J, Thayer K, Dorne J. 2021. Sourcing data on chemical properties and hazard data from the US-EPA CompTox chemicals dashboard: A practical guide for human risk assessment. Environ Intl. 154:106566.
- Yang Q, Abedi-Valugerdi M, Xie Y, Zhao X, Möller G, Nelson B, DePierre J. 2002. Potent suppression of the adaptive immune response in mice upon dietary exposure to the potent peroxisome proliferator, perfluorooctanoic acid. Intl. Immunopharmacol. 2(2–3):389–397.
- Yao J, Pan Y, Sheng N, Su Z, Guo Y, Wang J, Dai J. 2020. Novel perfluoroalkyl ether carboxylic acids (PFECAs) and sulfonic acids (PFESAs): Occurrence and association with serum biochemical parameters in residents living near a fluorochemical plant in China. Environ Sci Technol. 54(21):13389–13398.
- Yu L, Li W, Lv G, Sun G, Yang L, Chen J, Zhou L, Ding Y, Zhang Z, Tang X, et al. 2023. De novo somatic mosaicism of CYBB caused by intronic LINE-1 element insertion resulting in chronic granulomatous disease. J Clin Immunol. 43(1):88–100.
- Zhang C, McElroy A, Liberatore H, Alexander N, Knappe D. 2022. Nov 4 Stability of per- and polyfluoroalkyl substances in solvents relevant to environmental and toxicological analysis. Environ Sci Technol. 56(10):6103–6112.
- Zhang G, Roell K, Truong L, Tanguay R, Reif D. 2017. A data-driven weighting scheme for multivariate phenotypic endpoints recapitulates zebrafish developmental cascades. Toxicol Appl Pharmacol. 314:109–117.
- Zheng L, Dong G, Jin Y, He Q. 2009. Immunotoxic changes associated with a 7-day oral exposure to perfluorooctanesulfonate (PFOS) in adult male C57BL/6 mice. Arch Toxicol. 83(7):679–689.
- Zheng L, Dong G, Zhang Y, Liang Z, Jin Y, He Q. 2011a. Type 1 and Type 2 cytokine imbalance in adult male C57BL/6 mice following a 7-day oral exposure to perfluorooctanesulfonate (PFOS). J Immunotoxicol. 8(1):30–38.
- Zheng X, Liu H, Shi W, Wei S, Giesy J, Yu H. 2011b. Effects of perfluorinated compounds on development of zebrafish embryos. Environ Sci Pollut Res. 19(7):2498–2505.
- Zhong Y, Shen L, Ye X, Zhou D, He Y, Zhang H. 2020. Mechanism of immunosuppression in zebrafish (Danio rerio) spleen induced by environmentally relevant concentrations of perfluorooctanoic acid. Chemosphere. 249:126200.